DOI:
10.1039/C9RA03847G
(Paper)
RSC Adv., 2019,
9, 19641-19647
Efficient solid-state emission and reversible mechanofluorochromism of a tetraphenylethene-pyrene-based β-diketonate boron complex†
Received
22nd May 2019
, Accepted 10th June 2019
First published on 24th June 2019
Abstract
A new twisted donor–acceptor (D–A) dye (BF2-TP) that was composed of tetraphenylethene and pyrene connected with a β-diketonate boron moiety has been synthesized and characterized. Such a dye showed unique intramolecular charge transfer (ICT) features, which were evidenced by spectral analysis and theoretical calculations. More importantly, BF2-TP solid samples exhibited an obvious mechanofluorochromic (MFC) behavior. Upon grinding with a spatula, the as-prepared powder sample illustrated a remarkable red shift of 62 nm, with considerable color contrast from yellow (562 nm) to orange red (624 nm). Its fluorescence color can be reversibly switched by repeating both the grinding–fuming and grinding–annealing processes. The mechanochromism is attributed to the phase transformation between amorphous and crystalline states. The results obtained would be helpful for designing novel MFC materials.
Introduction
Mechanofluorochromic (MFC) dyes refer to a kind of “smart” material that exhibits tunable and switchable solid state fluorescence in response to mechanical stimuli including pressing, grinding, crushing, or rubbing, and can be reverted to the original state by recrystallization, thermal treatment, or exposure to solvent vapors.1,2 Currently, these dyes have sparked tremendous interest owning to their unique properties and promising applications in the fields of optoelectronic devices,3 mechanical sensors,4 security ink,5 rewritable media,6 and fluorescent switches,7 and so on. MFC dyes convert the fluorescence color via changing their chemical structure or aggregate morphology.8 The former is a general method to alter the emission of a dye, but obtain limited success because of the irreversible and incomplete chemical reactions in the condensed phase. Most of the reported MFC dyes, the fluorescence color change are achieved through switch of their morphology under mechanical stimuli. These dyes can convert from crystal phase to amorphous state, or between two different crystalline phases upon modification of the intermolecular interactions, and produce a meta-stable state before and after the application of mechanical forces.8a,9 Generally, the dyes possess nonplanar π-conjugated structures and hence afford a looser molecular packing pattern in the crystal states, and can be easily damaged under external stimuli. As is well known, the derivatives of tetraphenylethene,6a,10 triphenylacrylonitrile,11 triphenylamine,12 and cyanoethylene,13 as well as some boron complexes14 are preferentially considered to act as MFC materials. Although the mechanisms for mechanochromism are somewhat clear, MFC dyes with extremely large MFC shifts (>60 nm) and obvious color contrast under simple mechanical force are still rare.15 Lots of MFC materials, showing the MFC shifts within tens of nanometers, have been synthesized.10a,14,16 MFC materials with bright solid-state emission and considerable color contrast are crucial for their usage in real world.17 It is very important to rationally design and synthesize such new dyes with excellent MFC performances.
As one class of typical fluorescent dyes, β-diketonate boron complexes display impressive optical properties including high fluorescence quantum yields in the solution and in the solid states, large molar extinction coefficients, electron mobilities, and high electron affinities.18,19 Therefore, they possess potential applications in laser dyes, imaging cells fluorophores, organic field effect transistors, and organic light emitting diodes (OLEDs).20–23 Recent studies show that some β-diketonate boron complexes exhibited excellent MFC behavior, and numerous derivatives belonging to this family have been described. For example, Nguyen and co-workers24 prepared a series of β-diketonate boron derivatives, and the photophysical properties of these dyes were reported to be related to the alkyl chains length. Gao et al.25,26 synthesized a series of β-diketonate boron complexes, the excellent photoluminescence properties in the solid state and sensitive to mechanical forces can generally be observed. Usually, the dyes with moderate intramolecular charge transfer (ICT) properties exhibit high MFC capability. Moreover, it is worth mentioning that the extended π-conjugated dyes constructed by two π-conjugated structures intersecting at a β-diketonate boron core have recently attracted significant interest since their rigid π-conjugated skeletons bring a range of desired attributes. This phenomenon compelled us to explore novel MFC dyes based on β-diketonate boron complexes. In addition, pyrene derivatives are considered to be excellent luminogens due to their polarity sensitive vibronic emission, high charge carrier mobility, long fluorescence lifetime, chemical stability and also possessing large planar surface through effective π–π stacking between the molecules.27 Herein, we designed and synthesized a novel D–A type MFC dye BF2-TP, which composed of two chromophores of a pyrene unit and a tetraphenylethene moiety (Scheme 1). The steric hindrance comes from the two bulky substituents attaching on the central β-diketonate boron core endows the compound with a high torsional molecular conformation, resulting in effective inhibition of close π-packing and enhancement of emission quantum yields in the solid states. Results demonstrated that BF2-TP gave strong emission in solution and in the solid state. In addition, BF2-TP exhibit reversible mechanofluorochromic behavior upon the treatment of grinding/fuming or thermal annealing, and the emission color changed between yellow and orange red with large MFC shifts (Δλmax = 62 nm), showing a remarkable mechanochromism. These results are important for the development of novel MFC dyes that respond to mechanical stimulation, and extending the viability of MFC function into organic fluorescent materials.
