DOI:
10.1039/C9RA03605A
(Review Article)
RSC Adv., 2019,
9, 19748-19761
Factors affecting the efficiency of a bioelectrochemical system: a review
Received
14th May 2019
, Accepted 11th June 2019
First published on 25th June 2019
Abstract
The great potential of bioelectrochemical systems (BESs) in pollution control combined with energy recovery has attracted increasing attention. Classified by their functions in the BES, microorganisms including degraders, electricigens, and element cycle-related microbes play key roles in pollutant degradation and electricity generation, and the functions of these microbes are affected by various environmental and operating conditions. This review systematically summarizes the effects of crucial conditions on the efficiency of the process of contaminant removal combined with electricity generation in BESs, with particular focus on the pH, temperature, conductivity, substrates, inoculums, magnetic field and reactor design parameters, such as architecture, electrode material, and electrode potential. The aim of this review is to help reveal the microbial functions during the bioelectrochemical remediation of environmental media and to optimize the system by determining the appropriate conditions for functional microorganisms, thus better promoting the transition of BESs from the laboratory to actual applications.
1. Introduction
A bioelectrochemical system (BES) is a tool that converts chemical energy directly into a valuable resource, such as hydrogen in microbial electrolysis cells (MECs) and electricity in microbial fuel cells (MFCs), by means of microbial catalysis.1–3 Presently, BESs show great potential for the removal of pollutants from a variety of environments, such as wastewater,4–6 contaminated sediments7,8 and soils.9–12
BESs can be fed any biodegradable organic matter, from simple molecules (such as carbohydrates or proteins) to complex mixtures (such as petroleum hydrocarbons or swine wastewater), that can be effectively degraded by microorganisms in the system, resulting in the output of electrical energy or hydrogen.13,14 Consequently, BESs reduce energy loss and waste generation,15 which greatly reduces costs compared to physical and chemical remediation techniques. Furthermore, based on the inexhaustible electron acceptor of the solid anode, the enhancement ability of the biocurrent is sustained, which overcomes the deficiency of the electron acceptor in a contaminated medium.16,17 However, there are still some problems to be solved in the actual application of BESs, such as improving the degradation of complex compounds, controlling the reaction process of microorganisms, and continuing to reduce costs.
Recently, researchers have made extensive efforts to overcome some deficiencies, such as improving the reactor configuration and electrode materials,18,19 finding efficient electrogenic bacteria,20 and achieving multi-factor enhancement of BES.21–24 In BESs, microorganisms play a key role in the degradation of pollutants and the generation of electricity or hydrogen,25–27 so the intricate interactions of functional microbes have been thoroughly studied. However, the activity and number of functional microorganisms are directly influenced by the surrounding conditions.
In this review, factors presently affecting the performance of BESs are summarized, e.g., pH, temperature and conductivity. The understanding of relationships between the reinforcement measures and performance of a BES aims to assist in revealing the biological mechanism and providing the guidance for BES application.
2. The reaction spontaneity in BESs
BES generally consists of an anode chamber and a cathode chamber, and both electrodes are connected by an external circuit. BES uses electrochemically active microorganisms as the catalysts in the anode to oxidize contaminants and transfer electrons to the cathode through the external circuit. Microbial anodes perform a core function in a BES: they oxidize various resistant organic compounds, including polycyclic aromatic hydrocarbons (PAHs), phenols, and polychlorinated biphenyls (PCBs), that cannot be degraded easily under natural conditions.7,11,28,29 The system is constructed either for electricity generation via spontaneous redox reactions (in MFCs) or applying external electrical power to initiate an otherwise non-spontaneous reaction to produce value-added products, such as hydrogen reduced from protons (in MECs) or methane reduced from carbon dioxide (via microbial electrosynthesis).1–3,25 Theoretically, the potential of the redox reaction occurring on the surface of the electrodes determines the potential of the electrodes and the spontaneity of the reaction. When the potential of the cathode where the reduction reaction occurs is higher than the potential of the anode where the oxidation reaction occurs, the corresponding Gibbs free energy is less than 0, so the reaction can be spontaneous, and the system can output electric energy to the outside (MFCs). Otherwise, the system needs additional energy to trigger the reaction (MECs).30,31
3. Diverse functions in BESs
A BES is a complex with various biodegradation processes of substrates and many types of redox systems, such as the nitrogen cycle, sulfur cycle, and iron cycle; thus, the participating microorganisms are also diverse. Based on their roles in the system, the bacteria can be divided mainly into electrogenic bacteria, degrading bacteria, and element cycle-related bacteria, each of which serve integral functions. In the anodic region, the degrading/fermentative microorganisms, which are primarily bacteria or fungi, break pollutants down into an assimilable carbon source for the growth of electrogenic microorganisms and element cycle-related microorganisms, such as the nitrogen transformation microorganisms, which are usually archaea and are capable of providing the available nitrogen source. Consequently, a metabolic network of a bacteria-fungi-archaea consortium is established to perform specific diverse functions, e.g., electricity generation and pollutant degradation32,33 (Fig. 1). Notably, a bacterium may play different roles in the BES. For example, the electrogenic bacteria (e.g., Geobacter) or related bacteria (e.g., sulfate reducers) can also degrade the contaminants and transfer electrons to the anode in some cases.34
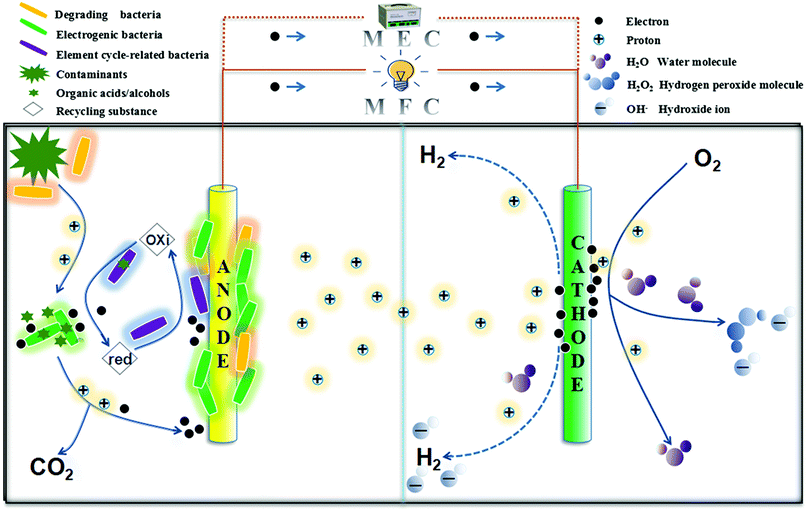 |
| Fig. 1 Overview of anodic and cathodic reactions in a bioelectrochemical system (BES). Degrading bacteria, electrogenic bacteria, and element cycle-related bacteria are integrated into the anode chamber. The main chemical process occurs in the cathode chamber, and the dashed line indicates that it occurs under the conditions of an external power supply. | |
In the process of bioelectrochemical treatment, there are many substances acting as electron acceptors besides the anode, such as sulfate, nitrate, and Fe(III).16,35 They capture electrons during the substrate degradation process and participate in the cycles of nitrogen, sulfur, iron, etc., with the assistance of related bacteria. Studies have shown that microorganisms can oxidize organic pollutants to carbon dioxide in anaerobic conditions with the reduction of sulfate, nitrate, or Fe(III),36–38 and the addition of these electron acceptors can promote the metabolic activity of related microorganisms. It was found the enrichment of Desulfobulbaceae and Desulfobacteraceae, which belong to traditional sulfate-reducing bacteria (SRB), during the degradation of toluene in a BES.39 Sulfate is reduced to sulfide by SRB, and sulphide can be oxidized to elemental sulfur by a biological process or a chemical process on the anodic surface; the sulfur can then be reduced to sulfide again, which can be re-oxidized to elemental sulfur, thus constituting a sulfur cycle that promotes current generation.39–41 Moreover, in nitrogen-containing BESs, denitrifying bacteria can use organic pollutants, such as carbon and energy sources, for their own growth and use nitrate as an electron acceptor to degrade organic pollutants under anaerobic conditions.42 Additionally, the presence of iron oxides in a BES can lead to the enrichment of iron-reducing bacteria, some of which can oxidize some electron donors effectively to promote the degradation of the substrate.43 There is a potential that the presence of external electron acceptors could stimulate the growth of related microorganisms, which could use organic pollutants energetically. Consequently, the elemental cycles involved with various functional bacteria affect the electron transfer process and change the physicochemical properties of the environmental media, thereby affecting the pollutant degradation efficiency and the energy output of the BES.
