DOI:
10.1039/C9RA03931G
(Paper)
RSC Adv., 2019,
9, 20415-20423
Highly effective transformation of methyl phenyl carbonate to diphenyl carbonate with recyclable Pb nanocatalyst†
Received
24th May 2019
, Accepted 12th June 2019
First published on 1st July 2019
Abstract
Diphenyl carbonate (DPC) is a type of versatile industrial chemical, and the disproportionation of methyl phenyl carbonate (MPC) is a key step to produce DPC. However, the design and formulation of a catalyst for the efficient synthesis of DPC is a major challenge due to its small equilibrium constant. The support material is a critical factor influencing the performance of Pb nanocatalysts. Thus, a series of Pb-based catalysts over MgO, ZrO2, SiO2, TiO2 and Al2O3 were prepared to investigate the effect of the support materials on the physicochemical properties and catalytic performances for the conversion of MPC to effectively synthesize DPC. The catalysts were well characterized by XRD, BET, TEM, XPS, ICP-OES, H2-TPR, Py-IR and NH3-TPD. The results showed that the nature of the support obviously affected the structural properties and catalytic performances, and Pb was dispersed better on SiO2, TiO2, ZrO2 and MgO than on Al2O3, and showed stronger metal-support interaction over MgO and ZrO2. The activity results revealed that PbO/MgO and PbO/ZrO2 exhibited higher catalytic activities because they contained higher Pb dispersion and more Lewis acid sites, and the catalytic activities followed the order PbO/MgO > PbO/ZrO2 > PbO/SiO2 > PbO/Al2O3 > PbO/TiO2. On the contrary, PbO/MgO and PbO/ZrO2 exhibited better reusability due to strong interaction between the highly dispersed Pb and the supports, and the activity decrease in the case of PbO/SiO2, PbO/Al2O3 and PbO/TiO2 mainly resulted from the Pb leaching loss. This work would contribute to exploiting novel catalytic materials in a wide range of applications for the efficient synthesis of organic carbonates.
1. Introduction
Due to its outstanding electrical, mechanical and heat resistance properties, polycarbonate (PC), as an important engineering thermoplastic for ubiquitous applications in several industries (automobiles, office equipment, medical devices, etc.), has attracted considerable interest in the last decades.1,2 Diphenyl carbonate (DPC), a building block, was often employed for the phosgene-free synthesis of PC by melt transesterification with bisphenol-A, and was also used for the production of pharmaceuticals, polymer materials, fine chemicals, etc.3–5 It was, therefore, necessary to develop efficient and environmentally benign synthetic routes to replace the highly toxic synthesis via phosgene. Among the alternative routes (phosgene method,6,7 oxidative carbonylation,8,9 transesterification,10,11 etc.), the transesterification of phenol and dimethyl carbonate (DMC) was regarded as a most likely pathway to synthesize DPC without employing phosgene since the starting materials presented low toxicity and high biodegradability. This route consisted in two-step reaction where methyl phenyl carbonate (MPC) of the intermediate was first produced by the transesterification (Scheme 1-(1)), followed by the further transesterification reaction of MPC and phenol (Scheme 1-(2)) or the disproportionation of MPC (Scheme 1-(3)). Concerning the second-step reaction the equilibrium constants K2 and K3 indicated that DPC was mainly produced by the second step disproportionation instead of the further transesterification.12,13 On the contrary, the first step was rate-determining step since K1 was much lower than K3. Hence, considerable studies on the first step transesterification have been particularly carried out,14–17 but only few efforts have been made to the second step disproportionation.18,19 Indeed, the disproportionation of MPC is also the key step to produce DPC. Overall, it is very significant to intensively study on MPC disproportionation, which is advantageous to improving the yield of DPC and the conversion of the starting materials in future industrial applications.
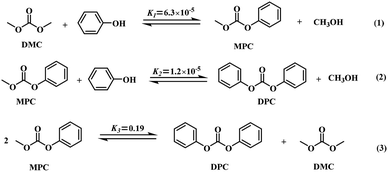 |
| Scheme 1 Transesterification process between DMC and phenol into DPC. | |
The disproportionation reaction suffered from the low yield and selectivity of DPC due to the relatively low equilibrium constant and reaction rate. Therefore, the development of highly active catalysts is crucial in this reaction. Among various homogeneous catalytic systems reported like organo-tin/titanium catalysts, even though they exhibited outstanding catalytic activities,19,20 they had received little consideration because of the separation problems between DPC and the catalysts. Therefore, heterogeneous catalysis currently attracted more attention because these catalysts might be facilely recovered from the reaction mixture by simple filtration and could be recycled, making the procedure more feasible.
Recently, we studied the catalytic performances of various metal oxides including TiO2, MoO3, PbO and so on,21–23 and found that PbO was prepared into the supported Pb catalysts or mixed metal oxides significantly improved the catalytic activity, life time and stability.24,25 However, the nature and property of the support is often found to modify the distribution, loading, size, shape and electronic state of the active species as well as their interactions with support with some special physical–chemical properties, which is well known to strongly affect the catalytic activity and stability.26–31 Therefore, the study to investigate the structure–function relationship of the supports on Pb-based catalysts for MPC disproportionation is very worthwhile, which is conducive to screen suitable and promising supports.