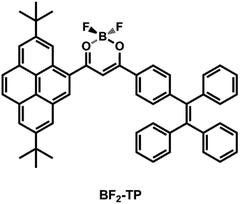 |
| Scheme 1 The molecular structure of BF2-TP. | |
Experimental
Materials and measurements
1H and 13C NMR spectra were recorded on a Bruker-Avance 400 MHz spectrometer by using CDCl3 as the solvents. Elemental analyses were performed with a PerkinElmer 240C elemental analyzer by investigation of C, H, and N. MS spectra were measured on Agilent Technologies 6224 spectrometer and MALDI-TOF MS Performance (Shimadzu, Japan). UV-visible spectra were carried out on a Shimadzu UV-2550 spectrophotometer. Fluorescence measurements were measured on a Cary Eclipse Fluorescence Spectrophotometer. The absolute fluorescence quantum yield for the solid sample was obtained using an Edinburgh FLS920 steady state spectrometer using an integrating sphere. Density functional theory (DFT) calculations were done employing the Gaussian 09W suit of programs at the B3LYP/6-31G(d) level. XRD patterns were measured on a Bruker D8 Focus Powder X-ray diffraction instrument.
Synthesis
Tetrahydrofuran (THF) was dried over sodium and distilled under nitrogen immediately prior to use. Dichloromethane (DCM) was distilled under normal pressure over sodium hydride under nitrogen before use. The other chemicals were used as received without further purification. Column chromatography was carried out on silica (200–300 mesh). Compounds 3 and 5 were synthesized according to the literatures.25,28
The synthetic routes for β-diketonate boron complex BF2-TP were shown in Scheme 2. Firstly, the tetraphenylethene derivative 3 was synthesized by Suzuki–Miyaura coupling between 1 and boric acid 2. Then the key intermediate 6 was obtained through the Friedel–Crafts alkylation reaction and the next Friedel–Crafts acyl reaction by using pyrene 4 as the starting material. Finally, the target molecules BF2-TP was prepared between 3 and 6 in the presence of sodium hydride in anhydrous THF followed by complexation with boron trifluoride diethyl etherate, to give a yield of 62%. BF2-TP has good solubility in common organic solvents such as THF, DCM, chloroform, and toluene, but show poor solubility in aliphatic hydrocarbon solvents (such as cyclohexane and n-hexane) and protic solvents (such as methanol and ethanol). The intermediates and the final products have been carefully purified and fully characterized by 1H and 13C NMR, MALDI-TOF mass spectrometry, and C, H, N elemental analyses.
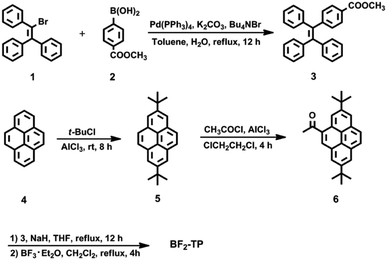 |
| Scheme 2 Synthetic routes of BF2-TP. | |
1-(2,7-Di-tert-butylpyren-4-yl)ethanone (6)
AlCl3 (12.2 g, 91.8 mmol) is added in 1,2-dichloroethane (100 mL) followed by the addition of acetyl chloride (7.2 g, 91.8 mmol) slowly over 30 min at 5 °C. Compound 5 (9.42 g, 30.0 mmol) in 1,2-dichloroethane (40 mL) was then added slowly, and the reaction mixture was stirred for 2 h at room temperature and kept for 2 h at 35 °C. Then the mixture was cooled to room temperature and the solvent was removed under reduced pressure. An orange solid appeared after dilute HCl (200 mL) was added to the flask. The solid is filtered and washed with water, followed by recrystallization from ethanol to give a white solid (9.63 g). Yield 90% mp 133.0–135.0 °C. 1H NMR (400 MHz, CDCl3) δ 9.25 (s, 1H), 8.61 (s, 1H), 8.30–8.25 (m, 3H), 8.08–8.01 (m, 2H), 2.96 (s, 3H), 1.61 (s, 18H) (Fig. S3†); 13C NMR (100 MHz, CDCl3) δ 201.93, 149.50, 149.06, 134.73, 132.37, 130.78, 128.89, 128.33, 127.12, 126.69, 124.53, 123.90, 123.66, 123.52, 122.93, 121.88, 35.50, 35.22, 31.94, 31.88, 29.92 (Fig. S4†); TOF LC/MS: m/z: calculated for C26H28O: 356.2140; found: 356.2132 (Fig. S5†). Anal. calcd. for C28H22O: C 87.60, H 7.92; found: C 87.71, H 7.83.