4. Factors affecting the efficiency of BESs
The functional microorganisms in a BES are a succession of indigenous microbes, and they are therefore affected by the initial environmental properties, such as pH, temperature, moisture content, oxygen level, conductivity, nutrient ions, growth factors, types and concentrations of contaminants.10,44 On this basis, the system is subjected to bioelectrochemical treatment and various enhancement measures, commonly including adjustment of pH, temperature or moisture, magnetic field acclimation of anode microorganisms, anaerobic treatment in the anode, aeration in the cathode, the addition of external nutrient sources (glucose, vitamins, inorganic salt ions, etc.) or co-metabolic substrates (acetate, pyruvate, glucose, sucrose, etc.), changes in the conductivity of the environmental media or electron transfer activity (doping with activated carbon, carbon fibre, gravel, salt, external electron mediators, etc.), current enhancement by changing the external resistance, or setting of the potential via a potentiostat. These measures could change the properties of the environmental media and thereby the microbial activity, species and structure that control the removal of contaminants and the production of electricity in the BES.
4.1 pH
During the operation of the BES, the organic matter near the anode is oxidized to produce protons and electrons. The electrons are transferred to the cathode through an external circuit, and the protons migrate to the cathode in the environmental medium and need to pass a proton exchange membrane (PEM) or salt bridge in a dual-chamber system. At the same time, the cathodic reaction consumes protons and electrons, which combine with oxygen to form water, for example in an air-cathode.45 However, the environmental media, especially soil or sediments, and the PEM or salt bridges have a huge internal resistance that hinders the transmission of protons, resulting in a proton transfer rate much lower than the anodic output rate and the cathodic consumption rate, thus producing a pH gradient from anode to cathode.46,47 For example, proton accumulation occurred close to the anode, leading to the formation of an acid–alkaline transition zone from the anode to the cathode in a bioelectrochemical remediation system.11 A suitable pH range is required for the survival of microorganisms, so the acid–base differentiation between the anode and cathode adversely affects the growth and activity of microorganisms.48 Microbial activities are greatly suppressed if the pH is outside the appropriate range. For example, a variation in pH can cause changes in the charge carried by biological macromolecules, such as proteins and nucleic acids, affecting their biological activity, or the electrical charge of cell membranes, disturbing the ability of microbial cells to absorb nutrients.49 Microorganisms in a BES adapt to different pH conditions, and it is of great significance to identify the pH values that are simultaneously suitable for both electrogenic microorganisms and degrading microorganisms.
During anode synthesis with wastewater maintained at pH 7.5, 7.0, 6.5, 6.0, and 5.5 with 50 mM phosphate buffer for 5 days each and an aerated 50 mM phosphate buffer with a pH of 7.0 as the catholyte, the highest chemical oxygen demand (COD) removal and coulombic efficiency were observed at pH 6.5 (ref. 50) (Table 1). Therefore, the pH of the anolyte is an important parameter of BESs and directly controls the electron and proton generation, thus affecting the metabolic activity of the substrate-specific microbes. Under high pH conditions, protons tend to be reduced, which is not conducive to the generation and transmission of electrons, and a higher pH (>9) favours the growth of methanogens.51,52 In contrast, a slightly lower pH (6.2–7.5) can inhibit the production of methane, but the lower pH (5.5) is unfavourable to the hydrolysis and carboxylation of organic substances and destroys the dynamic balance of the degradation reaction.53,54 Additionally, the pH affects the internal resistance of the BES mainly by altering the form of ions, such as heavy metals, in the soil system.55 The acidification of the anode enhances the desorption of heavy metals on the surface of the soil particles and reduces the internal resistance of the soil BES, while the alkalization of the cathode causes precipitation of cations and lowers the electrical conductivity, thus increasing the internal resistance. Moreover, the difference in the pH between the cathode and the anode also affects the internal resistance of the BES. For example, a higher pH difference could increase the proton flux rate through the PEM and reduce the internal resistance of the system.50 In addition to the above effects, pH also exerts an influence on the elemental circulation in the system, such as the nitrogen and sulfur cycles. For example, pH can regulate the denitrification and sulfate reduction process of microorganisms, thus changing the direction and speed of electron transfer.56
Table 1 Electrochemical performances of BESs under pH differences between anode and cathode
Medium |
Anode pH |
Cathode pH |
pH difference |
Energy production |
Ref. |
Wastewater |
5.4 |
9.5 |
4.1 |
0.55 mA/3 mm3 |
46 |
6.8 |
7.3 |
0.5 |
1.35 mA/3 mm3 |
Wastewater |
5.5 |
7.5 |
2 |
129.40 mW m−2 |
54 |
5.5 |
7.5 |
2 |
142.41 mW m−2 |
Wastewater |
7.5 |
7 |
−0.5 |
0.56 mA |
50 |
7 |
7 |
0 |
0.73 mA |
6.5 |
7 |
0.5 |
1.1 mA |
6 |
7 |
1 |
0.72 mA |
5.5 |
7 |
1.5 |
0.53 mA |
Nutrient solution with acetate |
7.2 |
7.1 |
−0.1 |
22 000 mW m−3 |
56 |
PBS with glucose |
7 |
7 |
0 |
1720 mW m−2 |
123 |
Soil polluted by petroleum |
— |
— |
0.1–0.2/cm anode soil |
0.85 mW m−2 |
11 |
Wastewater polluted by petroleum |
7 |
7 |
0 |
0.72 mW m−2 |
64 |
Modified SL3 media |
11 |
7 |
−4 |
1.6 mW m−2 |
80 |
Pb contaminated soil |
5.5 |
9.5 |
4 |
3.6 mW cm−2 |
55 |
Cd contaminated soil |
4.8 |
8.5 |
3.7 |
7.5 mW cm−2 |
Therefore, the acid–base differentiation between electrodes is a vital limiting factor for the performance of BESs through its effect on the activity of microorganisms and the bioavailability of substrates. To solve this problem, we can add alkaline substances to the anode, acidic substances to the cathode after the acid–base differentiation of the electrodes, or add a buffer solution to increase the buffer capacity of the system.45,56 Additionally, enhancing the mass transfer capacity of the system is an important means to alleviate this problem. For example, we can add large-diameter particles and/or silica colloids to the soil system to increase porosity and promote mass transfer or shorten the distance between electrodes to reduce mass transfer resistance.11,21,57 Moreover, BESs operated for different purposes utilize different dominant microorganisms, so the optimal pH conditions resulting in the best performance of the system also vary. For example, the anode pH, which is alkaline (pH = 9), is the most favourable for the functional process of hydrogenogens in BESs for hydrogen production.52 The pH range selected for BESs for electricity production is generally neutral, which optimizes the activity of electrogenic bacteria. For example, pH = 6.80 is optimal for the traditional model electrogen Geobacter sulfurreducens.58 BESs mainly used for biodegradation should also take into account the effect of pH on the bioavailability of the substrates. For example, pH = 6.0–7.0 is the most favourable for the degradation of alkanes by Alcanivorax in BESs degrading petroleum hydrocarbons,59 and an alkaline anode pH (pH = 8.6) could be maintained in BESs operated for the denitrification of wastewater streams.60
4.2 Temperature
Temperature is an essential condition for the growth of microorganisms, and changes in temperature affect the microbial growth rate, activity and distribution in BESs.32,61 For most microorganisms, 35–40 °C is the optimum temperature range for growth, enzyme activity, and formation of a stable biofilm, thereby increasing the substrate degradation rate and the electricity production in the BES.29,32,62,63 In many studies, increasing the temperature will increase the power output of the BES. It was demonstrated that the Arrhenius law is followed between anode current output and temperature in the range of 30–45 °C.63 However, when the temperature reaches a certain level, the activity and structure of the microbial enzyme are impaired, thereby reducing the performance of the BES. A study investigated the effect of temperature on the anaerobic biodegradation of petroleum hydrocarbons in an MFC and showed that 40 °C was the optimum operating temperature and that the degradation rate and maximum power density were twice as high at 40 °C than at 30 °C, whereas the degradation rate at 50 °C was reduced to a quarter of that at 40 °C.64
The effect of temperature on the BES involves many kinetic and thermodynamic principles due to the occurrence of complex reactions, and the temperature is not directly proportional to the electricity production or the efficiency of degradation in a BES. For example, although high temperatures can increase the microbial reaction kinetics, mass transfer efficiency, and thermodynamic activity of the system, high temperatures also promote the growth and reproduction of non-electrogenic microorganisms, such as fermentation bacteria and methanogens. These other microorganisms compete with electrogenic microorganisms, thus decreasing the current density and coulombic efficiency with an increase in COD removal efficiency.50,65 In contrast, low temperature conditions inhibit the growth of methanogenic bacteria and help generate hydrogen.32,64 In a study of the effect of temperature fluctuations on MEC performance and methanogenic microorganisms, the number of methanogens decreased by 68–91% at 4–9 °C, and when the temperature returned to 30 °C, the quantity of methanogens and methane production returned to the original level.32 Temperature changes have no obvious effect on the major predominantly electricity-producing bacterium Geobacter, and this insensitivity to temperature is attributed to its wide adaptive temperature range.32,66 Based on the different sensitivities of various microorganisms to temperature, selecting suitable temperature conditions can result in satisfactory BES performance. In mixed inoculated BESs for biodegradation, high temperatures can enhance the utilization rate of the substrate by increasing various microbial activities, and 40 °C is the optimal choice.64 However, to increase the efficiency of energy production in reactors, it is beneficial to operate at a lower temperature where the activity of methanogens is inhibited.32 In practice, insulating materials (such as mineral wool and foam) and solar energy can be used to control the temperature. For example, during sewage treatment, solar energy can be used to heat wastewater to promote microbial growth, and insulating material can be used to reduce heat diffusion.67
