DOI:
10.1039/C9RA04602J
(Paper)
RSC Adv., 2019,
9, 26321-26326
Transition metal atom (Ti, V, Mn, Fe, and Co) anchored silicene for hydrogen evolution reaction
Received
19th June 2019
, Accepted 14th August 2019
First published on 22nd August 2019
Abstract
Non-noble element catalysis for hydrogen evolution reaction (HER) is a promising pathway for mass hydrogen production through electrochemical water splitting. In this work, the catalytic performance of metal (alkali, alkali-earth, and transition metal) atoms anchored to silicene was investigated by density functional theory. Results showed that all the studied metal atoms are energetically favorably absorbed on the silicene with large binding energies. The pristine silicene is catalytically inert for HER, while the metal (Fe, V, Mn, Ti, Co, Ni, Be, and Cr) atom anchored silicene is catalytically active for HER with the calculated Gibbs free energies in the range between −0.09 and 0.18 eV, which is very close to the optimum value of 0.0 eV. These results suggested that the catalytic behavior of silicene can be effectively improved by metal adsorption. Such metal (Fe, V, Mn, Ti, Co, Ni, Be, and Cr) atom anchored silicenes can be used as potential catalysts for HER.
1. Introduction
The demand for energy is rapidly increasing with the growth of the world economy. Fossil fuels have been the main energy supply in the past few decades. In recent years, the energy crisis is becoming complicated due to the limited supply of fossil fuels and environmental concerns associated with carbon dioxide emission from the burning of fossil fuels.1 To meet the daunting energy demands in an environmentally sustainable way, the need of large-scale non-fossil fuel clean energy sources is urgent.2 Hydrogen, the simplest and relatively abundant element, has a high energy density by mass and is sustainable and eco-friendly as an alternative fuel.3,4 Among the various methods of hydrogen production, electrochemical water splitting is considered sustainable and useful for mass hydrogen production. Although the platinum-group metals work efficiently in the hydrogen production from water via hydrogen evolution reaction (HER), their scarcity and high cost hinder their widespread application. Developing efficient noble metal free electrocatalysts comparable to the platinum-group metals is urgent and remains a big challenge.5
Various non-noble metal electrocatalysts, including metal alloys, transition metal compounds, and carbonaceous nanomaterials,6 have been investigated for HER. Whereas all of them show some catalytic activity for HER, none of them are comparable with the platinum-group metal catalysts. Many kinds of schemes were investigated to further improve their catalytic performance. The electrochemical performance of metal alloys can be improved by increasing the density of the catalytically active sites for HER. For example, the HER performance of AuPd7 was enhanced though introducing porosity.8 Transition metal chalcogenides, such as MoS2,9,10 CoP,11 CoSe2,12 and WS2,13 show good catalytic performance, hence attracting much attention to be used as electrocatalysts for HER. However the catalytically active sites located at the edges site in either metallic or semiconducting transition metal sulfides,14 and in the trithio- or triseleno-phosphate compounds as well,15 thus leaving large surface site unavailable for HER. Doping has been used to increase the number of catalytically active sites, such as Se-doped MoS2,16 C-doped MoS2,17 Heterostructures, such as MoSe2/CoSe2,18 CoP/WS2,19 and MoSe2/NiSe,20 have also been evaluated as electrocatalysts for HER. Besides, many other approaches, such as decreasing the size,8 introducing defects,21,22 and controlling the phase transition23–25 in MoS2, have been proposed to increase the catalytically active sites.
Silicene, a single silicon atomic layer, has a graphene-like structure. The successful synthesis of silicene on metal surface using epitaxial growth method26,27 has given rise to a lot of attention due to its excellent electrochemical properties. The pristine silicene has a zero band gap,28 which can be tuned by in-plane strain and external vertical electrical field.29,30 The field-effect transistors based on the silicene has been fabricated by Li's group.31 The adsorption of some adatoms on silicene has been studied32–36 and the buckled silicene shows strong adsorption of the adatoms. The electronic properties of silicene can be modified by the adsorption of adatoms.37 The recent development of single-atom catalysis offers electrocatalytic mechanism with great potential,38 among which the isolated metal atom being anchored to two-dimensional materials has been well developed as catalyst for HER. The Pt atom confined into graphene showed higher catalytic activity than the conventional Pt nanoparticles.39 Pt and Co atoms adsorbed on g-C3N4 show good catalytic performance.40 The Pt,41 Pd,42 Zn,43 Ni,44 W,45 V, Fe, Co, Ni, Cu,46 and Se47 atoms adsorbed on MoS2 also show high catalytic performance for HER. This inspired us to study the strong binding of metal adatoms to silicene as catalyst for HER. Compared to other support materials, the silicene is also an atomic thick material with buckled layer structure, which results in large surface area.48 Although the silicene has a similar zero band-gap characteristic with graphene. It is expected silicene with high reactivity with adsorption of adatom due to the sp3-like buckled structure. Previous calculation has shown that many adatoms (such as Li, Na, K, Ca, Co, Ni, Pd and Pt) with stronger binding to silicene than to graphene.36 Importantly, Si is the second most abundant element on Earth, thus silicene is expected to be good support materials for single-atom catalysis.