In the present work, we formulate Pb-based catalysts by employing potential metal oxides as support phases and focus on studying the effect of various supports on the physicochemical properties of the catalysts and their catalytic performance to provide the role of support materials for developing efficient catalysis materials for MPC disproportionation to effectively synthesize DPC. Herein the Pb-based catalysts are prepared using wet-impregnation process over PbO loading onto SiO2, TiO2, Al2O3, MgO and ZrO2 supports. At the same time, the catalysts are thoroughly characterized by X-ray diffraction (XRD), temperature programmed reduction of H2 (H2-TPR), transmission electron microscopy (TEM), X-ray photoelectron spectrum (XPS), N2 adsorption/desorption measurement, pyridine-infrared spectroscopy (Py-IR) and temperature programmed desorption of NH3 (NH3-TPD) to clarify the support role on the Pb-based catalysts. In addition, the stabilities of these catalysts are also investigated in detail.
2. Experimental section
2.1 Material preparation
All chemicals adopted in this work were analytical purity, which were purchased from Sinopharm Chemical Reagent Co., Ltd and were directly used as received without further purification. Deionized water was used in all experimental synthesis procedures as needed.
Pure SiO2 was synthesized by sol–gel process. For this preparation, the desired amounts of tetraethyl orthosilicate, ethanol and distilled water were mixed together at room temperature. Then the ammonium aqueous solution was added gradually into the above solution under stirring at 50 °C to generate a transparent gel. The obtained gel was aged at 50 °C overnight, dried at 110 °C for 12 h and subsequently calcinated 550 °C for 5 h.
Pure TiO2 was prepared by hydrolysis process. Briefly, a proper amount of tetrabutyl titanate was dissolved in ethanol, and heated up to 50 °C under stirring. Then, appropriate amount of water was slowly added to promote the hydrolysis and was kept for 24 h. Finally, the sediment was filtered, washed with distilled water, and dried at 110 °C for 12 h and calcinated at 550 °C for 5 h.
Pure Al2O3 was prepared by a precipitation process. A certain amount of aluminum nitrate nonahydrate were dissolved in the deionized water and heated slowly to 90 °C. Afterwards, ammonia solution with 25% concentration was added into this solution under stirring until that the pH of 9–10 was reached. The as-prepared solid sediment was aged for 24 h and then vacuum filtered off and washed repeatedly. Finally, the obtained samples were dried at 110 °C for 12 h and subsequently calcinated at 550 °C for 5 h. Likewise, pure MgO and ZrO2 were prepared by using the similar process, respectively.
The preparation of supported Pb-based catalysts was carried out by incipient wetness impregnation process. Briefly, SiO2, TiO2, Al2O3, ZrO2 and MgO supports were homogeneously impregnated by using the aqueous solution of lead nitrate as precursor salt at ambient temperature for 24 h, respectively. After impregnation, the samples were dried in air at 110 °C for 12 h, and finally calcinated at 550 °C for 5 h. And the PbO loading used was 10 wt% for all the catalysts.
2.2 Material characterization
The X-ray powder diffraction (XRD) analysis was carried out on a DX-2700B diffractometer with the Ni-filtered CuKα radiation (1.5418 Å). The X-ray tube was operated at 40 kV and 30 mA, and the powder diffractogram was recorded at 0.02° intervals in the range of 5–90° with the scanning rate of 1.2° min−1.
The determination of the elemental composition was conducted by Optima 2000 DV inductively coupled plasma-optical emission spectrometer (PerkinElmer Inc., USA).
N2 adsorption–desorption isotherms were measured at liquid nitrogen temperature (−196 °C) using a 3H-2000PS2 adsorption instrument (BeiShiDe Instrument). Before the measurements, the catalysts were pretreated at 250 °C for 6 h under a high vacuum environment to remove physically adsorbed water. The specific surface area was calculated using the Brunauer–Emmett–Teller (BET) method and the pore volume and porous distribution was derived from Barrett–Joyner–Halenda (BJH) equation.
The morphology of the catalysts was investigated by transmission electron microscopy (JEM-1011) with a field-emission gun operating at 200 kV. The samples were prepared by dropping an ethanol suspension of the powder particles on a carbon film supported copper grid.
X-ray photoelectron spectrum (XPS) was employed to detect the chemical composition and states of the atoms on the catalysts, and conducted on a ESCALAB250Xi X-ray photoelectron spectrometer with C as the internal standard (C 1s = 284.7 eV).
H2-TPR experiment was carried out on a Micro TP-5076 chemisorption analyzer. Prior to the reduction, the catalyst (about 50 mg) was pretreated in N2 stream (20 mL min−1) at 300 °C for 1.5 h and then cooled to room temperature. After that, the flowing H2–N2 mixture (10% H2 by volume) was switched on, and the temperature was gradually increased from 50 to 800 °C with a ramp of 10 °C min−1. The H2 consumption was measured with a thermal conduction detector (TCD) that had been calibrated using the standard sample of CuO.