BESs experience constant temperature changes between day and night in field applications, which requires the BESs to have a certain degree of endurance so that they can still run stably within a certain temperature range.68 Studies have shown that different starting temperatures have a great influence on the long-term performance of a BES. It was found that a BES started at a high temperature had a higher electric stability than one started at a low temperature, indicating that lower temperatures may delay or prevent the adequate start-up of the system.69 Consequently, it may be desirable to heat the wastewater (e.g., to 40 °C) during the establishment of the anode biofilm.29 Considering the adverse effects of high temperatures on the system's energy production, we could start the system at a high temperature and then operate at a relatively low temperature. In addition to the direct influence of temperature on microorganisms in the BES, there are also effects on the physical and chemical properties of the treatment media (water, sediment, soil, etc.), ion membranes, electrodes, etc., causing difficulties in the study of the single influence of temperature on a certain factor.70 For example, a high temperature could enhance the mobility of water molecules and soluble ions, thereby reducing the resistivity of the system.71 Additionally, heat pretreatment of graphite brushes, which serve as anodes, has been found to improve power generation by increasing the N/C ratios and reducing the C–O composition on the electrode surface.72
4.3 Conductivity
The anode in a BES acts as a continuous source of electron acceptors, effectively promoting the metabolic reaction rate of anaerobic microorganisms that charge more electrons from organic contaminants.73 Studies have shown that the large internal resistance of BESs, i.e., the low electrical conductivity, especially in systems involving soil and sediments, is an important limiting factor for their efficacy, not only suppressing the metabolism of microorganisms but also resulting in low coulombic efficiency of the substrates.28,57 Therefore, the extracellular electron transfer (EET) efficiency from the microbial cell to the electrode determines the degradation of the substrates and the production of electricity in a BES. BESs are applied mainly in two different forms of media: aqueous media, such as in wastewater treatment, and solid media, such as in soil and sediment remediation. The factors affecting the electron transfer efficiency in the two types of media include the aqueous ion concentration, sediment and soil moisture, salinity, and porosity, etc. Correspondingly, there are many measures to increase the conductivity of the BES, such as increasing the concentration of ions in wastewater treatment; increasing the soil moisture, salinity, and porosity in soil remediation; or by directly adding conductive media, such as carbon materials, into the media.28,57,74,75 The increase in electron transfer efficiency stimulates the growth of electrogenic microorganisms, and thus organic contaminants are effectively degraded. However, the effects of different enhancement measures on microbes are not limited to those listed here. Only a more comprehensive elaboration of the effects on the functional microbes under different conditions can produce the best selection in the application of BESs.
4.3.1 Ion concentration. BESs were originally applied in wastewater treatment, and the ion concentration determines the conductivity of aqueous solutions. Increasing the ion concentration directly reduces the internal resistance of the BES and promotes electron transfer and thus electricity generation.62 Therefore, the effects of different types and concentrations of ions in solution on the microorganisms in the BES need more attention. Some metal ions, such as Na+, K+, Cu2+, and Zn2+, are essential elements for the growth of microorganisms. When these elements are at a certain level, they can promote the growth of microorganisms, and the bacteria maintain a dynamic balance of the desired metal ions through various physiological and metabolic reactions.74 However, when the content exceeds a certain value, conditions are toxic to microorganisms and even cause the bacteria to become nonviable. In an investigation of the effects of different concentrations of NaCl (0.5–2.5%) on the anaerobic biodegradation of petroleum hydrocarbons in an MFC, the MFC performance improved as the concentration increased until the value reached 1%, with the power density rising to 1.06 mW m−2. As the concentration continued to increase, the electricity production decreased, which might have been due to the excessively high ion concentration that reduced the water in the microbial cells and their activity.64,76 For another example, Cu2+ is the core ion that forms the basic coenzyme in various biological processes, but when the content of Cu2+ is excessive, it produces active oxides that combine with biological macromolecules, such as proteins, disrupting their normal physiological functions.77 Studies have also shown that precipitation of heavy metal ions in the anodic compartment can modify the anode and improve electron transport performance, but when this process is excessive, the activities of functional bacteria are suppressed, leading to a significant reduction in voltage generation and COD removal rates.55,78Therefore, although a high ion concentration can reduce the internal resistance of the system, excessive concentrations tend to reduce the reactivity of microorganisms, resulting in an unsatisfactory system performance. To solve this problem, we can weigh these two effects to find an appropriate ion concentration in the actual operation or inoculate bacteria that are resistant to high salinities or are acclimated to heavy metals, which can effectively promote substrate degradation and electricity generation under extreme conditions. For example, two salinity-resistant archaea (Haloferax volcanii and Natrialba magadii) were inoculated in high-salinity BESs with the addition of a redox mediator, and consequently, the power density increased from 0.12 μW cm−2 to 50.98 and 5.39 μW cm−2, respectively.74 In addition, increasing the ion concentration of the cathodic chamber solution can also reduce the internal resistance and increase the power generation without regard to the survival of the microbial communities at the anode in a two-chamber system for treating wastewater. However, the two-chamber system is not convenient for use in actual applications.64,79
Ions also have a similar effect on microorganisms in soil or sediment BESs. Adjusting the type and concentration of ions can make the activity and structure of the microbial community favourable for its required function in the BES. Certainly, it is acceptable to appropriately increase the ion concentration to reduce its large internal resistance or add the necessary nutrient ions for a microorganism to increase its biological activity.74,80 In addition, for systems with high ion concentrations, we can de-salt the medium to lower the concentration. For example, increasing the water content of the soil can increase the solubility of ions, thus promoting electron transfer efficiency, and rinsing contaminated saline-alkaline soils can significantly reduce the salt stress on microorganisms, thereby shortening the start-up time and increasing the production of electricity and hydrocarbon degradation.23
4.3.2 Soil moisture. Water is an indispensable substance for the metabolism of microorganisms, and it is also a medium for the exchange of substances by microorganisms. An increase in moisture in the solid media of a BES can not only reduce the internal resistance of the system, thus enhancing the electron transfer efficiency, but can also increase the activity and metabolic rate of microorganisms, thus promoting the utilization efficiency of substrates. During the bioremediation of saline-alkaline soil contaminated by petroleum hydrocarbons, an increase in the soil water content from 23 to 33% led to an improvement in the electricity generation capacity and the removal of hydrocarbons due to the large increase (two orders of magnitude) in the abundance of hydrocarbon degrading bacteria in the soil near the anode.11 This increase was attributed to the high water content relieving the high salinity stress and improving the mass transfer in soils. In addition, moisture also affects the chemical structure of nutrients, such as nitrogen and carbon sources, in the system, resulting in different levels of bioavailability.81 For example, an increase in moisture can promote the dissolution of hydrophilic contaminants into a water-soluble state, thereby accelerating their metabolic degradation by microbes. At present, solid media BESs have generally been studied under anaerobic environments with water-saturated, waterlogged or water-tight conditions, aiming to reduce the internal resistance of the system and increase current intensity, thereby stimulating the biodegradation of the contaminants.11 In fact, BESs exhibit more advantages in the biodegradation of aged pollutants, when we intend to carry out aerobic treatments, such as aeration, before the BES bioremediation to improve the degradation efficiency in a practical application of a BES.