In this paper, the catalytic activity of alkali, alkali-earth, transition metal atoms adsorbed on silicene for HER was investigated by using density functional theory (DFT). The calculation results showed that the pristine silicene is catalytically inert for HER due to the large positive value (0.57 eV) of Gibbs adsorption energy for hydrogen. However, transition metal atoms adsorbed on silicene, such as Ti and Co, shows an excellent catalytic activity for HER.
2. Computational details
The SIESTA code49 developed based on DFT was adopted for all the simulations. The electron-ion core interaction were described by using norm-conserving pseudopotentials, and the valence electron wave functions were expanded using a double-ζ basis set plus polarization function50 with an energy cut-off of 180 Ry. The generalized gradient approximation (GGA) of Perdew–Burke–Ernzerhof (GGA-PBE) functional was adopted to describe the electron exchange–correlation interaction.50 There are two atoms in the unit cell of silicene. The atomic positions and lattice constants were fully relaxed with geometry optimization by using conjugate gradient method until the force on each atom is less than 0.01 eV Å−1. The Brillouin zone (BZ) was sampled with a 20 × 20 × 1 k-point mesh51 for the unit cell of silicene. The plane of silicene is parallel to the x–y plane; a vacuum layer with a thickness of 30 Å along the z-direction was used to avoid periodic image interactions.
The electrocatalytic activity of alkali, alkali-earth, and transition metal adatoms adsorbed on silicene was evaluated by calculating the Gibbs free energy (ΔGH) of hydrogen adsorption. ΔGH was calculated by the following equation:
|
ΔGH = ΔEH + ΔEZPE − TΔSH
| (1) |
where Δ
EZPE is the difference of zero-point energy between the adsorption state and gas phase, the value of Δ
EZPE is calculated to be ∼0.16 eV.
52,53 Δ
SH is the difference of entropy between the adsorption state of hydrogen and gas phase. At the standard temperature of 300 K and standard atmospheric pressure of 1 bar, the value of −
TΔ
SH is calculated to be 0.20 eV.
23,52 To sum up, the value of Δ
EZPE −
TΔ
SH is equal to 0.365 eV. Δ
EH was calculated by the
eqn (2):
|
 | (2) |
where
E*H and
E* are the total energies adatom adsorbed silicene with and without hydrogen adsorption, respectively.
EH2 is the total energy of hydrogen molecule in the gas phase.
54
The basis set superposition error (BSSE) induced by the artificial shortening of distances and strengthening of the interactions was corrected by applying the counterpoise corrections using ‘ghost’ atoms.55,56
3. Result and discussion
Silicene has a hexagonal atomic arrangement, with the top and side views of silicene is shown in Fig. 1a and b, respectively. The dashed rhombus denotes the unit cell of silicene. The silicene has a wrinkled surface, which consists of I- and II-sub-structure atoms.57 Using first principle calculation, the lattice constant of silicene is calculated to be 3.86 Å with the bond length of 2.28 Å for Si–Si bond. The buckling distance (δ) is 0.51 Å. The angle of ∠Si–Si–Si is 115°, which is in good agreement with the previous work.58,59 The angle between Si–Si bond and the z axis is 122°. The band structure of silicene is shown in Fig. 1c. Silicene is a zero band-gap material with both the valence band maximum (VBM) and conduction band minimum (CBM) located at k point, both crossing the linear dispersion with the Fermi level.60
 |
| Fig. 1 (a) Top and (b) side view of silicene, and the top and bottom atoms are labeled as I- and II-sublattice. The rhombus denotes the primitive unit cell of silicene. (c) Band structure and (d) phonon dispersion of silicene. | |
The phonon dispersion of silicene was calculated by using the frozen phonon method50 with a 6 × 6 supercell. Phonon dispersion of silicene along the high-symmetry direction is shown in Fig. 1d. There are two atoms in the unit cell, one is for I-sublattice and the other one for the II-sublattice, so there are six branches of phonon spectrum consisting of longitudinal acoustic (LA), transverse acoustic (TA), flexural out-of-plane acoustic (ZA), longitudinal optical (LO), transverse optical (TO), and flexural out-of-plane optical (ZO), which agrees with the previous results.61,62 The ZA branch near the Γ point shows a quadratic trend, which is a typical characteristic of two-dimensional materials and can be explained by the macroscopic elastic theory.61,62 There is no imaginary frequency for the silicene, which indicates that the silicene is dynamically stable.