6-(2,7-Di-tert-butylpyren-4-yl)-2,2-difluoro-4-(4-(1,2,2-triphenyl-vinyl)phenyl)-2H-1,3,2-dioxaborinin-1-ium-2-uide (BF2-TP)
A mixture of 3 (2.00 g, 5.13 mmol) and 6 (1.66 g, 4.66 mmol) was dissolved in dry THF (80 mL), and then NaH (60%, 1.03 g, 25.75 mmol) was added. Under an atmosphere of nitrogen, the mixture was refluxed with stirring for 24 h. After the reaction was over, the mixture was cooled to room temperature. And then the mixture was acidified with dilute HCl and extracted with DCM. After solvent removal, the solid residue was dried under vacuum. The obtained solid was dissolved in dry DCM (80 mL), and boron trifluoride diethyl etherate (2.95 mL, 3.31 g, 23.30 mmol) was added to the solution. The mixture was stirred for 24 h under an atmosphere of nitrogen at room temperature. Then the mixture was poured into the water, and extracted with DCM. After solvent removal, the crude product was purified by column chromatography (silica gel, DCM/petroleum ether, v/v = 2/3), affording a yellowish green solid (2.31 g). Yield 65%. 1H NMR (400 MHz, CDCl3) δ 8.94 (s, 1H), 8.65 (s, 1H), 8.34 (d, J = 5.2 Hz, 2H), 8.29 (s, 1H), 8.10–8.04 (m, 2H), 7.98 (d, J = 8.4 Hz, 2H), 7.25 (d, J = 8.4 Hz, 3H), 7.18–7.15 (m, 9H), 7.06 (s, 6H), 1.60 (s, 9H), 1.59 (s, 9H) (Fig. S6†); 13C NMR (100 MHz, CDCl3) δ 186.69, 182.30, 151.96, 149.65, 149.50, 143.91, 142.88, 142.64, 139.50, 133.17, 132.20, 131.33, 131.23, 131.09, 129.65, 128.73, 128.54, 128.27, 128.05, 127.77, 127.33, 127.05, 126.62, 125.45, 124.34, 123.43, 121.09, 98.10, 35.54, 35.27, 31.84 (Fig. S8†). HRMS (MALDI-TOF) m/z: [M + H]+ calcd. for C53H46BF2O2: 763.3559; found: 763.7036 (Fig. S9†). Anal. calcd. for C53H45BF2O2: C 83.46, H 5.95; found: C 83.54, H 5.81.
Preparation of the samples for mechanofluorochromism study
The grinding powders were obtained by grinding the as-prepared powder with a pestle in the mortar. The fumed samples were prepared by fuming the grinding powder with DCM for 2 min. The annealing samples were obtained by heating the ground powders at 200 °C for 5 min.
Result and discussion
Absorption and fluorescence studies
Optical properties of the compound BF2-TP in solvents with different polarities was investigated by UV-vis and fluorescence spectroscopy. The UV-vis absorption and fluorescence emission spectra are demonstrated in Fig. 1, and the corresponding photophysical data are summarized in Table S1.† From the UV-vis absorption spectra (Fig. 1a), a relative strong absorption band at λ = 280–360 nm was attributed to π–π* local electron transitions of the conjugate system, which did not shift with increasing polarity of the deduced from the solvent-dependent absorption spectra. For example, in hexane, the CT band of BF2-TP centered at 411 nm and red-shifted gradually with increasing solvent polarity, its emission band reached 429 nm in DMSO. Form the fluorescence spectra (Fig. 1b), along with the increase of the polarity of the solvent, the emission bands of BF2-TP red-shifted rapidly and became broad. In the same time, the large Stokes shift is also observed. For example, BF2-TP emitted blue light appeared at 507 nm in hexane and green light located at 645 nm in DMSO with the band broadening, and was accompanying with large Stokes shift from 4548 cm−1 in hexane to 7806 cm−1 in DMSO. Combining the obvious transition to the low-energy direction, the significant Stokes shifts, and the extension of the emission bands indicated that ICT transitions of BF2-TP take place in polar solvents.29 It should be mentioned that the fluorescence spectra of BF2-TP exhibited vibrational structures in non-polar solvents, such as hexane and cyclohexane, which recommends two separated close-lying excited states. This phenomenon suggests that the locally excited (LE) state is responsible for the emission of BF2-TP in nonpolar solvents.30 The effects of solvents on the emission features can be further evaluated by the relationship between the emission maximum energy and a function of the Lippert solvent polarity (Lippert–Mataga). It illustrated the conformational change of the excited state surface prior to the emission.31 As shown in Fig. 1c, the slope can be used to assess the change in dipole moment upon excitation, and the break in the linear relationship suggests the existence of two different excited states. The deviation of the emission maximum energy in cyclohexane and hexane from the linear relationship followed by those in other solvents are in support of the fact that the emission of BF2-TP origins from the LE state in non-polar solvents, and from the ICT state in polar solvents.32 Moreover, the fluorescence quantum yield (Φf) of BF2-TP (Table S1†) showed a notable decrease with the increasing solvent polarity. The Φf value was from 0.369 to 0.083 when the solvent was changed from apolar (e.g., hexane) to highly polar (e.g., DMSO), further confirming unique ICT nature of compound BF2-TP.