The number of acid sites was determined by NH3-TPD using the same instrument as that used for H2-TPR. Before the adsorption of NH3, the sample (about 100 mg) was first treated at 300 °C for 1.5 h and then decreased to 50 °C in a N2 flow of 20 mL min−1. After the saturated adsorption of NH3 (10 mL min−1) at 50 °C for 30 min, the physically adsorbed NH3 was subsequently degassed from the sample for 1 h under the N2 flow (20 mL min−1). Finally, the sample was heated to 800 °C with a rate of 10 °C min−1, the amounts of desorbed NH3 was monitored by the thermal conduction detector (TCD). The TPD profile was decomposed into Gaussian curves to quantify the weak, medium and strong acid sites.
Py-IR spectra were measured on a Thermo Nicolet 380 FT-IR spectrometer to determine the type of acid sites. Before the measurement, the impurity was removed from the sample under 10−2 Pa condition at 200 °C for 2 h, and then pyridine vapor was introduced into the sample for the saturated adsorption. After the evacuation at 200 °C for 1 h in flowing He atmosphere, the Py-IR spectra were subsequently collected at room temperature.
2.3 Catalytic reaction and the product analysis
MPC was synthesized by the reversible disproportionation of DMC and DPC, and its purity was 99.8% determined by high performance liquid chromatography.20 In a typical experiment, the catalytic performance test of MPC was performed in a three-necked glass beaker at atmospheric pressure. MPC of 150 mmol and a proper amount of the catalysts were placed into the beaker under a flowing nitrogen and slowly heated under vigorous stirring. When the reaction temperature was reached, it was kept for the reaction. As the reaction processed, DMC might be distilled out and continuously collected in a flask beaker to promote the equilibrium reaction towards the production of DPC. Upon the completion of the reaction, the reaction was decreased down to ambient temperature. The reaction mixture was analyzed by GC-MS instrument (HP-6890/5973) equipped with HP-5 capillary chromatography packed column. Subsequently, the products were quantitatively analyzed with a gas chromatograph (GC-7890A) equipped with HP-5 capillary column (30 m × 0.32 mm × 0.25 μm) and a flame ionization detector (FID).
3. Results and discussion
3.1 Characterization of the catalysts
3.1.1 BET characterization. The BET surface area, pore volume and pore size distribution determined by nitrogen adsorption–desorption measurement for all the catalysts and their corresponding supports were listed in Table 1. The average pore diameters for these materials were in the mesoporous range, and the surface area and pore volume of the catalysts decreased in comparison to the corresponding supports. It was noted that the SiO2 support displayed the highest BET surface area among all support materials, moreover, after impregnating, the PbO/SiO2 catalyst also exhibited a larger BET specific surface area and pore volume in contrast to PbO/MgO, PbO/Al2O3, PbO/ZrO2 and PbO/TiO2, and the orders of both the surface area and pore volume for them were as follows: PbO/SiO2 > PbO/ZrO2 > PbO/MgO > PbO/TiO2 > PbO/Al2O3, in the same orders as that for the pure supports. The average pore diameters of the catalysts also decreased than those of the pure supports. This was probably due to PbO being dispersed on the surface of these supports or filled in the pore structure after the lead impregnating, which might result in the decrease of these textural properties.
Table 1 Results of BET measurements for the catalysts and corresponding supports
Catalysts |
BET surface area (m2 g−1) |
Total pore volume (cm3 g−1) |
Average pore diameter (nm) |
PbO/MgO |
34.5 |
0.157 |
9.0 |
MgO |
36.9 |
0.182 |
11.3 |
PbO/Al2O3 |
20.7 |
0.049 |
7.9 |
Al2O3 |
24.8 |
0.070 |
11.6 |
PbO/ZrO2 |
43.3 |
0.106 |
8.2 |
ZrO2 |
44.3 |
0.098 |
8.8 |
PbO/TiO2 |
30.3 |
0.073 |
9.9 |
TiO2 |
33.4 |
0.074 |
11.3 |
PbO/SiO2 |
67.3 |
0.522 |
11.1 |
SiO2 |
95.4 |
0.747 |
12.3 |
3.1.2 XRD characterization. To detect the structures of Pb species on various supports, XRD measurement were performed for both the catalysts and the pure supports. As could be seen in Fig. 1, XRD patterns of pure supports exhibited their respective characteristic peaks, while there were some differences with their corresponding Pb-based catalysts among the characteristic peaks. For instance, SiO2 exhibited a broad peak at around 22°, and after PbO loading on its surface no PbO crystalline phase diffraction peak was observed for PbO/SiO2. The diffraction peak at 25.4, 37.9, 48.1, 54.1 and 62.5° were attributed to anatase TiO2, and analogously the characteristic peak ascribed to PbO phases was absent for PbO/TiO2. ZrO2 displayed the existence of monoclinic (2θ = 30.3°) and tetragonal (2θ = 24.3 and 31.5°) zirconia phases, and PbO/ZrO2 exhibited the same structure with that of ZrO2 support. The diffraction peak at 25.5° belonging to γ-Al2O3 support was clearly observed,32,33 while PbO crystalline phases appeared at 20.8, 25.4, 26.6, 27.7 and 29.7° for PbO/Al2O3. PbO/MgO exhibited also the same crystalline structure with those of MgO support, and no PbO diffraction peak was observed. The XRD results suggested that the PbO active phases had better dispersion on the surface of SiO2, TiO2, ZrO2 and MgO supports in an amorphous state than that on Al2O3.