The strong binding of adatoms to a two-dimensional is beneficial to maintaining a long cycle life for catalytic performance. The binding between the metal adatom and silicene was evaluated by using the eqn (3):
|
Eads = Eadatom + Esilicene − Eadatom@silicene
| (3) |
where
Eadatom@silicene and
Esilicene are the total energies of silicene with and without metal adatom adsorption, respectively.
Eadatom is the energy of an isolated metal adatom. The lager the adsorption energy is, the stronger binding between adatom and silicene is. There are four possible symmetry adsorption sites for adatoms on the surface of silicene (as shown in
Fig. 1a and b), which are the top of upper silicon atom (T), the valley site,
i.e. the top of bottom silicon atom (V), the bridge site of Si–Si bond (B), and the center of the hexagon (H). The calculated binding energies are listed in
Table 1.
Table 1 Calculated binding energy (eV) of alkali, alkali-earth, and transition metal adatoms anchored to silicene. The catalytic active sites for HER with the Gibbs free energies for hydrogen adsorption. And adsorption energy (eV) of hydrogen molecules on pristine, alkali, alkali-earth, and transition metal atoms anchored silicene
|
Binding energy (eV) |
HER |
Adsorption energy(eV) |
T |
V |
H |
B |
Optimum site |
Adsorption site |
ΔGH (eV) |
Adsorption site |
Eads(H2) (eV) |
Li |
2.16 |
2.34 |
2.65 |
2.34 |
H |
T |
0.19 |
T |
0.01 |
Na |
1.85 |
1.99 |
2.22 |
1.99 |
H |
T |
0.20 |
T |
0.00 |
K |
2.25 |
2.06 |
2.25 |
2.06 |
H |
T |
0.27 |
T |
−0.06 |
Be |
0.61 |
1.54 |
1.24 |
1.53 |
V |
Tad |
0.10 |
Tad |
−0.02 |
Mg |
0.63 |
1.16 |
1.04 |
1.16 |
V |
Tad |
0.34 |
Tad |
0.00 |
Ca |
1.37 |
1.37 |
1.29 |
1.36 |
V |
T |
0.39 |
Tad |
0.16 |
Sc |
4.54 |
4.12 |
4.31 |
4.54 |
B |
T |
−0.16 |
T |
−0.31 |
Ti |
5.20 |
5.44 |
5.70 |
5.43 |
H |
Tad |
−0.01 |
H |
−0.02 |
V |
6.32 |
6.16 |
6.32 |
6.16 |
H |
Tad |
−0.07 |
T |
0.00 |
Cr |
6.37 |
6.54 |
6.61 |
6.54 |
H |
T |
0.18 |
T |
0.00 |
Mn |
6.45 |
6.56 |
6.87 |
6.56 |
H |
T |
−0.06 |
T |
0.00 |
Fe |
6.47 |
6.58 |
7.17 |
6.58 |
H |
T |
−0.09 |
T |
−0.01 |
Co |
7.61 |
7.79 |
8.47 |
7.79 |
H |
Tad |
0.02 |
T |
−0.01 |
Ni |
6.97 |
7.21 |
7.97 |
7.21 |
H |
T |
0.13 |
T |
0.00 |
Cu |
4.33 |
4.51 |
5.02 |
4.51 |
H |
T |
0.57 |
T |
0.00 |
Pristine |
— |
— |
— |
— |
— |
T |
0.57 |
T |
0.00 |
For the alkali adatoms (Li, Na, and K) adsorption on the silicene, all of them are strongly bound to the H site. The binding energies are 2.65, 2.22, and 2.25 eV for Li, Na, and K, respectively. The geometry structure of alkali atoms absorbed on silicene is shown in Fig. 2a. Similar to the alkali atom absorbed on graphene,63 as the alkali adatoms are absorbed on the silicene, the valence electrons of alkali atoms transfer to the silicene, resulting in strong binding between them. The alkali-earth atoms show different energy preferable adsorption sites compared with alkali atoms, favoring to be absorbed at the V site, as shown in Fig. 2b. The binding energies are 1.54, 1.16, and 1.37 eV for Be, Mg, and Ca adatoms, respectively. From Table 1, it can be seen that except Sc, all the studied transition metal adatoms are strongly bounded to the H site of silicene. The atomic configurations are shown in Fig. 2c. The bridge site is energetically favorable one for Sc adsorption. The binding energies are in the range between 4.54 and 8.47 eV, much larger than the alkali and alkali-earth atoms. It is interesting to find that the Fe atom is absorbed at the center of the hexagon in the silicene