4.3.3 Soil porosity. The porosity of the soil mainly affects the mass transfer of the system, including the transmission of electrons and the diffusion of pollutants and oxygen. Generally, an increase in porosity increases the efficiency of mass transfer and reduces concentration polarization, thereby increasing the utilization rate of the substrate by microorganisms. After the addition of 33% mass fraction of large-grained sand into soil contaminated by petroleum hydrocarbons, the soil porosity increased from 44.5 to 51.3%, the internal resistance decreased by 46%, and the degradation efficiency for petroleum hydrocarbons increased by 268%, with the stimulation of the growth of the hydrocarbon-degrading bacteria Alcanivorax, in a 135 day operation of a soil MFC.21 Sandy soil has the advantages of good ventilation, strong water and substrate permeability, and high organic mineralization speed but the disadvantages of weak water retention and water supply capacity, while clayey soil has the opposite characteristics; however, loamy soil has the advantages of both. Therefore, proper adjustment of the sand-to-soil ratio in the treatment medium is also an effective method for promoting the metabolism of microorganisms and the performance of a BES in practical applications.
4.3.4 The addition of conductive materials. The application of conductive carbon materials, such as activated carbon and carbon fibre, to the BES is not only used to promote the transfer of electrons in the media but also provides microorganisms with a favourable attached space.10 Activated carbon anodes greatly extended their surface area when multiplying numerous electrogenic bacteria, which play an important role in supporting the high performance of the BES. Compared to noble metals (e.g., platinum), activated carbon is also a cheap catalyst with good catalytic performance, which is affected by the pore structure, specific surface area, content of graphitization and functional groups.82 The addition of conductive carbon fibres into soils is a recently developed novel method to improve MFC performance. These fibres not only effectively shape functional microbial communities in the BES but are also easily separated from the remediated soil and reused.28 Moreover, the addition of silica gel to soils promoted the formation of a silica colloid network, which reduced the soil resistivity.57 The enhancement of electron transfer in the BES by means of conductive materials has received more attention in recent years. Consequently, research on new materials such as magnetic nanoparticles has also become a popular topic, which may play a key role in the practical application of BESs in the future.
4.4 Magnetic field
Previous studies have shown that the application of a magnetic field of a certain intensity to the BES affected its energy production performance and degradation efficiency.83 Magnetic fields acting on microorganisms can cause a series of biological reactions, resulting in macroscopic magnetic effects of microorganisms, which is called the magnetic biological effect.84 Possible mechanisms of magnetic fields on living organisms include the impact on cell membranes and transmembrane signals, the impact on microbial genes, and the impact on cellular enzyme activity.85 The magnetic biological effect is generally applied to the microbial treatment of wastewater in which the activity of functional microorganisms is strengthened by the magnetic field treatment, and the microorganisms in the sludge possess a stronger adsorption and utilization ability relative to the organic matrix in wastewater, so that the biological purification efficiency of the wastewater is improved.86 In a BES, this process may affect the activity of enzymes and the bioelectrochemical activity or biomass of biofilms and thus exert an influence on the organizational structure of microorganisms.87–89 Studies have indicated that a static magnetic field promoted the synthesis of dehydrogenase and lipid peroxidation, exerting a positive effect on the degradation of organic matter.90,91 The occurrence of this effect may be attributed to the promotion of electron transfer on the anode surface in a static magnetic field, thus adjusting the biocatalysis and conversion of electrode-related enzyme assembly.
Under the stimulation of a static magnetic field, the microbial community can also produce more extracellular polymers that allow the microorganisms to resist unfavourable environmental conditions and improve the stability of the biofilm structure.88 In addition, the magnetic field may also affect the internal resistance of the BES and the electroactive matter on the electrode surface. In a study of the influence of a magnetic field on the performance of an MFC, the MFC had a stronger ability to produce electricity and a smaller apparent internal resistance under a magnetic field of 200 mT than the blank control group, and the electroactive substances on the surface of the electrode increased, thereby decreasing the electrode activation resistance.92 Some studies have also found that the nitrification reaction of nitrogen compounds was more thorough under certain magnetic field intensities, and the magnetic field inhibited the production of methane, thereby increasing the coulomb efficiency,93 which was reasonably due to the effect on the microbial community structure and expression of functional genes.
A magnetic field only works within a certain intensity range. Low magnetic field intensity can motivate microbial activity, while a high magnetic field intensity inevitably inhibits the physiological process of microorganisms. Previous study investigated the influence of static magnetic fields of different intensities (20–360 mT) on MFC anode biofilms and found that a static magnetic field of 220 mT resulted in the best MFC performance, with an improvement in production activity, a higher coulomb efficiency and a shortening of the start-up time.88 Studies have also shown that applying magnetic material in a BES facilitated the screening of electrogenic bacteria in the vicinity of ferromagnetic external electron acceptors.94 The effect of the mechanism of the magnetic field on the BES is complex and is not yet fully understood, but an expected role could be played in the application of BESs.
4.5 Substrates
Substrates in the BES include contaminants and co-metabolizing substrates, which are divided into fermentable substrates and non-fermentable substrates. Previous studies indicate that functional microorganisms in BESs are closely related to the substrates, and both the types and concentrations of the substrates play an important role in shaping the structure of the microbial communities.34 At present, BES is studied mainly for the removal of organic pollutants, including petroleum hydrocarbons, pesticides and antibiotics in wastewater. In particular, as a recalcitrant, toxic and complex pollutant, petroleum hydrocarbons have been well studied in BES under different operating conditions (summarized in Table 2). During the treatment process, the environmental media usually contain a range of more complex substrates, in which electrogenic bacteria, degrading bacteria, and element cycle-related bacteria serve as diverse integral components of the system.