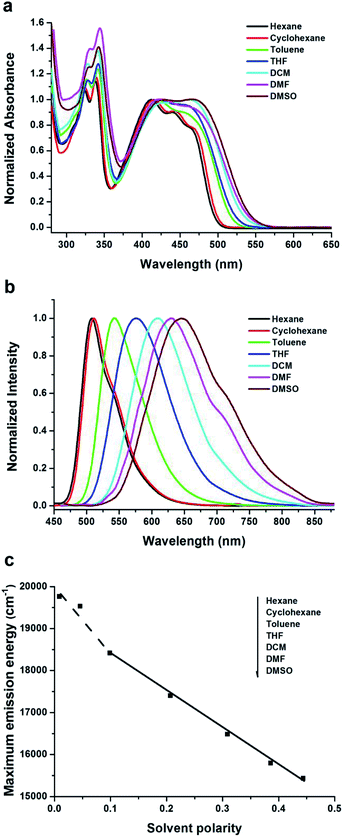 |
| Fig. 1 Normalized UV-vis absorption (a) and PL (b, λex = 420 nm) spectra of BF2-TP in different solvents (1.0 × 10−5 mol L−1). (c) Lippert–Mataga plot: fluorescence emission maximum energy of BF2-TP as a function of solvent polarity. | |
To gain a better insight into optical properties of BF2-TP, density functional theory (DFT) calculations was performed at the B3LYP/6-31G(d) level basis in the Gaussian 09W program package. As shown in Fig. 2, the HOMO is delocalized on the tert-butyl substituted pyrene fragment, while the LUMO is mainly distributed over the dioxaborine ring, as well as the tetraphenylethylene group. Thus, it can be inferred from the orbital distribution pattern that ICT occurs largely from the tert-butyl substituted pyrene unit to the dioxaborine ring regardless of whether tetraphenylethylene was present as a competing donor unit. In addition, the geometry optimized structures of BF2-TP demonstrate highly twisted spatial conformation. The dihedral angles between tert-butyl substituted pyrene (B) and the dioxaborine ring (C) is 43.7°, and the dihedral angles between the side benzene rings (A) in tetraphenylethene and the dioxaborine ring (C) is 16.9°. Such torsional angles in BF2-TP will prevent the molecules from packing in a close π–π stacking mode in the solid state and thus may endow it with MFC feature.
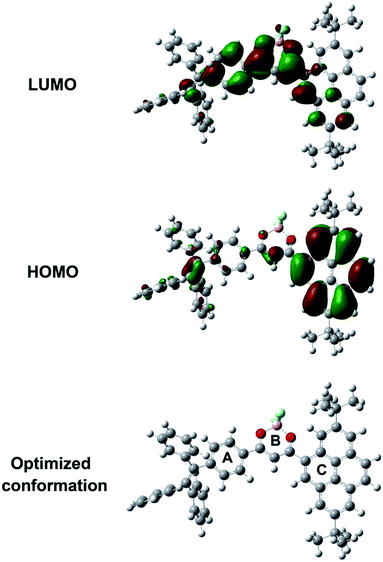 |
| Fig. 2 The frontier orbital contributions of BF2-TP calculated by DFT in Gaussian 09 at the B3LYP/6-31G(d) level. | |
Mechanofluorochromic properties
The crude product was purified on a silica-gel column to afford an orange powder. The BF2-TP powder demonstrated strong yellow emissions under 365 nm UV-light illumination, the quantum efficiencies of the as-prepared solid states was 0.46. Interestingly, after grinding with a mortar and pestle, the powder changed its emission color into orange red, demonstrating a property of mechanochromic fluorescence. After exposure to the vapor of DCM for 2 min, the emission color of the ground powder changed back to initial yellow and the as-prepared powder was obtained again. The mechanofluorochromic process is reproducible, which meant that the fluorescence transformation between yellow and orange red is reversible switched. As shown in Fig. S1,† the dye displayed excellent reversibility with almost no fatigue in response throughout the six cycles. The PL spectra measurement was applied to monitor such a reversible color switching under external stimuli. As depicted in Fig. 3a, the emission spectrum of BF2-TP is obvious with the initial emission centered at λmax 562 nm shifting to 624 nm after the mechanical grinding; that is, a spectral red-shift of 62 nm occurred simply by grinding. After fuming treatment, the emission peak of the ground solid blue-shifted to the initial wavelength. It is noted that the vapor fuming progress can be replaced by thermal annealing. When the ground sample of BF2-TP is annealed at 200 °C for 5 min, it could recover to the emission color of the original state corresponding as-prepared sample. At the same time, the recovery of the emission spectrum of BF2-TP could also be observed by heating its ground sample.