plane. The transition metal adatoms (Ti, V, Cr, Mn, Fe, Co, Ni, and Cu) are strongly bounded to the H site of silicene and form covalent bonds with the nearest Si atoms. Among them, the Mn, Fe, Co, Ni and Cu atoms have smallest atomic radius. The bond lengths of Mn–Si, Co–Si, Ni–Si and Cu–Si bonds are 2.35, 2.60, 2.61, and 2.70 Å, respectively. Whereas the bond-length of six Fe–Si bond is about 2.32 Å, which is close to Si–Si bond length (2.28 Å), results in the Fe atoms absorbed at the center of the hexagon in the silicene plane. These results are in agreement with the previous work.36 The strong binding energies are beneficial for them as catalyst for HER.
 |
| Fig. 2 Top and side view for bonding geometry structures for (a) alkali, (b) alkali-earth, (c) Sc, (d) transition metal (except Fe atom) and (e) Fe atoms on silicene. | |
After identifying the favorable adsorption sites for adatoms on the silicene, the catalytic performance for HER of the alkali, alkali-earth, and transition metal atoms anchored on the silicene was investigated by the calculation of the Gibbs free energy for hydrogen adsorption. For the pristine silicene, the H is preferable to be absorbed at T site, given the large ΔGH of 0.57 eV, which is much smaller that H absorbed on graphene (1.80 eV).64,65 This result is also caused by the high reactivity of the sp3-like buckled structure compared to the sp2-hybridization in graphene.37 The large positive value means that the hydrogen is difficult to bind to the silicene, indicating the pristine silicene is catalytically inert for HER. The silicene with metal adatom anchored (adatom@silicene) can be classified into three types according to their adsorption sites, i.e. the H type (Li, Na, K, Ti, V, Cr, Mn, Co, Ni, Cu, and Fe), V type (Be, Mg, and Ca) and the B type (Sc). The possible adsorption sites for hydrogen on the three types of adatom@silicene systems are shown in Fig. 3a–c. Four possible adsorption sites are considered, i.e. the top of the adatom (Tad), the top of upper silicon atom (T), the valley site (V), and the center of the hexagon (H). The energetically favorable adsorption sites for hydrogen on adatom@silicene system along with the calculated ΔGH are listed in Table 1. A large positive or negative value of ΔGH indicates that hydrogen adsorption on the catalyst is too strong or too weak, both of them are not beneficial for the HER.66
 |
| Fig. 3 The possible adsorption sites of H atom on adatom@silicene with the metal atom absorbed at the (a) H, (b) V, (c) B sites and (d) pristine silicene. | |
The free energy diagrams of alkali, alkali-earth, and transition metal atoms anchored to silicene for HER are shown in Fig. 4a, b, and c, respectively. The values of ΔGH decrease from 0.57 to 0.19, 0.20, and 0.27 eV for the Li, Na, and K@silicene, respectively, indicating that the catalytic performance can be improved by the alkali atom adsorption. However, these values of ΔGH are still larger than the optimum value of 0.0 eV, thus the alkali atom anchored to silicene is not a good catalyst for HER. The value of ΔGH is decreased for H adsorbed on alkali-earth atoms anchored to silicene. The values of ΔGH is 0.10 eV for hydrogen adsorbed on Be@silicene, indicating Be@silicene is a potential catalyst for HER. Most of the transition metal atoms anchored to silicene show good catalytic behavior for HER due to the small absolute value of |ΔGH|, such as 0.02, −0.01, −0.06 eV for hydrogen adsorbed on Co@silicene, Ti@silicene, and Mn@silicene, respectively.