Table 2 Performance of BESs for the bioremediation of petroleum hydrocarbons under different operating conditions
Configuration |
Medium |
Pollutants |
pH |
T (°C) |
Inoculum |
Operating time |
Remarks |
Power density (mW m−2) |
Degradation (%) |
Ref. |
Two-chamber MFC with a CEM |
Wastewater |
Petroleum |
Anode: 7 |
20 |
Anaerobically digested sludge |
60 days |
— |
0.6 |
85 |
64 |
30 |
0.72 |
95 |
Cathode: 7 |
40 |
1.15 |
97 |
50 |
0.26 |
60 |
Tubular two-chamber MFC |
Wastewater |
Petroleum |
Anode: 6.3 |
14–23 |
Petroleum acclimated |
155 days |
For in situ deployment |
6.75 |
>90 |
127 |
Tubular single-chamber MFC |
15–25 |
Microbial population |
For ex situ deployment |
≈77 |
Double-cell MFC |
Wastewater |
Diesel range organics |
Cathode: 7 |
30 |
Diesel contaminated groundwater |
21 days |
Diesel: 300 mg L−1 |
31 |
82 |
128 |
Dual-chamber system with a CEM |
Wastewater |
Nitrobenzene |
Cathode: 7 |
25 |
Effluent from acetate-fed MFCs |
A few weeks |
1.5 mM of nitrobenzene |
— |
1.29 mol−3 d−1 |
42 |
Single chambered system with open air cathode |
Sludge |
Petroleum |
Anode: 7 |
29 |
Anaerobic sludge |
17 days |
1.11 g TPH L−1 |
53.11 |
35 |
101 |
3.34 g TPH L−1 |
40.83 |
25 |
5.56 g TPH L−1 |
17.52 |
15 |
11.1 g TPH L−1 |
12.02 |
10 |
Dual-chambered MFC |
Sediment |
Benzoate and toluene |
— |
25 |
Cultures of G. metallireducens |
Over 600 hours |
Application of potentiostat |
— |
— |
16 |
Sediment column MFC |
Sediment |
Petroleum |
Sediment: 7.2 |
30 |
— |
66 days |
TPH: 16 000 mg kg−1 |
— |
24 |
7 |
U-tube air-cathode MFC |
Soil |
Petroleum |
Anode: 6.8–7.1 |
23 ± 3 |
— |
25 days |
Water content: 33% |
0.85 |
15.2 |
11 |
Tubular system with air cathode |
Soil |
Petroleum |
Soil: 6.16 |
20–22 |
— |
64 days |
Biochar anode |
|
78.7 |
10 |
Carbon cloth anode |
73.1 |
Single-chamber with air cathode |
Soil |
Petroleum |
6.75–8 |
30 |
— |
135 days |
Soil to sand 5 : 1 |
0.087 |
15 |
21 |
7–8.1 |
Soil to sand 2 : 1 |
0.094 |
22 |
Single-chamber with air cathode |
Soil |
Petroleum |
8.71 |
30 |
— |
135 days |
Glucose addition 0.1% |
35 |
15 |
22 |
8.46 |
Glucose addition 0.5% |
43 |
14 |
Single-chamber with air cathode |
Soil |
Petroleum |
7.95 |
30 |
— |
65 days |
Soil via rinsed salt (RS) |
|
26 |
28 |
7.96 |
Soil mixed with carbon fibre (MC) |
40 |
8.1 |
RS + MC |
60 |
To study the effect of solely substrate on the microbial community structure, scholars have studied BESs fed with particular substrates as a carbon source. In MFCs fed on acetate, lactate, and glucose as substrates, respectively, the model electrogenic bacteria Geobacter sulfurreducens and an uncultured bacterium affiliated with Bacteroidetes were found on all anodes, while certain species of Firmicutes were found only in the glucose-fed MFC, which may play a role in converting complex carbon sources to simple substances and scavenging oxygen.95 Other studies have also shown that Clostridium and Bacilli in Firmicutes were commonly associated with the glucose-fed BES, whereas the enrichment of Geobacter-like species was always found in the BES with acetate as a substrate.96 The enrichment in β-Proteobacteria and the reduction in γ-Proteobacteria at all anodes of MFCs fed with acetate, butyrate, and glucose (this pattern did not hold for the one fed with propionate) further confirmed that different types of carbon sources induced the growth of different microorganisms.34 Table 3 shows the unique microbial communities under different types of pure substrates as electron donors.
Table 3 Unique microbial communities and electrochemical performances under different types of pure substrates as electron donors
Pure substrate |
Concentration |
Inoculum |
Dominant bacteria |
Substrate-related bacteria |
Main electrogenic bacteria |
Power density (mW m−2) |
Coulombic efficiency (%) |
Ref. |
Acetate |
5 mM |
Activated sludge |
Alphaproteobacteria |
— |
Geobacter |
— |
Over 70 |
129 |
Cysteine |
385 mg L−1 |
Anaerobic marine sediment |
Gammaproteobacteria |
Shewanella and Pseudoalteromonas |
Shewanella and Pseudomonas |
19 |
— |
130 |
Acetate |
200 mg L−1 |
Anaerobic sludge |
Deltaproteobacteria |
Betaproteobacterial |
Geobacter |
48.4 ± 0.3 |
72.3 ± 5.3 |
131 |
Lactate |
Deltaproteobacteria |
Betaproteobacterial |
Geobacter |
52.0 ± 4.7 |
61.2 ± 0.4 |
Glucose |
Deltaproteobacteria |
Spirochaetales and Firmicutes |
Geobacter |
40.3 ± 3.9 |
63.9 ± 2.2 |
Ethanol |
10 mM |
Anaerobic sludge |
Betaproteobacterial |
Azoarcus and Desulfuromonas |
Geobacter |
40 ± 2 |
42–61 |
132 |
Cellulose |
7.5 g L−1 |
Rumen microorganisms |
Firmicutes |
Clostridium, Ruminococcus and Geovibrio |
Geobacter and Comamonadaceae |
55 |
— |
133 |
Formate |
20 mM |
Anaerobic digested fluid |
Epsilonproteobacteria and Clostridia |
Acetobacterium and Arcobacter |
Geobacter |
— |
5.3 |
134 |
Acetate |
0.5–2.2 mM |
Anaerobic sludge |
Betaproteobacterial |
— |
Geobacter |
64.3 |
72.3 |
34 |
Butyrate |
Betaproteobacterial |
— |
Geobacter |
51.4 |
43 |
Glucose |
Betaproteobacterial |
— |
Geobacter |
156 |
36 |
Acetate |
1000 mg COD L−1 |
Domestic wastewater |
Bacteroidetes and Deltaproteobacteria |
Geobacter and Chlorobia |
Geobacter and Bacteroides |
61 ± 1 |
19.6 ± 0.3 |
96 |
Butyrate |
Bacteroidetes, Clostridia and Alphaproteobacteria |
|
Geobacter |
55 ± 1 |
18.9 ± 0.2 |
Glucose |
Bacteroidetes and Deltaproteobacteria |
Clostridium and Bacilli |
|
52 ± 1 |
16.9 ± 0.2 |
Propionate |
5 mM |
— |
Deltaproteobacteria |
— |
Geobacter |
37.4 |
31.5 ± 0.6 |
135 |
Acetate |
2 g L−1 |
Livestock wastewater |
Deltaproteobacteria |
Desulfuromonas |
Desulfuromonas |
1063 |
— |
136 |
Starch |
Firmicutes |
Enterococcus |
Geobacter |
660 |
— |
Glucose |
Firmicutes |
Carnobacterium |
Geobacter |
632 |
— |
Furthermore, investigating the response of the microbial community structure to substrate changes is an important method for revealing the effects of substrates on microbes. Studies have shown that BESs developed from different carbon sources have different adaptation abilities to substrate changes. BESs enriched with glucose had better performance than BESs enriched with acetate or butyrate, and BESs enriched with acetate cannot use glucose immediately after the substrate is changed.96 These results showed that the addition of glucose can increase the substrate utilization and stability of the system, but numerous non-producer bacteria also utilize this energy source, thereby reducing the coulomb efficiency. The study of pure substrate-fed BESs provides insight into the bioremediation of complex contaminated substrates with greater microbial diversity. At present, the electrochemical treatments of wastewater from different sources (e.g., factories, pig farms, breweries), pesticides (e.g., atrazine, hexachlorobenzene, lindane), antibiotics and petroleum hydrocarbons have been well studied, and it is meaningful to explore the relationships among functional microorganisms under specific substrate conditions.
In addition, substrates with different morphologies and bioavailabilities could exert an influence on the performance of the BES. A study showed that MFCs constructed from agricultural soil had an electricity generation approximately 17 times higher than forest soil-based MFCs. Although the soil organic carbon of the former was far lower than that of the latter, the quality of the available organic matter played a more important role in shaping the high performance community structure.97 Studies have further indicated that the performance of the system is inversely proportional to the complexity of the substrate, which is proportional to the bioavailability of the substrate.98,99
In addition to the type and morphology of the substrate, its effective concentration is a non-negligible factor for the formation of microbial community structures in BESs. One study investigated the effects of fuel concentration on the performance of MFCs.100 The results showed that the power density increased with the fuel concentration. A higher concentration of contaminants can provide more carbon sources for hydrocarbon-degrading bacteria but also impair the activity of electrogenic bacteria. For instance, the amount of substrate removal increased with the increase in the concentration in the biodegradation of real field petroleum sludge with different organic loads of 1.11, 3.34, 5.56, 11.10 g TPH L−1, but the highest electricity production capacity was obtained in the lowest concentration reactor. Furthermore, bioaugmentation of anodic microflora in the highest concentration reactor enhanced the electricity production ability of the BES,101 suggesting that there was an optimum concentration of contaminants for microbial utilization and that special approaches should be taken depending on different types and concentrations of substrates in the actual environmental media.102 For example, bioaugmentation of electrogenic bacteria may be an effective method for highly contaminated systems, or a BES could be applied in the secondary treatment of highly polluted media in which the concentration is suitable for effective utilization by microorganisms.