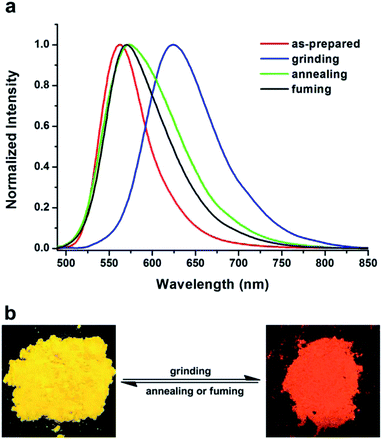 |
| Fig. 3 (a) Normalized fluorescent spectra of BF2-TP in different solid-states: as-synthesized, grinding and fuming, λex = 370 nm. (b) Photos of BF2-TP color changes under grinding and fuming stimuli. | |
The experiment of time-resolved fluorescence was carried out (Fig. S2a†) and the corresponding data are illustrated in Table S2.† The fluorescence decay curves were fitted by a double-exponential decay for BF2-TP in solid states, which revealed the mixture of two distinguished emission states in the amorphous phase. The fluorescent lifetime of ground sample and as-prepared sample were 7.57 ns and 3.61 ns, respectively. The longer lifetime of 7.57 ns indicted the formation of excimers after pressing.
Switchable mechanochromic dye of BF2-TP with a large shift of 62 nm prompts us to evaluate it as a kind of smart material with numerous potential applications. An example of such applications is demonstrated in Fig. 4, after being simply pressed by streaking a metal spatula on a piece filter paper with sprayed as-synthesized powder, an orange red letter appeared on the yellow background due to the amorphization of BF2-TP in the written “A” area under UV light illumination (Fig. 4b). Interestingly, after thermal annealing or vapor fuming the letter “A” can be merged in the background because of the crystallization of BF2-TP in area of “A” (Fig. 4c), and the clear orange red letter “B” can be written again (Fig. 4d). Such writing and erasing process can be repeated many times through repeating writing and fuming processes. The letters are invisible under room lighting but become visible with UV illumination. On the basis of its excellent MFC properties, BF2-TP may be utilized as optical recording materials.
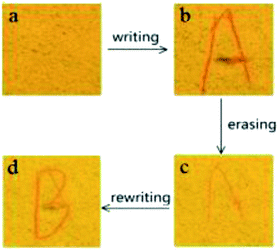 |
| Fig. 4 Photos of the luminescence writing/erasing process of BF2-TP on filter papers under 365 nm UV light illumination: (a) fluorescence emission of as-prepared powder; (b) the letter of “A” was written with a spatula; (c) the paper was erased by vapor fuming or thermal annealing; (d) rewritable the letter of “B” generated with a spatula. | |
With the aim of getting insight into the mechano-induced emission color changes, powder X-ray diffraction (XRD) was used to study the crystalline states (Fig. 5a). Many sharp and intense reflection peaks were observed in the diffraction pattern of the untreated sample, indicating that the as-prepared BF2-TP is well-ordered arrangement crystalline structure. In sharp contrast, all of the diffraction peaks displays diffuse and depressed reflections after grinding, verifying that the ground sample is amorphous. Furthermore, when fumed with DCM or annealed, sharp reflection peaks resemble to those of the as prepared powder emerge out, suggesting the ground sample can be readily converted back into an ordered crystalline lattice. Accordingly to these results, the mechanochromism of BF2-TP should be attributed to the crystalline–amorphous phase transformations, which greatly influences photophysical properties. Analysis by differential scanning calorimetry (DSC) also substantiates the above claim, and the results are presented in Fig. 5b. The as-prepared sample of BF2-TP demonstrated a strong endothermic peak at 362 °C, which corresponded to its melt point. After grinding, there are two cold-crystallization transition peaks at 167.0 °C and 195.0 °C before melting, indicating that a metastable aggregation structure in the ground sample is produced, and it would transfer to the more-stable state. After treatment by annealing at 200 °C for 5 min or solvent fuming by DCM for 2 min, the cold-crystallization transitions vanish and the shapes of the DSC curve is very similar to that obtain from the as-prepared sample. These results further confirm that the grinding treatment causes the transition in morphology of BF2-TP, meanwhile, the morphology transition can easily be recovered through fuming or annealing treatment.
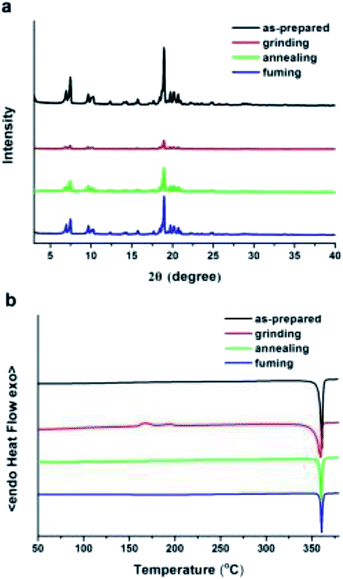 |
| Fig. 5 (a) XRD patterns of BF2-TP in different solid states: as-prepared, grinding, annealing and fuming. (b) DSC curves of BF2-TP in the ground and the crystalline states. | |
Conclusion
In this work, a novel D-A structured compound BF2-TP showing typical ICT characteristics has been successfully obtained and potential application of BF2-TP has also been investigated. The emission color of BF2-TP powders changed from initial yellow to final orange red under simple mechanical force, accompanied with the remarkable spectral shift of 62 nm. Moreover, the spectroscopic properties and morphological structures of the solid BF2-TP can be smartly switched in the grinding-heating or grinding–fuming fluorochromic process. The result indicates that the MFC behavior of BF2-TP is attributed to the reversible phase transformation between crystal and amorphous states. Thus, these intriguing properties enable BF2-TP for various potential applications in security inks, rewritable sensors, and light-emitting devices.