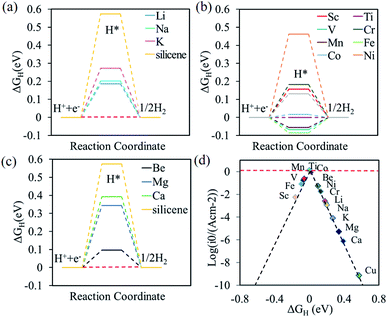 |
| Fig. 4 Gibbs free energy diagram of HER at the equilibrium potential for (a) alkali, (b) alkali-earth, and (c) transition metal atoms anchored silicene, (d) volcano curve of exchange current as a function of the Gibbs free energy. | |
The catalytic performance of HER is mainly determined by the adsorption ability of reaction intermediate (H*), which can be evaluated by the exchange current density as a function of Gibbs free energy of hydrogen adsorption in the shape of a volcano plot.67 Based on the assumption of Norskov,68 the theoretical exchange current i0 was calculated by using eqn (4) and (5) for ΔGH >0 and ΔGH < 0, respectively, at pH = 0,
|
 | (4) |
|
 | (5) |
where
k0 is the reaction rate constant,
kb is the Boltzmann constant, and
T is the temperature. The volcano curve for the alkali, alkali-earth, and transition metal atoms anchored to silicene is shown in
Fig. 4d. The catalytic activity is related to the positions of
i0 and Δ
GH, the closer the position of these values to the peak, the better the catalyst is. As can be seen from
Fig. 4d, the values of Δ
GH for Sc, Fe, Mn and V anchored to silicene are in the left side of the volcano curve. The value of Δ
GH for Sc@silicene is far away from the volcano peak, indicating the hydrogen is tightly bound to Sc@silicene. While other adatoms anchored to silicene are in the left side of the volcano curve, the values of Δ
GH for Li, Na, K, Mg, Ca, and Cu@silicene are with larger distance to the peak, indicating that the hydrogen is difficult to be bound to the catalyst. The Fe, V, Mn, Ti, Co, Ni, Be, and Cr are very close to the peak with maximum exchange current, indicating they are catalytically active for HER as they are anchored to the silicene.
As a good catalyst, the molecular hydrogen should be released from the catalyst site easily. The adsorption energy (Eads(H2)) of the molecular hydrogen on pristine, alkali, alkali-earth, and transition metal atoms anchored silicene was calculated using the eqn (6):
|
Eads(H2) = EH2@system − EH2 − Esystem
| (6) |
where
EH2@system and
Esystem are the total energies of pristine, alkali, alkali-earth, and transition metal atoms anchored silicene with and without H
2 adsorption, respectively.
EH2 is the energy of hydrogen molecule. According to the equation, the larger the adsorption energy is, the easier for the releasing of H
2 is.
69 All the four possible symmetry adsorption sites as H absorbed on the surface of adatom@silicene (as shown in
Fig. 1a and b) were considered for H
2 adsorption. The calculated adsorption energies and energy favorable sites are listed in
Table 1. The adsorption energies are −0.01, 0.00, 0.00, −0.02, −0.01, 0.00, −0.02, and 0.00 for the Fe, V, Mn, Ti, Co, Ni, Be, and Cr anchored silicene, respectively, indicating that these systems are highly active for the releasing H
2 gas.
4. Conclusion
In conclusion, the adsorption of alkali, alkali-earth, and transition metal adatoms on silicene as well as the catalytic performance of adatom anchored silicene for HER were investigated using DFT calculation. All the metal atoms are strongly bound to the silicene with large binding energy. The alkali adatoms (Li, Na, and K) are strongly bound to the H site, the alkali-earth atoms favors to be absorbed at the V site, the transition metal atoms except for Sc are strongly bounded to the H site while Sc is bound to the B site. The metal atoms adsorption can enhance the catalytic performance of silicene. The values of ΔGH are −0.01, −0.07, −0.06, −0.09 and 0.02 eV for Ti, V, Mn, Fe, and Co atoms anchored to silicene, respectively. These results suggest that the metal adatom (Ti, V, Mn, Fe, and Co) anchored to silicene offer promising low-cost catalysts for HER.
Conflicts of interest
There are no conflicts to declare.
References
- S. H. Hong, S. H. Ahn, I. Choi, S. G. Pyo, H. J. Kim, J. H. Jang and S. K. Kim, Appl. Surf. Sci., 2014, 307, 146–152 CrossRef CAS.
- P. P. Patel, P. J. Hanumantha, M. K. Datta, O. I. Velikokhatnyi, D. H. Hong, J. A. Poston, A. Manivannan and P. N. Kumta, Int. J. Hydrogen Energy, 2017, 42, 17049–17062 CrossRef CAS.
- J. Tymoczko, F. Calle-Vallejo, W. Schuhmann and A. S. Bandarenka, Nat. Commun., 2016, 7, 10990 CrossRef CAS PubMed.