4.6 Inoculum
The function of the BES can be achieved using a pure strain or a mixture of bacteria, and pure bacteria strains can be isolated and enriched from the anode of a BES or selected according to the known electrogenic microorganisms.103,104 An MFC inoculated with a pure bacteria strain is conducive to revealing the electron transfer mechanism. Studies have shown that the electron transfer efficiency of pure-culture MFC systems is higher than that of MFCs inoculated with heterogeneous bacteria.20,103,105 Additionally, the inoculation of domesticated electrogenic bacteria in a BES can increase the charge output of the system under extreme conditions. For example, a high ion concentration can reduce the internal resistance of a BES, but it increases the stress on the growth of microorganisms. Researchers inoculated two highly salt-tolerant bacteria in a high ion concentration in an MFC, and the power densities were more than 10–100 times greater than the control.74 However, anode biofilms in pure-culture BESs are thinner than those in mixed-culture BESs, reducing the proton transfer ability and nutrient availability, which are adverse to the performance.106 In addition, the pure-culture BES requires strict operating conditions and a sterile environment, resulting in high costs. In a system that is simultaneously inoculated with multiple inoculations, the internal resistance of the anode was lower than that of a reactor inoculated with one type of inoculation, indicating that the mixed consortia played a mutually reinforcing role.105 This effect may occur because the mixed inoculation can effectively reduce the activation loss of the anode and the mechanism by which these two electrogenic microorganisms transport electrons is different, leading to an increase in the utilization efficiency of substrates.
In actual operation, we can directly use indigenous microorganisms to oxidize the substrate and transfer the electrons to the anode, which is simple and economical. Furthermore, a mixed inoculation can reduce the accumulation of metabolites on the anode,107 possibly due to the different microorganisms using different types of carbon sources and the synergistic relationship among the microorganisms. Notably, the type of inoculum source also has a great influence on BES performance. The community structure of the anode biofilm can be shaped by different initial inocula, resulting in different electricity production capabilities.108–110 In studies on the effects of the inoculum on MFC power output and microbial communities, the results indicated that river sediment-inoculated MFC had a higher power density than MFCs inoculated with activated sludge, anaerobic sludge, garden soil, and wastewater because the river sediment-inoculated MFC exhibited the best anode biofilm, as confirm by scanning electron microscopy.104,109 In addition, reactors inoculated with aerobic sludge have higher maximum voltages and longer high pressure durations than anaerobic sludge-inoculated reactors, which could be ascribed to the former containing a large number of facultative electrogenic bacteria.47 Moreover, in three reactors inoculated with anaerobic sludge from a wastewater plant, rice paddy field soil, and coastal lagoon sediment, the MFC inoculated with coastal lagoon sediment showed the highest performance in terms of the treatment time, and the results showed that the three different inoculum sources all contained functional electrical production groups, whereas different community members controlled the performances of the MFCs.44 Therefore, it is concluded that the microbial constitution of the inoculum determines the BES performance. For better application in practice, we can inoculate the reactor with the previous reactor effluent, which is enriched with numerous functional electrogenic bacteria, thereby reducing the start-up time and increasing the degradation rate of the substrates.111
4.7 Reactor design parameters
The configuration of the BES involves various components, including the anode, cathode and PEM, and improvements in the configuration can be combined with the optimization of other factors to further improve the overall system performance. A traditional BES consists of an anode chamber and a cathode chamber separated by a proton-permeable material, such as salt bridge or a PEM.14 Microorganisms grow in the anode chamber and produce electrons from the organic matter, while the cathode chamber usually requires aeration to provide sufficient dissolved oxygen (DO) as an electron acceptor or external power supply to reduce protons.26 The energy required for aeration and the cost of an ion exchange membrane in a dual-chamber system hinder the use of this style of system in the practical bioremediation of contaminated substrates.112 Moreover, the aeration of the cathode chamber inevitably impairs the anaerobic environment of the anode chamber and affects the activity of the functional microorganisms.46,106 This highlights the advantages of a single-chamber system that does not require aeration, which is replaced by the direct transfer of oxygen from the air to the cathode, thus alleviating the influence of oxygen diffusion to the anode. The single-chamber system exhibits great potential in practical applications because of its high space utilization efficiency and low cost. However, this system still has deficiencies in that the reduction reaction occurring at the cathode is slow, necessitating the use of catalysts, and the anode microbial activity is restricted by the absence of a PEM.75,113
As the core of a BES, the anode is the substrate to which the functional microorganisms are attached. The choice of anode affects not only the amount of attached microorganism biomass but also the efficiency of the electron transfer from the microorganism cells to the surface of the electrode. Carbon-based materials are widely used in BESs because of their good electrical conductivity and low cost, and these materials mainly include carbon paper, carbon cloth, carbon felt, carbon brushes, graphite rods, graphite particles and the like.3,12 Various carbon-based materials have different electrochemical properties due to their specific surface area and biocompatibility. Three types of anode materials, graphite felt, graphite foam and a graphite rod, were applied in glucose-fed BESs. The results showed that the best performance was exhibited by the graphite felt, followed by the graphite foam, and the good performance was attributed to the high surface area for microbial attachment and the low electrode internal resistance.114 Similarly, graphite brush anodes with higher surface areas and an excellent pore structure generate much more power output in BESs than carbon paper electrodes and possess the potential to be scaled up in larger BESs.72,115 Although graphite brush anodes cannot be directly used for the bioremediation of solid media, the combination of carbon fibres incorporated into environmental media and carbon rods forming a composite anode similar to a carbon brush is promising in site remediation.28
Low electron transfer efficiency is the main factor limiting BES, making the modification of anode materials an effective way to improve system performance. Anodic modification typically introduces important functional groups on the surface of the electrode, allowing the negatively charged bacterial cell wall to interact with positively charged functional groups, thereby accelerating electron transport and increasing electrode efficiency.116 For example, the carbon cloth anode is directly modified with ammonia gas under high temperature conditions, increasing the positive charge number on the electrode surface, and the power density is increased from 1330 to 1970 mW m−2.117 Higher COD removal and coulombic efficiency were also observed through the modification of the anode by polydopamine (PDA) and attributed to the introduction of abundant amino groups.45 Furthermore, specific functional groups, such as quinoid and amide, can also be introduced to the surface of the anode by electrochemical oxidation and can directly interact with the c-type cytochrome on the outer wall of electrogenic bacteria.118,119 Novel nanomaterials are gradually being used for the modification of anodes in BESs because of their excellent physical and chemical properties. Powdered carbon nanotubes added in the anode chamber can be combined with G. subreducens to form an effective anode composite membrane,120 and graphene can promote microbial adhesion on the surface of the anode.121 Inevitably, the use of special materials and complex modification processes increases the cost of the system and affects its practical application. Therefore, the key to anode material modification is to find ways to effectively improve its electricity production performance while reducing the cost.
4.8 Others
In addition to the influencing factors mentioned above, the system's external loads, potentials, electric field strengths, selection of cathode catalysts, addition of electron mediators or other nutrient sources also exert impacts on the shaping of the BES microbial community structures and the reduction of substrates. For example, the external resistance of different magnitudes results in unequal current intensities, and higher current intensity can usually stimulate microorganisms to degrade organic contaminants better, but a smaller external resistance is not conducive to the output of bioelectricity.122 Moreover, the increase in the anode potential from −400 to +200 mV (versus Ag/AgCl) promoted electricity generation, whereas the higher potential than +400 mV (versus Ag/AgCl) impaired the ability of the BES to produce electricity because of a decrease in the microbial biomass; therefore, an appropriate potential range is necessary for the functional microorganisms in BESs.123 Additionally, electric fields with different intensities can affect the migration of ions in the BES and thus exert different degrees of stress on microorganisms.124 Various cathode catalysts have different redox potentials and electrocatalytic activities, and the potential poisoning effects of the catalyst on microorganisms should be avoided in practice.125 Although the addition of mediators into a BES can promote electron delivery from the electrochemically active bacteria to the anode, the permeability of the cell membrane to the redox mediator molecules and the toxicity of the redox mediator should also be considered.64 For a system with sufficient carbon sources, the addition of a nitrogen source is bound to promote the quantity and activity of the microorganisms, which require a specific ratio of carbon to nitrogen, commonly C/N = 10
:
1.126
5. Conclusion
The BES must be robust enough to be applied in the field for bioremediation or energy production, and research is needed to investigate the functional microorganisms under the influence of various conditions.29 It is difficult to clearly study the effects of various interaction measures on the microbial community structure, and yet such research makes sense for the exploration of the microbiological function mechanism and the optimization of BESs to better guide its transition from the laboratory to actual applications. Here, we summarize the effects of various factors on functional microorganisms and contaminant removal in BESs and provide corresponding optimization measures.