Conflicts of interest
There are no conflicts to declare.
Acknowledgements
We are grateful to the Program for Innovative Research Team of Science and Technology in the University of Henan Province (18IRTSTHN004), and Anyang Normal University Program (AYNU-2017-B16, AYNUKP-2018-B20) for financial support of this research.
Notes and references
-
(a) K. M. Wiggins, J. N. Brantley and C. W. Bielawski, Chem. Soc. Rev., 2013, 42, 7130–7147 RSC;
(b) Z. G. Chi, X. Q. Zhang, B. Q. Xu, X. Zhou, C. P. Ma and Y. Zhang,
et al.
, Chem. Soc. Rev., 2012, 41, 3878–3896 RSC;
(c) Y. Wang, M. J. Li, Y. M. Zhang, J. Yang, S. Y. Zhu and L. Sheng,
et al.
, Chem. Commun., 2013, 49, 6587–6589 RSC;
(d) X. Q. Zhang, Z. G. Chi, B. J. Xu, C. J. Chen, X. Zhou and Y. Zhang,
et al.
, J. Mater. Chem., 2012, 22, 18505–18513 RSC.
-
(a) F. Ciardelli, G. Ruggeri and A. Pucci, Chem. Soc. Rev., 2013, 42, 857–870 RSC;
(b) R. Misra, T. Jadhav, B. Dhokale and S. M. Mobin, Chem. Commun., 2014, 50, 9076–9078 RSC;
(c) N. Mizoshita, T. Tani and S. Inagaki, Adv. Mater., 2012, 24, 3350–3355 CrossRef CAS;
(d) H. Zhang, F. Gao, X. D. Cao, Y. Q. Li, Y. Z. Xu and W. G. Weng,
et al.
, Angew. Chem., Int. Ed., 2016, 55, 3040–3044 CrossRef CAS;
(e) C. P. Ma, B. J. Xu, G. Y. Xie, J. J. He, X. Zhou and B. Y. Peng,
et al.
, Chem. Commun., 2014, 50, 7374–7377 RSC;
(f) K. Ohno, S. Yamaguchi, A. Nagasawa and T. Fujihara, Dalton Trans., 2016, 45, 5492–5503 RSC;
(g) S. S. Zhao, L. Chen, L. Wang and Z. G. Xie, Chem. Commun., 2017, 53, 7048–7051 RSC;
(h) H. W. Wu, C. Hang, X. Li, L. Y. Yin, M. J. Zhu and J. Zhang,
et al.
, Chem. Commun., 2017, 53, 2661–2664 RSC.
-
(a) C. L. Li, S. P. Wang, W. P. Chen, J. B. Wei, G. C. Yang and K. Q. Ye,
et al.
, Chem. Commun., 2015, 51, 10632–10635 RSC;
(b) C. L. Li, J. B. Wei, J. X. Han, Z. Q. Li, X. X. Song and Z. L. Zhang,
et al.
, J. Mater. Chem. C, 2016, 4, 10120–10129 RSC.
-
(a) A. Facchetti, Chem. Mater., 2010, 23, 733–758 CrossRef;
(b) J. Mei, J. Wang, J. Z. Sun, H. Zhao, W. Z. Yuan and C. M. Deng,
et al.
, Chem. Sci., 2012, 3, 549–558 RSC;
(c) S. Pagidi and P. Thilagar, J. Mater. Chem. C, 2017, 5, 6537–6546 RSC.
-
(a) S. Sasaki, G. P. C. Drummen and G. Konishi, J. Mater. Chem. C, 2016, 4, 2731–2743 RSC;
(b) Y. Yuan, W. Yuan and Y. Chen, Sci. China Mater., 2016, 59, 507–520 CrossRef CAS.
-
(a) Q. Y. Lu, X. F. Li, J. Li, Z. Y. Yang, B. J. Xu and Z. G. Chi,
et al.
, J. Mater. Chem. C, 2015, 3, 1225–1234 RSC;
(b) X. L. Luo, J. N. Li, C. H. Li, L. P. Heng, Y. Q. Dong and Z. P. Liu,
et al.
, Adv. Mater., 2011, 23, 3261–3265 CrossRef CAS;
(c) C. Li, X. Luo, W. Zhao, Z. Huang, Z. Liu and B. Tong,
et al.
, Sci. China: Chem., 2013, 56, 1173–1177 CrossRef CAS.
-
(a) Z. Ma, M. Teng, Z. Wang, S. Yang and X. Jia, Angew. Chem., Int. Ed., 2013, 52, 12268–12272 CrossRef CAS;
(b) X. Cheng, D. Li, Z. Zhang, H. Zhang and Y. Wang, Org. Lett., 2014, 16, 880–883 CrossRef CAS;
(c) Y. Shi, Y. Cai, Y. J. Wang, M. Chen, H. Nie and W. Qin,
et al.