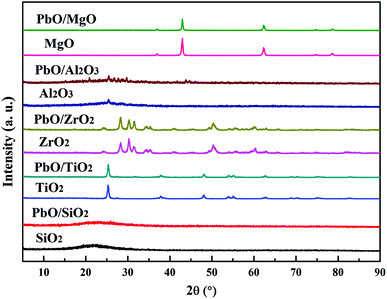 |
| Fig. 1 XRD patterns of the catalysts and corresponding supports. | |
3.1.3 H2-TPR characterization. H2-TPR measurement was conducted to investigate the reduction behaviors of PbO species over the catalysts. The H2-TPR profiles of both the catalysts and pure supports were shown in Fig. 2. Firstly, for SiO2, ZrO2 and MgO supports, there were no evident reduction peak appearances in the temperature ranges of 100–800 °C. By contrast, the reduction characteristic peaks at 730 and 781 °C were observed for pure TiO2 and Al2O3, respectively. Secondly, for various Pb-based catalysts, only one distinct reduction characteristic peak was observed in PbO/SiO2, PbO/TiO2, PbO/ZrO2 and PbO/MgO respectively, suggesting that mainly PbO was reduced. As reported in the literatures,25,34 pure PbO revealed a clear reduction peak of 588 °C, which was assigned to PbO species reduction. Therefore, these peaks in PbO/SiO2 (552 °C), PbO/TiO2 (591 °C), PbO/ZrO2 (348 °C) and PbO/MgO (410 °C) were typically ascribed to the reduction of highly dispersed surface PbO species, nevertheless, it was noteworthy that there were also obvious differences in the peak positions for them, suggesting that PbO loading onto different supports had a great influence on the reduction temperature of PbO species. Notably, the reduction temperature of PbO species on SiO2, ZrO2 and MgO supports were shifted to lower temperatures. This was mainly due to the strong interaction between PbO species and the supports which was favorable for the stability of the catalysts during reaction. Whereas in the case of PbO/TiO2 the reduction peak shifted to a higher temperature and this was mainly due to the poor interaction between PbO and the TiO2 support. Thus, the results suggested that the interaction strengths between the active component and the different supports were remarkably different.
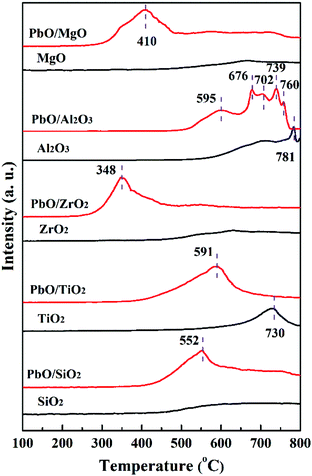 |
| Fig. 2 H2-TPR profiles of the catalysts and corresponding supports. | |
Thirdly, for PbO/Al2O3, five reduction characteristic peaks appeared. The first reduction peak at 595 °C corresponded to the surface PbO specie reduction, and the second reduction peak at 676 °C might result from the reduction of the bulk PbO, as the XRD results displayed the existence of PbO crystalline diffraction peaks, which was primarily assigned to the weak interaction between PbO and the Al2O3 support so that the PbO species was difficult to reduce at a higher reduction temperature. The following three peaks with reduction temperatures at 702, 739 and 760 °C were probably associated with the reduction of the Pb–Al mixed species formed via the interaction at high temperature (>700 °C) of partially reduced Pb-species and the support.35,36 Overall, according to the reduction temperatures of PbO species, it could be inferred that the interaction strengths between PbO and the supports were in the following order of PbO/ZrO2 > PbO/MgO > PbO/SiO2 > PbO/TiO2 > PbO/Al2O3, which might lead to different catalytic properties.
3.1.4 TEM characterization. Fig. 3 displayed the TEM images of the catalysts. As shown in Fig. 3(A and a), PbO/SiO2 mainly represented the spherical-like structure, and the PbO particles might be clearly observed, indicating that they were highly dispersed on the surface of the SiO2. For PbO/TiO2, PbO/ZrO2, PbO/Al2O3 and PbO/MgO displayed in Fig. 3(B, b)–(E, e), their morphology were remarkably different from each other. The diameters of all these catalysts were also not uniform, and the dissociation of PbO particles and the supports could not be detected by visual inspection. Comparatively speaking, PbO/ZrO2 exhibited a smaller particle size than the other catalysts.