- G. P. Gao, Y. Jiao, F. X. Ma, Y. L. Jiao, E. Waclawik and A. J. Du, J. Phys. Chem. C, 2015, 119, 13124–13128 CrossRef CAS.
- A. K. Kunhiraman and M. Ramasamy, J. Nanopart. Res., 2017, 19, 203 CrossRef.
- A. Eftekhari, Int. J. Hydrogen Energy, 2017, 42, 11053–11077 CrossRef CAS.
- J. J. Peng, L. X. Chen, P. Song, X. L. Wu, A. J. Wang and J. H. Yuan, Int. J. Hydrogen Energy, 2016, 41, 8839–8846 CrossRef.
- Y. F. Xiao, Y. Liu, Z. Tang, L. Wu, Y. Zeng, Y. F. Xu and Y. H. He, RSC Adv., 2016, 6, 51096–51105 RSC.
- T. F. Jaramillo, K. P. Jorgensen, J. Bonde, J. H. Nielsen, S. Horch and I. Chorkendorff, Science, 2007, 317, 100–102 CrossRef CAS PubMed.
- J. Kibsgaard, Z. B. Chen, B. N. Reinecke and T. F. Jaramillo, Nat. Mater., 2012, 11, 963–969 CrossRef CAS PubMed.
- L. L. Li, X. Y. Li, L. H. Ai and J. Jiang, RSC Adv., 2015, 5, 90265–90271 RSC.
- K. Wang, D. Xi, C. J. Zhou, Z. Q. Shi, H. Y. Xia, G. W. Liu and G. J. Qiao, J. Mater. Chem. A, 2015, 3, 9415–9420 RSC.
- J. Zhang, Q. Wang, L. H. Wang, X. A. Li and W. Huang, Nanoscale, 2015, 7, 10391–10397 RSC.
- D. S. Kong, H. T. Wang, J. J. Cha, M. Pasta, K. J. Koski, J. Yao and Y. Cui, Nano Lett., 2013, 13, 1341–1347 CrossRef CAS PubMed.
- C. F. Du, Q. H. Liang, R. Dangol, J. Zhao, H. Ren, S. Madhavi and Q. Y. Yan, Nano-Micro Lett., 2018, 10, 67 CrossRef PubMed.
- X. P. Ren, Q. Ma, H. B. Fan, L. Q. Pang, Y. X. Zhang, Y. Yao, X. D. Ren and S. Z. Liu, Chem. Commun., 2015, 51, 15997–16000 RSC.
- Y. Li, J. Wang, X. K. Tian, L. L. Ma, C. Dai, C. Yang and Z. X. Zhou, Nanoscale, 2016, 8, 1676–1683 RSC.
- C. H. Mu, H. X. Qi, Y. Q. Song, Z. P. Liu, L. X. Ji, J. G. Deng, Y. B. Liao and F. Scarpa, RSC Adv., 2016, 6, 23–30 RSC.
- J. L. Jin, Y. Z. Zhu, Y. Z. Liu, Y. Li, W. C. Peng, G. L. Zhang, F. B. Zhang and X. B. Fan, Int. J. Hydrogen Energy, 2017, 42, 3947–3954 CrossRef CAS.
- X. L. Zhou, Y. Liu, H. X. Ju, B. C. Pan, J. F. Zhu, T. Ding, C. D. Wang and Q. Yang, Chem. Mater., 2016, 28, 1838–1846 CrossRef CAS.
- L. J. Yang, W. J. Zhou, J. Lu, D. M. Hou, Y. T. Ke, G. Q. Li, Z. H. Tang, X. W. Kang and S. W. Chen, Nano Energy, 2016, 22, 490–498 CrossRef CAS.
- W. Shi and Z. Wang, J. Taiwan Inst. Chem. Eng., 2018, 82, 163–168 CrossRef CAS.
- D. Voiry, H. Yamaguchi, J. W. Li, R. Silva, D. C. B. Alves, T. Fujita, M. W. Chen, T. Asefa, V. B. Shenoy, G. Eda and M. Chhowalla, Nat. Mater., 2013, 12, 850–855 CrossRef CAS PubMed.
- W. W. Shi, Z. G. Wang and Y. Q. Fu, J. Nanopart. Res., 2017, 19, 296 CrossRef.
- W. W. Shi, Z. G. Wang and Y. Q. Fu, J. Phys. D: Appl. Phys., 2017, 50, 405303 CrossRef.