Functional microorganisms in BESs, including electrogenic bacteria, degrading bacteria, and element cycle-related bacteria, harvest electrons from the degradation of substrates and promote the removal of contaminants, and these communities are affected by various environmental conditions. Here, we systematically summarized the effects of various enhancements (operational factors) on the BES performance with respect to the functional microorganisms and contaminant removal, with the aim of determining the optimal conditions during polluted environmental remediation by BESs and the effective measures that should be taken. Admittedly, the potential of BESs to remediate contaminated substrates needs to be further explored, and there are still some challenges yet to overcome in the use of BESs to actually remove contaminants. Therefore, the BES mechanism should be studied more deeply and comprehensively. At present, research on microorganisms in BESs remains mainly at the species identification stage, and we should therefore pay more attention to the specific roles of functional genes and functional proteins under particular conditions of the system. The study of electrogenic microorganisms is still an area worthy of attention, and the study of electrogenic microbial secretions and the biological interaction with coexisting neighbours involved in carbon, nitrogen, phosphorus and sulphur transformation will lead to new discoveries. Undoubtedly, the great potential for BESs in pollution control and energy recovery requires us to be committed to its practical application in the future.
Conflicts of interest
The authors declare that they have no conflict of interest.
Acknowledgements
This study was financially supported by the National Key R&D Program of China (No. 2017YFD0800704), the National Natural Science Foundation of China (No. 41601536) and the Central Public-Interest Scientific Institution Basal Research Fund (AEPI).
References
- D. R. Bond, D. E. Holmes, L. M. Tender and D. R. Lovley, Science, 2002, 295, 483–485 CrossRef CAS PubMed.
- D. R. Lovley, Curr. Opin. Biotechnol., 2006, 17, 327–332 CrossRef CAS PubMed.
- B. E. Logan and K. Rabaey, Science, 2012, 337, 686–690 CrossRef CAS PubMed.
- C. Zhao, P. P. Gai, C. H. Liu, X. Wang, H. Xu, J. R. Zhang and J. J. Zhu, J. Mater. Chem. A, 2013, 1, 12587–12594 RSC.
- W. W. Li, H. Q. Yu and Z. He, Energy Environ. Sci., 2014, 7, 911–924 RSC.
- H. M. Wang, H. P. Luo, P. H. Fallgren, S. Jin and Z. J. Ren, Biotechnol. Adv., 2015, 33, 317–334 CrossRef CAS PubMed.
- J. M. Morris and S. Jin, J. Hazard. Mater., 2012, 213, 474–477 CrossRef PubMed.
- W. W. Li and H. Q. Yu, Biotechnol. Adv., 2015, 33, 1–12 CrossRef CAS PubMed.
- D. Y. Huang, S. G. Zhou, Q. Chen, B. Zhao, Y. Yuan and L. Zhuang, Chem. Eng. J., 2011, 172, 647–653 CrossRef CAS.
- L. Lu, T. Huggins, S. Jin, Y. Zuo and Z. J. Ren, Environ. Sci. Technol., 2014, 48, 4021–4029 CrossRef CAS PubMed.
- X. Wang, Z. Cai, Q. X. Zhou, Z. N. Zhang and C. H. Chen, Biotechnol. Bioeng., 2012, 109, 426–433 CrossRef CAS PubMed.
- X. J. Li, X. Wang, L. P. Weng, Q. X. Zhou and Y. T. Li, Energy Technol., 2017, 5, 1156–1164 CrossRef CAS.
- B. E. Logan, B. Hamelers, R. A. Rozendal, U. Schrorder, J. Keller, S. Freguia, P. Aelterman, W. Verstraete and K. Rabaey, Environ. Sci. Technol., 2006, 40, 5181–5192 CrossRef CAS PubMed.
- D. R. Lovley, Curr. Opin. Biotechnol., 2008, 19, 564–571 CrossRef CAS PubMed.
- Z. He, Environ. Sci. Technol., 2013, 47, 332–333 CrossRef CAS PubMed.
- T. Zhang, S. M. Gannon, K. P. Nevin, A. E. Franks and D. R. Lovley, Environ. Microbiol., 2010, 12, 1011–1020 CrossRef CAS PubMed.
- A. Dominguez-Garay, J. R. Quejigo, U. Dorfler, R. Schroll and A. Esteve-Nunez, Microb. Biotechnol., 2018, 11, 50–62 CrossRef CAS PubMed.
- C. Zhao, P. P. Gai, C. H. Liu, X. Wang, H. Xu, J. R. Zhang and J. J. Zhu, J. Mater. Chem. A, 2013, 1, 12587–12594 RSC.
- X. H. Peng, H. B. Yu, X. Wang, Q. X. Zhou, S. J. Zhang, L. J. Geng, J. W. Sun and Z. Cai, Bioresour. Technol., 2012, 121, 450–453 CrossRef CAS PubMed.
- J. W. Zhang, E. R. Zhang, K. Scott and J. G. Burgess, Environ. Sci. Technol., 2012, 46, 2984–2992 CrossRef CAS PubMed.
- X. J. Li, X. Wang, Z. J. Ren, Y. Zhang, N. Li and Q. Zhou, Chemosphere, 2015, 141, 62–70 CrossRef CAS PubMed.
- X. J. Li, X. Wang, L. Wan, Y. Zhang, N. Li, D. Li and Q. Zhou, J. Chem. Technol. Biotechnol., 2016, 91, 267–275 CrossRef CAS.
- X. J. Li, X. Wang, Y. Zhang, Q. Zhao, B. Yu, Y. Li and Q. Zhou, Sci. Rep., 2016, 6, 32861 CrossRef CAS PubMed.
- L. Wang, Y. Liu, J. Ma and F. Zhao, Water Res., 2016, 88, 322–328 CrossRef CAS PubMed.
- K. Rabaey and W. Verstraete, Trends Biotechnol., 2005, 23, 291–298 CrossRef CAS PubMed.
- B. E. Logan, Nat. Rev. Microbiol., 2009, 7, 375–381 CrossRef CAS PubMed.
- C. Koch, B. Korth and F. Harnisch, Microb. Biotechnol., 2018, 11, 22–38 CrossRef PubMed.
- X. J. Li, X. Wang, Q. Zhao, L. L. Wan, Y. T. Li and Q. X. Zhou, Biosens. Bioelectron., 2016, 85, 135–141 CrossRef CAS PubMed.
- M. Oliot, B. Erable, M. L. De Solan and A. Bergel, Electrochim. Acta, 2017, 258, 134–142 CrossRef CAS.
- X. X. Cao, M. Z. Fan, P. Liang and X. Huang, Chem. Res. Chin. Univ., 2009, 30, 983–987 CAS.
- S. Srikanth, M. Kumar and S. K. Puri, Bioresour. Technol., 2018, 265, 506–518 CrossRef CAS PubMed.
- L. Lu, D. Xing and N. Ren, Environ. Sci. Technol., 2012, 46, 6874–6881 CrossRef CAS PubMed.
- M. E. Kokko, A. E. Makinen and J. A. Puhakka, Adv. Biochem. Eng., 2016, 156, 263–292 CAS.
- K. J. Chae, M. J. Choi, J. W. Lee, K. Y. Kim and I. S. Kim, Bioresour. Technol., 2009, 100, 3518–3525 CrossRef CAS PubMed.
- D. R. Lovley, J. D. Coates, E. L. BluntHarris, E. J. P. Phillips and J. C. Woodward, Nature, 1996, 382, 445–448 CrossRef CAS.
- T. Saito, M. Mehanna, X. Wang, R. D. Cusick, Y. J. Feng, M. A. Hickner and B. E. Logan, Bioresour. Technol., 2011, 102, 395–398 CrossRef CAS PubMed.
- A. Sikora, J. Wojtowicz-Sienko, P. Piela, U. Zielenkiewicz, K. Tomczyk-Zak, A. Chojnacka, R. Sikora, P. Kowalczyk, E. Grzesiuk and M. Blaszczyk, J. Microbiol. Biotechnol., 2011, 21, 305–316 CAS.
- K. M. Handley, N. C. VerBerkmoes, C. I. Steefel, K. H. Williams, I. Sharon, C. S. Miller, K. R. Frischkorn, K. Chourey, B. C. Thomas, M. B. Shah, P. E. Long, R. L. Hettich and J. F. Banfield, ISME J., 2013, 7, 800–816 CrossRef CAS PubMed.