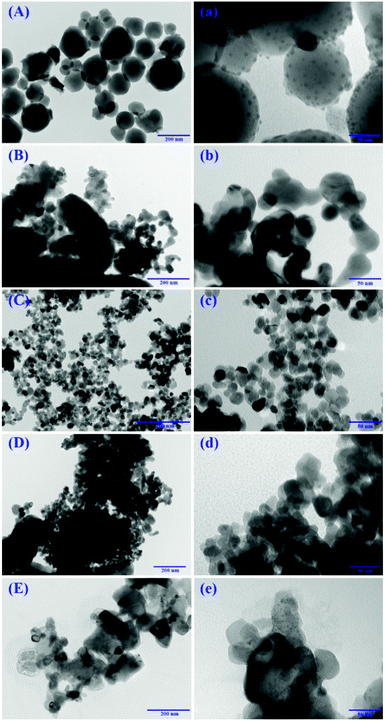 |
| Fig. 3 TEM pictures of PbO/SiO2 (A and a), PbO/TiO2 (B and b), PbO/ZrO2 (C and c), PbO/Al2O3 (D and d) and PbO/MgO (E and e). | |
3.1.5 XPS characterization. XPS analyses were employed to identify the chemical composition and states of the catalysts. The XPS survey spectrum verified the presence of Pb in the supported catalysts. As shown in Fig. 4(A), the definitive binding energy peak located at approximately 142.9–144.0 eV and 137.9–139.2 eV corresponded to Pb 4f5/2 and Pb 4f7/2,23,37 indicating that Pb2+ species existed on the surface of these catalysts. Fig. 4(B) showed that the O 1s spectra of the catalysts (except PbO/Al2O3 catalyst) could be deconvoluted into two Gaussian peaks (OI and OII). In general, OI was ascribed to the lattice oxygen in the literature,28,34 and OII was related to the adsorbed oxygen species on the catalyst surface. The binding energy of the O 1s spectra was different in each catalyst, which was probably due to the different support properties for the supported Pb-based catalysts.
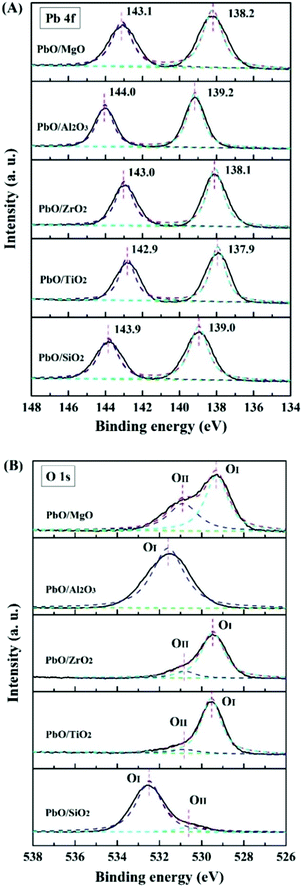 |
| Fig. 4 XPS spectra of the catalysts. | |
3.1.6 NH3-TPD characterization. The process of MPC disproportionation to produce DPC was mainly dependent on the acidity of the catalyst. Herein NH3-TPD was carried out for measuring the acidic properties of the catalysts. The NH3-TPD profiles were displayed in Fig. 5. According to the literatures,38,39 the acid sites of the catalysts were usually classified based on desorption temperature range such as weak (around 100–200 °C), medium (around 200–400 °C), and strong (above 400 °C) acidic sites, respectively. As shown in Fig. 3, the acidity of the catalysts depended on the nature of the supports, and PbO/SiO2, PbO/TiO2 and PbO/ZrO2 exhibited remarkable and broad NH3 desorption peaks, suggesting the wide distribution of acid strengths from weak, medium and strong acid sites respectively. For PbO/MgO catalyst, only one strong high-temperature NH3 desorption peak at the range of 250 to 700 °C was detected, suggesting a strong acid property. PbO/Al2O3 showed much stronger NH3 desorption peak in the high-temperature region, indicating more strong acidic sites existed on the surface. Besides, the acidity data was also calculated and the results were given in Table 2. Clearly, the total acid amount in PbO/MgO was remarkably higher than those of other catalysts, and followed the sequence of PbO/MgO > PbO/ZrO2 > PbO/SiO2 > PbO/TiO2 > PbO/Al2O3. According to previous studies, the acidity of the catalysts was correlated with the activity, thus the high acidity of the catalyst was beneficial for the activity.
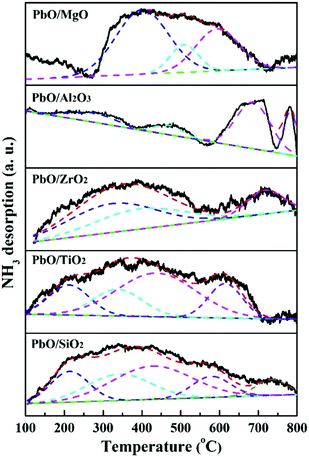 |
| Fig. 5 NH3-TPD profiles of the catalysts. | |
Table 2 The acidity of the catalysts determined by NH3-TPD
Catalysts |
Acid amounts (mmol g−1) |
Total acid amounts (mmol g−1) |
AL/AB ratio |
Weak |
Medium |
Strong |
PbO/MgO |
0 |
0.030 |
0.048 |
0.078 |
0.582 |
PbO/Al2O3 |
0 |
0.001 |
0.009 |
0.010 |
— |
PbO/ZrO2 |
0.004 |
0.019 |
0.029 |
0.052 |
0.610 |
PbO/TiO2 |
0.002 |
0.004 |
0.017 |
0.023 |
0.579 |
PbO/SiO2 |
0.002 |
0.014 |
0.020 |
0.036 |
0.489 |
3.1.7 Py-IR characterization. The type of acid sites was performed by pyridine adsorption FT-IR spectroscopy. Fig. 6 showed the Py-IR spectra of the catalysts. As shown in Fig. 6, PbO/SiO2, PbO/TiO2, PbO/ZrO2 and PbO/MgO exhibited two remarkable absorption peaks at around 1438–1446 and 1584–1596 cm−1, which were attributed to Lewis and Brønsted acidic sites, respectively.40,41 However, for PbO/Al2O3, only one absorption peak was displayed at 1446 cm−1 that suggested the presence of only Lewis acidic sites. Meanwhile, the concentration ratio of Lewis to Brønsted acid sites was calculated by semiquantitative analysis based on the relative peak area corresponding to Lewis and Brønsted acidity (AL/AB) in Table 2. Apparently, the AL/AB ratios were higher for PbO/ZrO2 and PbO/MgO compared to others, and combined with the acid amount results determined by NH3-TPD, which indicated that PbO/ZrO2 and PbO/MgO contained much more Lewis acidic sites.