, Sci. China: Chem., 2017, 60, 635–641 CrossRef CAS.
-
(a) Y. Sagara, T. Mutai, I. Yoshikawa and K. Araki, J. Am. Chem. Soc., 2007, 129, 1520–1521 CrossRef CAS;
(b) D. A. Davis, A. Hamilton, J. L. Yang, L. D. Cremar, G. D. Van and S. L. Potisek,
et al.
, Nature, 2009, 459, 68–72 CrossRef CAS;
(c) K. Wang, H. Zhang, S. Chen, G. Yang, J. Zhang and W. Tian,
et al.
, Adv. Mater., 2014, 26, 6168–6173 CrossRef CAS;
(d) K. Ariga, T. Mori and J. P. Hill, Adv. Mater., 2012, 24, 158–176 CrossRef CAS;
(e) S. J. Yoon, J. W. Chung, J. Gierschner, K. S. Kim, M. G. Choi and D. Kim,
et al.
, J. Am. Chem. Soc., 2010, 132, 13675–13683 CrossRef CAS.
-
(a) Y. Ooyama, Y. Kagawa, H. Fukuoka, G. Ito and Y. Harima, Eur. J. Org. Chem., 2009, 2009, 5321–5326 CrossRef;
(b) S. J. Yoon and S. Park, J. Mater. Chem., 2011, 21, 8338–8346 RSC.
-
(a) X. Y. Shen, Y. J. Wang, E. Zhao, W. Z. Yuan, Y. Liu and P. Lu,
et al.
, J. Phys. Chem. C, 2013, 117, 7334–7347 CrossRef CAS;
(b) J. H. Jia, P. C. Xue and R. Lu, Tetrahedron Lett., 2016, 57, 2544–2548 CrossRef CAS;
(c) J. Shi, W. Zhao, C. Li, Z. Liu, Z. Bo and Y. Dong,
et al.
, Chin. Sci. Bull., 2013, 58, 2723–2727 CrossRef CAS;
(d) M. P. Aldred, G. F. Zhang, C. Li, G. Chen, T. Chen and M. Q. Zhu, J. Mater. Chem. C, 2013, 1, 6709–6718 RSC;
(e) H. Gao, D. Xu, X. Liu, A. Han, L. Zhou and C. zhang,
et al.
, RSC Adv., 2017, 7, 1348–1356 RSC.
-
(a) Y. Gong, Y. Tan, J. Liu, P. Lu, C. Feng and W. Z. Yuan,
et al.
, Chem. Commun., 2013, 49, 4009–4011 RSC;
(b) W. Z. Yuan, Y. Q. Tan, Y. Y. Gong, P. Lu, J. W. Y. Lam and X. Y. Shen,
et al.
, Adv. Mater., 2013, 25, 2837–2843 CrossRef CAS.
-
(a) L. Zhou, D. Xu, H. Gao, A. Han, X. Liu and C. Zhang,
et al.
, Dyes Pigm., 2017, 137, 200–207 CrossRef CAS;
(b) Q. Q. Fang, Q. Wang, W. Lin, X. Yu, Y. Q. Dong and Y. W. Chi, Sens. Actuators, B, 2017, 244, 771–776 CrossRef CAS.
- J. Zhao, Z. Chi, Y. Zhang, Z. Mao, Z. Yang and E. Ubba,
et al.
, J. Mater. Chem. C, 2018, 6, 6327–6353 RSC.
- J. Zhao, J. Peng, P. Chen, H. Wang, P. Xue and R. Lu, Dyes Pigm., 2018, 149, 276–283 CrossRef CAS.
-
(a) A. Balch, Angew. Chem., Int. Ed., 2009, 48, 2641–2644 CrossRef CAS PubMed;
(b) Y. J. Dong, B. Xu, J. B. Zhang, X. Tan, L. J. Wang and J. L. Chen,
et al.
, Angew. Chem., Int. Ed., 2012, 51, 10782–10785 CrossRef CAS;
(c) Y. Zhang, K. Wang, G. Zhuang, Z. Xie, C. Zhang and F. Cao,
et al.
, Chem.–Eur. J., 2015, 21, 2474–2479 CrossRef CAS;
(d) W. Liu, S. Ying, Q. Sun, X. Qiu, H. Zhang and S. Xue,
et al.
, Dyes Pigm., 2016, 125, 8–14 CrossRef CAS.
-
(a) Y. Zhao, H. Lin, M. Chen and D. Yan, Ind. Eng. Chem. Res., 2014, 53, 3140–3147 CrossRef CAS;
(b) Y. Li, Z. Zhuang, G. Lin, Z. Wang, P. Shen and Y. Xiong,
et al.
, New J. Chem., 2018, 42, 4089–4094 RSC;
(c) M. Chen, R. Chen, Y. Shi, J. Wang, Y. Cheng and Y. Li,
et al.