- C. Berger, Z. M. Song, X. B. Li, X. S. Wu, N. Brown, C. Naud, D. Mayou, T. B. Li, J. Hass, A. N. Marchenkov, E. H. Conrad, P. N. First and W. A. de Heer, Science, 2006, 312, 1191–1196 CrossRef CAS.
- T. Shirai, T. Shirasawa, T. Hirahara, N. Fukui, T. Takahashi and S. Hasegawa, Phys. Rev. B: Condens. Matter Mater. Phys., 2014, 90, 039902 CrossRef.
- L. C. L. Y. Voon, J. J. Zhu and U. Schwingenschlogl, Appl. Phys. Rev., 2016, 3, 040802 Search PubMed.
- T. P. Kaloni, Y. C. Cheng and U. Schwingenschlogl, J. Appl. Phys., 2013, 113, 104305 CrossRef.
- Z. Y. Ni, Q. H. Liu, K. C. Tang, J. X. Zheng, J. Zhou, R. Qin, Z. X. Gao, D. P. Yu and J. Lu, Nano Lett., 2012, 12, 113–118 CrossRef CAS PubMed.
- L. Tao, E. Cinquanta, D. Chiappe, C. Grazianetti, M. Fanciulli, M. Dubey, A. Molle and D. Akinwande, Nat. Nanotechnol., 2015, 10, 227–231 CrossRef CAS PubMed.
- J. M. Zhang, W. T. Song, K. W. Xu and V. Ji, Comput. Mater. Sci., 2014, 95, 429–434 CrossRef CAS.
- X. X. Guo, P. Guo, J. M. Zheng, L. K. Cao and P. J. Zhao, Appl. Surf. Sci., 2015, 341, 69–74 CrossRef CAS.
- Y. P. Huang, J. M. Yuan, G. Guo and Y. L. Mao, Acta Phys. Sin., 2015, 64, 013101 Search PubMed.
- F. Li, C. W. Zhang, H. X. Luan and P. J. Wang, J. Nanopart. Res., 2013, 15, 1972 CrossRef.
- X. Q. Lin and J. Ni, Phys. Rev. B: Condens. Matter Mater. Phys., 2012, 86, 075440 CrossRef.
- H. Sahin and F. M. Peeters, Phys. Rev. B: Condens. Matter Mater. Phys., 2013, 87, 085423 CrossRef.
- A. Wang, J. Li and T. Zhang, Nat. Rev. Chem., 2018, 2, 65–81 CrossRef CAS.
- N. C. Cheng, S. Stambula, D. Wang, M. N. Banis, J. Liu, A. Riese, B. W. Xiao, R. Y. Li, T. K. Sham, L. M. Liu, G. A. Botton and X. L. Sun, Nat. Commun., 2016, 7, 13638 CrossRef CAS PubMed.
- X. G. Li, W. T. Bi, L. Zhang, S. Tao, W. S. Chu, Q. Zhang, Y. Luo, C. Z. Wu and Y. Xie, Adv. Mater., 2016, 28, 2427–2431 CrossRef CAS PubMed.
- J. Deng, H. B. Li, J. P. Xiao, Y. C. Tu, D. H. Deng, H. X. Yang, H. F. Tian, J. Q. Li, P. J. Ren and X. H. Bao, Energy Environ. Sci., 2015, 8, 1594–1601 RSC.
- J. Deng, H. B. Li, S. H. Wang, D. Ding, M. S. Chen, C. Liu, Z. Q. Tian, K. S. Novoselov, C. Ma, D. H. Deng and X. H. Bao, Nat. Commun., 2017, 8, 14430 CrossRef CAS PubMed.
- Y. Shi, Y. Zhou, D. R. Yang, W. X. Xu, C. Wang, F. B. Wang, J. J. Xu, X. H. Xia and H. Y. Chen, J. Am. Chem. Soc., 2017, 139, 15479–15485 CrossRef CAS PubMed.
- J. W. Miao, F. X. Xiao, H. B. Yang, S. Y. Khoo, J. Z. Chen, Z. X. Fan, Y. Y. Hsu, H. M. Chen, H. Zhang and B. Liu, Sci. Adv., 2015, 1, 1500259 CrossRef PubMed.
- X. Sun, J. Dai, Y. Q. Guo, C. Z. Wu, F. T. Hu, J. Y. Zhao, X. C. Zeng and Y. Xie, Nanoscale, 2014, 6, 8359–8367 RSC.