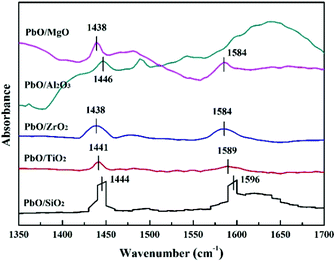 |
| Fig. 6 Py-IR spectra of the catalysts. | |
3.2 Results of the catalytic performance evaluation
The catalytic performance evaluation results for MPC disproportionation over the catalysts were revealed in Table 3. Pure supports were basically inactive under the current studies, while PbO loading on these supports exhibited considerably different catalytic performances. The catalytic activities of PbO/MgO and PbO/ZrO2 were close and obviously higher than those of PbO/SiO2, PbO/Al2O3 and PbO/TiO2, and the sequence of the activities for the MPC conversion was as follows: PbO/MgO > PbO/ZrO2 > PbO/SiO2 > PbO/Al2O3 > PbO/TiO2. Previous studies displayed that the BET surface area and acidity of catalyst played significant role in the catalytic activity and product selectivity. Nevertheless, this order of the activity was not correlated with the sequence of BET surface area, indicating that the surface area might be not a main factor determining the activity. Therefore, the catalytic activity might be closely related to the acidity. Combining with the characteristic results of Py-IR and NH3-TPD, the relationship between the activity and Lewis acidity was displayed in Fig. 7. It was clear that the activity depended on the acidic site, and the more Lewis acid site was, the higher the activity was. And the decrease sequence of MPC conversion was in accordance with the order of the Lewis acid site, suggesting that the Lewis acid was the critical factor to determine the catalytic activity. What's more, although the highest DPC yield was obtained over PbO/MgO due to the acidity of this catalyst being the highest among all the catalysts, yet it was also noteworthy that a strong acid strength could induce the decarboxylation decomposition of MPC into the byproduct anisole. Therefore, it could be ascertained that the higher anisole content was mainly due to its excessively strong acidity.20 Moreover, PbO/Al2O3 with more strong acid strength exhibited the higher anisole content, which further testified the reason. Thus, the results also suggested that medium and moderately strong acidic sites were favorable for the higher DPC selectivity.
Table 3 Catalytic performance results of MPC disproportionation over the catalystsa
Catalysts |
MPC conversion/% |
Yield/% |
DPC selectivity/% |
DPC |
Anisole |
Reaction conditions: MPC (150 mmol), catalyst (1.2 g), 200 °C, 2.5 h. Anisole was a small amount of byproduct. |
PbO/MgO |
60.1 |
56.0 |
4.1 |
93.2 |
PbO/ZrO2 |
56.3 |
55.2 |
1.1 |
98.0 |
PbO/SiO2 |
47.7 |
46.2 |
1.6 |
96.9 |
PbO/Al2O3 |
30.2 |
26.5 |
3.7 |
87.7 |
PbO/TiO2 |
24.3 |
22.9 |
1.4 |
94.2 |
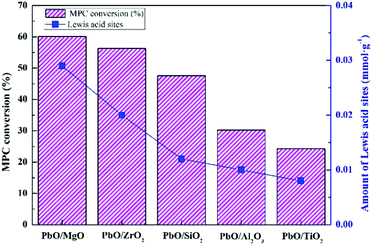 |
| Fig. 7 MPC conversion and Lewis acid sites on the catalysts. | |
3.3 Recyclability of the catalysts
Recycling experiments of the catalysts were performed to check the stability. After each run, the catalysts were separated by filtration, washed thoroughly with DMC and dried in vacuum at 110 °C overnight, and then reused for next cycle reaction. As displayed in Fig. 8, there was no remarkable change in the MPC conversion and yield DPC after three cycles over PbO/MgO and PbO/ZrO2. However, for PbO/SiO2, PbO/Al2O3 and PbO/TiO2 catalysts, the catalytic activities decreased gradually in the succeeding three runs, suggesting that PbO/MgO and PbO/ZrO2 possessed much better reusability than PbO/SiO2, PbO/Al2O3 and PbO/TiO2.