, Adv. Funct. Mater., 2018, 28, 1704689 CrossRef;
(d) C. Lin, Y. Liu, S. Peng, T. Shinmyozu and J. Yang, Inorg. Chem., 2017, 56, 4978–4989 CrossRef CAS.
-
(a) T. Han, X. Feng, D. Chen and Y. Dong, J. Mater. Chem. C, 2015, 3, 7446–7454 RSC;
(b) Y. Q. Dong, J. W. Lam and B. Z. Tang, J. Phys. Chem. Lett., 2015, 6, 3429–3436 CrossRef CAS;
(c) X. Hou, C. Ke, C. J. Bruns, P. R. McGonigal, R. B. Pettman and J. F. Stoddart, Nat. Commun., 2015, 6, 6884 CrossRef;
(d) F. Xu, T. Nishida, K. Shinohara, L. Peng, M. Takezaki and T. Kamada,
et al.
, Organometallics, 2017, 36, 556–563 CrossRef CAS.
- T. D. Liu, A. D. Chien, J. W. Lu, G. Q. Zhang and C. L. Fraser, J. Mater. Chem., 2011, 21, 8401–8408 RSC.
- G. Zhang, J. Lu, M. Sabat and C. L. Fraser, J. Am. Chem. Soc., 2010, 132, 2160–2162 CrossRef CAS.
-
(a) H. Maeda, Y. Mihashi and Y. Haketa, Org. Lett., 2008, 10, 3179–3182 CrossRef CAS;
(b) K. Ono, K. Yoshikawa, Y. Tsuji, H. Yamaguchi, R. Uozumi and M. Tomura,
et al.
, Tetrahedron, 2007, 63, 9354–9358 CrossRef CAS;
(c) E. Cogne-Laage, J. F. Allemand, O. Ruel, J. B. Baudin, V. Croquette and M. Blanchard-Desce,
et al.
, Chem.–Eur. J., 2004, 10, 1445–1455 CrossRef CAS.
- G. Zhang, J. Chen, S. J. Payne, S. E. Kooi, J. N. Demas and C. L. Fraser, J. Am. Chem. Soc., 2007, 129, 8942–8943 CrossRef CAS.
-
(a) A. Nagai, K. Kokado, Y. Nagata, M. Arita and Y. Chujo, J. Org. Chem., 2008, 73, 8605–8607 CrossRef CAS;
(b) A. Nagai, K. Kokado, Y. Nagata and Y. Chujo, Macromolecules, 2008, 41, 8295–8298 CrossRef CAS.
-
(a) J. Fabian and H. Hartmann, J. Phys. Org. Chem., 2004, 17, 359–369 CrossRef CAS;
(b) B. Domercq, C. Grasso, J. L. Maldonado, M. Halik, S. Barlow and S. R. Marder,
et al.
, J. Phys. Chem. B, 2004, 108, 8647–8651 CrossRef CAS;
(c) Y. Sun, D. Rohde, Y. Liu, L. Wan, Y. Wang and W. Wu,
et al.
, J. Mater. Chem., 2006, 16, 4499–4503 RSC.
- N. D. Nguyen, G. Q. Zhang, J. W. Liu, A. E. Sherman and C. L. Fraser, J. Mater. Chem., 2011, 21, 8409–8415 RSC.
- H. Gao, D. Xu, X. Liu and A. Han, Dyes Pigm., 2017, 139, 157–165 CrossRef CAS.
- H. Gao, D. Xu, Y. Wang, Y. Wang, X. Liu and A. Han,
et al.
, Dyes Pigm., 2018, 150, 59–66 CrossRef CAS.
-
(a) A. Kathiravan, K. Sundaravel, M. Jaccob, G. Dhinagaran, A. Rameshkumar and D. Arul Ananth,
et al.
, J. Phys. Chem. B, 2014, 118, 13573–13581 CrossRef CAS PubMed;
(b) M. Shellaiah, Y. H. Wu, A. Singh, M. V. R. Raju and H. C. Lin, J. Mater. Chem. A, 2013, 1, 1310–1318 RSC.
- Z. Wu, Z. Huang, R. Guo, C. Sun, L. Chen and B. Sun,
et al.
, Angew. Chem., Int. Ed., 2017, 56, 13031–13035 CrossRef CAS.
- J. S. Yang, K. L. Liau, C. M. Wang and C. Y. Hwang, J. Am. Chem. Soc., 2004, 126, 12325–12335 CrossRef CAS.
- G. Jones II, W. R. Jackson, C. Choi and W. R. Bergmark, J. Phys. Chem., 1985, 89, 294–300 CrossRef.
- C. Reichardt, Chem. Rev., 1994, 94, 2319–5238 CrossRef CAS.
- F. Loiseau, S. Campagna, A. Hameurlaine and W. Dehaen, J. Am. Chem. Soc., 2005, 127, 11352–11363 CrossRef CAS.
Footnote |
† Electronic supplementary information (ESI) available. See DOI: 10.1039/c9ra03847g |
|
This journal is © The Royal Society of Chemistry 2019 |