- H. T. Wang, C. Tsai, D. S. Kong, K. R. Chan, F. Abild-Pedersen, J. Norskov and Y. Cui, Nano Res., 2015, 8, 566–575 CrossRef CAS.
- B. R. Xia, L. An, D. Q. Gao, S. P. Shi, P. X. Xi and D. S. Xue, CrystEngComm, 2015, 17, 6420–6425 RSC.
- S. Xu, X. F. Fan, J. L. Liu, D. J. Singh, Q. Jiang and W. T. Zheng, Phys. Chem. Chem. Phys., 2018, 20, 8887–8896 RSC.
- D. Sanchez-Portal, P. Ordejon and E. Canadell, Principles and Applications of Density in Inorganic Chemistry II, 2004, vol. 113, pp. 103–170 Search PubMed.
- J. M. Soler, E. Artacho, J. D. Gale, A. Garcia, J. Junquera, P. Ordejon and D. Sanchez-Portal, J. Phys.: Condens. Matter, 2002, 14, 2745–2779 CrossRef CAS.
- R. A. Evarestov and V. P. Smirnov, Phys. Rev. B: Condens. Matter Mater. Phys., 2004, 70, 233101 CrossRef.
- J. K. Norskov, J. Rossmeisl, A. Logadottir, L. Lindqvist, J. R. Kitchin, T. Bligaard and H. Jonsson, J. Phys. Chem. B, 2004, 108, 17886–17892 CrossRef CAS.
- Y. A. Zhou, G. P. Gao, Y. Li, W. Chu and L. W. Wang, Phys. Chem. Chem. Phys., 2019, 21, 3024–3032 RSC.
- T. W. He, C. M. Zhang and A. J. Du, Chem. Eng. Sci., 2019, 194, 58–63 CrossRef CAS.
- I. G. Shuttleworth, J. Phys. Chem. Solids, 2013, 74, 128–134 CrossRef CAS.
- I. G. Shuttleworth, J. Phys. Chem. Solids, 2015, 86, 19–26 CrossRef CAS.
- M. Houssa, A. Dimoulas and A. Molle, J. Phys.: Condens. Matter, 2015, 27, 253002 CrossRef CAS PubMed.
- C. C. Lee, A. Fleurence, R. Friedlein, Y. Yamada-Takamura and T. Ozaki, Phys. Rev. B: Condens. Matter Mater. Phys., 2013, 88, 165004 Search PubMed.
- M. Houssa, G. Pourtois, V. V. Afanas'ev and A. Stesmans, Appl. Phys. Lett., 2010, 97, 112106 CrossRef.
- Z. X. Guo, S. Furuya, J. Iwata and A. Oshiyama, Phys. Rev. B: Condens. Matter Mater. Phys., 2013, 87, 235435 CrossRef.
- X. K. Gu and R. G. Yang, J. Appl. Phys., 2015, 117, 025435 Search PubMed.
- H. Zabel, J. Phys.: Condens. Matter, 2001, 13, 7679–7690 CrossRef CAS.
- K. T. Chan, J. B. Neaton and M. L. Cohen, Phys. Rev. B: Condens. Matter Mater. Phys., 2008, 77, 235430 CrossRef.
- J. J. Duan, S. Chen, M. Jaroniec and S. Z. Qiao, ACS Catal., 2015, 5, 5207–5234 CrossRef CAS.
- T. T. Liu, S. Wang, Q. J. Zhang, L. Chen, W. H. Hu and C. M. Li, Chem. Commun., 2018, 54, 3343–3346 RSC.
- S. S. Ding, P. He, W. R. Feng, L. Li, G. L. Zhang, J. C. Chen, F. Q. Dong and H. C. He, J. Phys. Chem. Solids, 2016, 91, 41–47 CrossRef CAS.
- A. Jain, Y. Shin and K. A. Persson, Nat. Rev. Mater., 2016, 1, 15004 CrossRef CAS.
- J. K. Norskov, T. Bligaard, A. Logadottir, J. R. Kitchin, J. G. Chen, S. Pandelov and J. K. Norskov, J. Electrochem. Soc., 2005, 152, J23–J26 CrossRef CAS.
- H. Zhu, J. F. Zhang, R. P. Yanzhang, M. L. Du, Q. F. Wang, G. H. Gao, J. D. Wu, G. M. Wu, M. Zhang, B. Liu, J. M. Yao and X. W. Zhang, Adv. Mater., 2015, 27, 4752–4759 CrossRef CAS PubMed.
|
This journal is © The Royal Society of Chemistry 2019 |
Click here to see how this site uses Cookies. View our privacy policy here.