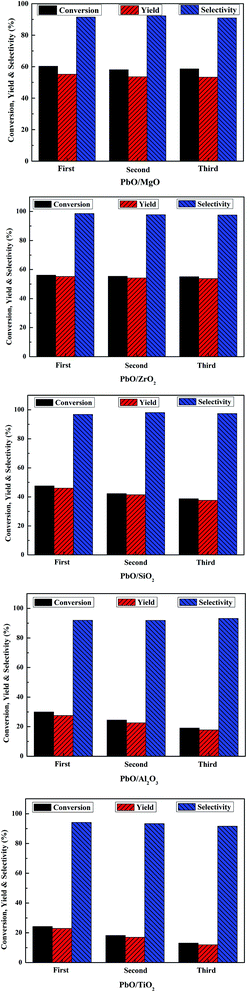 |
| Fig. 8 Reusability of the catalysts. Reaction conditions: MPC (150 mmol), catalyst (1.2 g), 200 °C, 2.5 h. | |
In heterogeneous catalysis, the leaching of active component was often the main reason which decreased the activity for further reuse. Therefore, the recovered catalysts were detected by ICP-OES analysis (Table S1†), and the content of Pb in the fresh and the third reused catalyst showed that Pb content had no significant loss over PbO/MgO and PbO/ZrO2, but dropped markedly over PbO/SiO2, PbO/Al2O3 and PbO/TiO2, respectively. Hence, the leaching loss of Pb might lead to the decrease of catalytic activities for the recovered PbO/SiO2, PbO/Al2O3 and PbO/TiO2 catalysts. Combined with the characteristic results of H2-TPR, the excellent recyclability of PbO/MgO and PbO/ZrO2 was possibly attributed to their strong interactions of the supports with highly dispersed Pb, which was potentially important for practical applications. Besides, the recovered catalysts after the third use were also detected by XRD characterization (Fig. S1†). The result indicated that the diffraction peaks of the third reused catalysts were basically identical to those of the fresh catalysts, suggesting that with the exception of PbO/Al2O3, PbO was still amorphous or microcrystalline states in the recovered catalysts. Therefore, MgO and ZrO2 might be suitable and promising support materials for the better preparation of Pb-based catalysts to transform MPC disproportionation to efficiently synthesize DPC in comparison with SiO2, Al2O3 and TiO2.
4. Conclusions
In summary, the support nature modified the structural properties of Pb catalysts and exerted marked influence on their catalytic performances. The catalysts were characterized by physicochemical techniques, and the results showed that PbO was dispersed better on SiO2, TiO2, ZrO2 and MgO than Al2O3, and the stronger metal-support interaction was obtained over MgO and ZrO2. The active results showed that the catalytic performances over MgO and ZrO2 to load Pb catalysts were significantly superior to those of SiO2, Al2O3 and TiO2, because of the higher active component dispersion and more Lewis acid active sites. Furthermore, the excellent recyclability in the case of PbO/MgO and PbO/ZrO2 was potentially important in industrial application. Thus, from this study it could be noted that the more Lewis acid site was present, the more the activity was, and the nature of the support was a key parameter for MPC disproportionation.
Conflicts of interest
There are no conflicts to declare.
Acknowledgements
The authors thank the financial support provided by the National High Technology Research and Development Program of China (863 program, No. 2013AA031703), National Natural Science Foundation of China (No. 21808048), Project funded by China Postdoctoral Science Foundation (2018M632782), the Key Project of Science and Technology Program of Henan Province (182102210050), the Science Research Start-up Fund of Henan Institute of Science and Technology (2015031) and the Project funded by Postdoctoral Science Foundation of Henan Province.
Notes and references
- M. C. Figueiredo, V. Trieu, S. Eiden, J. Heijl and M. T. M. Koper, ACS Catal., 2018, 8, 3087–3090 CrossRef CAS PubMed.
- S. Bahrami, S. N. Khorasani, A. Abdolmaleki, S. M. Davoodi and A. Farzan, J. Elastomers Plast., 2018, 50, 107–123 CrossRef CAS.
- G. Contreras-Zarazúa, J. A. Vázquez-Castillo, C. Ramírez-Márquez, G. A. Pontis, J. G. Segovia-Hernández and J. R. Alcántara-Ávila, Energy, 2017, 165, 637–649 CrossRef.
- A. Gawdzik, A. Mederska and T. Mederski, Polym. Bull., 2017, 74, 1553–1571 CrossRef CAS.
- R. Kaneg, H. Ogihar and I. Yamanaka, Catal. Sci. Technol., 2016, 6, 6002–6010 RSC.
- Y. Ono, Appl. Catal., A, 1997, 155, 133–166 CrossRef CAS.
- W. B. Kim, U. A. Joshi and J. S. Lee, Ind. Eng. Chem. Res., 2004, 43, 1897–1914 CrossRef CAS.
- M. Peng, X. Yang, J. X. Wu, H. Yuan, C. F. Yin and Y. X. Wu, Chem. Ind. Chem. Eng. Q., 2017, 23, 421–429 CrossRef CAS.
- G. Z. Fan, H. T. Zhao, Z. X. Duan, T. Fang, M. H. Wan and L. N. He, Catal. Sci. Technol., 2011, 1, 1138–1141 RSC.
- Y. Gao, Z. H. Li, K. M. Su and B. W. Cheng, Chem. Eng. J., 2016, 301, 12–18 CrossRef CAS.
- C. Z. Wang, T. Guo, M. M. Sun, C. H. Li, M. Guo, C. L. Li and Q. Wang, Sci. Adv. Mater., 2018, 10, 779–784 CrossRef CAS.
- S. L. Wang, R. Z. Tang, Y. Z. Zhang, T. Chen and G. Y. Wang, Chem. Eng. Sci., 2015, 138, 93–98 CrossRef CAS.
- S. H. Huang, B. Yan, S. P. Wang and X. B. Ma, Chem. Soc. Rev., 2015, 44, 3079–3116 RSC.
- Z. L Xiao, H. Yang, H. Zhang, T. Chen and G. Y. Wang, Chem. Pap., 2018, 72, 2347–2352 CrossRef.
- S. Han, M. Luo, X. L. Zhou, Z. He and L. P. Xiong, Ind. Eng. Chem. Res., 2012, 51, 5433–5437 CrossRef CAS.
- Y. T. Kim and E. D. Park, Appl. Catal., A, 2009, 356, 211–215 CrossRef CAS.
- W. Q. Zhou, X. Q. Zhao, Y. J. Wang and J. Y. Zhang, Appl. Catal., A, 2004, 260, 19–24 CrossRef CAS.
- Z. H. Fu and Y. Ono, J. Mol. Catal. A: Chem., 1997, 118, 293–299 CrossRef CAS.
- S. L. Wang, Y. Z. Zhang, Y. Chen, R. Z. Tang, T. Chen and G. Y. Wang, Chem. J. Chin. Univ., 2014, 35, 2177–2181 CAS.
- S. L. Wang, T. Chen, G. Y. Wang, C. X. Cui, H. Y. Niu and C. G. Li, Appl. Catal., A, 2017, 540, 1–6 CrossRef CAS.
- Z. Luo, Y. Wang, Y. Zhang, D. Wei, J. Jiang and G. Y. Wang, Fine Chem., 2008, 25, 813–816 CAS.
- S. L. Wang, Y. Z. Zhang, T. Chen and G. Y. Wang, J. Mol. Catal. A: Chem., 2015, 398, 248–254 CrossRef CAS.
- S. L. Wang, C. G. Li, Z. L. Xiao, T. Chen and G. Y. Wang, J. Mol. Catal. A: Chem., 2016, 420, 26–33 CrossRef CAS.
- Q. Fu, Y. C. Ou, Y. Zeng and G. Y. Wang, Chem. Ind. Eng. Prog., 2017, 36, 2748–2755 Search PubMed.
- S. L. Wang, H. Y. Niu, M. J. Guo, J. J. Wang, T. Chen and G. Y. Wang, Mol. Catal., 2019, 468, 117–124 CrossRef CAS.
- E. Leino, N. Kumar, P. Mäki-Arvela, A. R. Rautio, J. Dahl, J. Roine and J. P. Mikkola, Catal. Today, 2018, 306, 128–137 CrossRef CAS.
- C. José, M. V. Toledo, P. Nicolás, V. Lasalle, M. L. Ferreira and L. E. Briand, Catal. Sci. Technol., 2018, 8, 3513–3526 RSC.
- X. X. Wang, W. Chen, T. J. Lin, J. Li, F. Yu, Y. L. An, Y. Y. Dai, H. Wang, L. S. Zhong and Y. H. Sun, Chin. J. Catal., 2018, 39, 1869–1880 CrossRef CAS.
- J. S. Wang and H. Liu, RSC Adv., 2016, 6, 2374–2378 RSC.
- G. Tsilomelekis, A. Christodoulakis and S. Boghosian, Catal. Today, 2007, 127, 139–147 CrossRef CAS.
- K. V. R. Chary, B. R. Rao and V. S. Subrahmanyam, Appl. Catal., 1991, 74, 1–13 CrossRef CAS.
- L. Fratalocchi, C. G. Visconti, L. Lietti, G. Groppi, E. Tronconi, E. Roccaro and R. Zennaro, Catal. Sci. Technol., 2016, 6, 6431–6440 RSC.
- M. Jones, G. J. Hutchings, D. J. Willock, J. Scott and S. H. Taylor, J. Catal., 2018, 364, 102–111 CrossRef CAS.
- S. L. Wang, H. Y. Niu, J. J. Wang, T. Chen and G. Y. Wang, J. Alloys Compd., 2019, 777, 18–25 CrossRef CAS.
- L. Fratalocchi, C. G. Visconti, L. Lietti, N. Fischer and M. Claeys, Appl. Catal., A, 2018, 556, 92–103 CrossRef CAS.
- Y. H. Wu, W. Q. Xu, Y. Yang, J. Wang and T. Y. Zhu, Catal. Sci. Technol., 2018, 8, 297–306 RSC.
- B. Niu and Z. M. Xu, Green Chem., 2019, 21, 874–884 RSC.
- I. Prymak, O. Prymak, J. H. Wang, V. N. Kalevaru, A. Martin, U. Bentrup and S. Wohlrab, ChemCatChem, 2018, 10, 391–394 CrossRef CAS.
- L. H. Chagas, C. R. V. Matheus, P. C. Zonetti and L. G. Appel, Mol. Catal., 2018, 458, 272–279 CrossRef CAS.
- K. Wang, M. Dong, X. J. Niu, J. F. Li, Z. F. Qin, W. B. Fan and J. G. Wang, Catal. Sci. Technol., 2018, 8, 5646–5656 RSC.
- S. Pyen, E. Hong, M. Shin, Y. W. Suh and C. H. Shin, Mol. Catal., 2018, 448, 71–77 CrossRef CAS.
Footnote |
† Electronic supplementary information (ESI) available. See DOI: 10.1039/c9ra03931g |
|
This journal is © The Royal Society of Chemistry 2019 |