- M. Daghio, F. Aulenta, E. Vaiopoulou, A. Franzetti, J. B. Arends, A. Sherry, A. Suarez-Suarez, I. M. Head, G. Bestetti and K. Rabaey, Water Res., 2017, 114, 351–370 CrossRef CAS PubMed.
- P. K. Dutta, J. Keller, Z. G. Yuan, R. A. Rozendal and K. Rabaey, Environ. Sci. Technol., 2009, 43, 3839–3845 CrossRef CAS PubMed.
- Y. Zheng, Y. Xiao, Z.-H. Yang, S. Wu, H.-J. Xu, F.-Y. Liang and F. Zhao, Process Biochem., 2014, 49, 1345–1351 CrossRef CAS.
- Y. Mu, R. A. Rozendal, K. Rabaey and J. Keller, Environ. Sci. Technol., 2009, 43, 8690–8695 CrossRef CAS PubMed.
- J. D. Coates, R. T. Anderson, J. C. Woodward, E. J. P. Phillips and D. R. Lovley, Environ. Sci. Technol., 1996, 30, 2784–2789 CrossRef CAS.
- S. Ishii, S. Suzuki, Y. Yamanaka, A. Wu, K. H. Nealson and O. Bretschger, Bioelectrochemistry, 2017, 117, 74–82 CrossRef CAS PubMed.
- Z. W. Du, H. R. Li and T. Y. Gu, Biotechnol. Adv., 2007, 25, 464–482 CrossRef CAS PubMed.
- G.-C. Gil, I.-S. Chang, B. H. Kim, M. Kim, J.-K. Jang, H. S. Park and H. J. Kim, Biosens. Bioelectron., 2003, 18, 327–334 CrossRef CAS PubMed.
- S. S. Lu, Y. G. Zhao and R. Liu, Adv. Mater. Res., 2013, 724–725, 762–768 Search PubMed.
- F. Liang, Y. Xiao and F. Zhao, Chem. Eng. J., 2013, 218, 147–153 CrossRef CAS.
- J. Rousk, E. Baath, P. C. Brookes, C. L. Lauber, C. Lozupone, J. G. Caporaso, R. Knight and N. Fierer, ISME J., 2010, 4, 1340–1351 CrossRef PubMed.
- G. S. Jadhav and M. M. Ghangrekar, Bioresour. Technol., 2009, 100, 717–723 CrossRef CAS PubMed.
- B. Min, J. Kim, S. Oh, J. M. Regan and B. E. Logan, Water Res., 2005, 39, 4961–4968 CrossRef CAS PubMed.
- Y. P. Liu, Y. H. Wang, B. S. Wang and Q. Y. Chen, Int. J. Hydrogen Energy, 2014, 39, 14191–14195 CrossRef CAS.
- S. E. Oh, S. Van Ginkel and B. E. Logan, Environ. Sci. Technol., 2003, 37, 5186–5190 CrossRef CAS PubMed.
- S. Venkata Mohan, G. Mohanakrishna, B. P. Reddy, R. Saravanan and P. N. Sarma, Biochem. Eng. J., 2008, 39, 121–130 CrossRef CAS.
- N. Habibul, Y. Hu and G. P. Sheng, J. Hazard. Mater., 2016, 318, 9–14 CrossRef CAS PubMed.
- P. Clauwaert, J. Desloover, C. Shea, R. Nerenberg, N. Boon and W. Verstraete, Biotechnol. Lett., 2009, 31, 1537–1543 CrossRef CAS PubMed.
- A. Dominguez-Garay, A. Berna, I. Ortiz-Bernad and A. Esteve-Nunez, Environ. Sci. Technol., 2013, 47, 2117–2122 CrossRef CAS PubMed.
- A. Kadier, P. Abdeshahian, M. S. Kalil, H. Abu Hasan and A. A. Hamid, Iran. J. Sci. Technol. A, 2018, 42, 237–244 CrossRef.
- Y. C. Liu, L. Z. Li, Y. Wu, W. Tian, L. P. Zhang, L. Xu, Q. R. Shen and B. Shen, Bioresour. Technol., 2010, 101, 310–316 CrossRef CAS PubMed.
- T. Kim, J. An, H. Lee, J. K. Jang and I. S. Chang, Bioresour. Technol., 2016, 215, 290–295 CrossRef CAS PubMed.
- I. S. Michie, J. R. Kim, R. M. Dinsdale, A. J. Guwy and G. C. Premier, Appl. Microbiol. Biotechnol., 2011, 92, 419–430 CrossRef CAS PubMed.
- H. Liu, S. A. Cheng and B. E. Logan, Environ. Sci. Technol., 2005, 39, 5488–5493 CrossRef CAS PubMed.
- Y. Liu, V. Climent, A. Berna and J. M. Feliu, Electroanalysis, 2011, 23, 387–394 CrossRef CAS.
- O. Adelaja, T. Keshavarz and G. Kyazze, J. Hazard. Mater., 2015, 283, 211–217 CrossRef CAS PubMed.
- I. S. Michie, J. R. Kim, R. M. Dinsdale, A. J. Guwy and G. C. Premier, Energy Environ. Sci., 2011, 4, 1011–1019 RSC.
- D. R. Bond and D. R. Lovley, Appl. Environ. Microbiol., 2003, 69, 1548–1555 CrossRef CAS PubMed.
- A. C. Yiannopoulos, I. D. Manariotis and C. V. Chrysikopoulos, Bioresour. Technol., 2008, 99, 7742–7749 CrossRef CAS PubMed.
- I. S. Michie, J. R. Kim, R. M. Dinsdale, A. J. Guwy and G. C. Premier, Energy Environ. Sci., 2011, 4, 1011–1019 RSC.
- S. A. Cheng, D. F. Xing and B. E. Logan, Biosens. Bioelectron., 2011, 26, 1913–1917 CrossRef CAS PubMed.
- H. Deng, Y.-C. Wu, F. Zhang, Z.-C. Huang, Z. Chen, H.-J. Xu and F. Zhao, Pedosphere, 2014, 24, 330–338 CrossRef CAS.
- M. A. Barique, E. Tsuchida, A. Ohira and K. Tashiro, ACS Omega, 2018, 3, 349–360 CrossRef CAS.
- Y. J. Feng, Q. Yang, X. Wang and B. E. Logan, J. Power Sources, 2010, 195, 1841–1844 CrossRef CAS.
- X. Cao, H. L. Song, C. Y. Yu and X. N. Li, Bioresour. Technol., 2015, 189, 87–93 CrossRef CAS PubMed.
- X. C. Abrevaya, N. Sacco, P. J. D. Mauas and E. Corton, Extremophiles, 2011, 15, 633–642 CrossRef CAS PubMed.
- X. J. Li, X. Wang, Y. Y. Zhang, N. S. Gao, D. S. Li and Q. X. Zhou, Int. J. Electrochem. Sci., 2015, 10, 5086–5100 CAS.
- V. G. Paul, S. D. Minteer, B. L. Treu and M. R. Mormile, Environ. Technol., 2014, 35, 1003–1011 CrossRef CAS PubMed.
- A. K. Sharma, S. T. Pavlova, J. Kim, J. Kim and L. M. Mirica, Metallomics, 2013, 5, 1529–1536 RSC.
- H. Deng, Y. B. Jiang, Y. W. Zhou, K. Shen and W. H. Zhong, Eur. J. Soil Sci., 2015, 66, 369–377 CrossRef CAS.
- X. Wang, S. A. Cheng, X. Y. Zhang, X. Y. Li and B. E. Logan, Int. J. Hydrogen Energy, 2011, 36, 13900–13906 CrossRef CAS.
- V. G. Paul, S. D. Minteer, B. L. Treu and M. R. Mormile, Environ. Technol., 2014, 35, 1003–1011 CrossRef CAS PubMed.
- S. L. O'Brien, J. D. Jastrow, D. A. Grimley and M. A. Gonzalez-Meler, Glob. Change Biol., 2010, 16, 2573–2588 Search PubMed.
- X. H. Peng, H. B. Yu, X. Wang, Q. X. Zhou, S. J. Zhang, L. J. Geng, J. W. Sun and Z. Cai, Bioresour. Technol., 2012, 121, 450–453 CrossRef CAS PubMed.
- S. Dunca, D.-E. Creanga, O. Ailiesei and E. Nimitan, J. Magn. Magn. Mater., 2005, 289, 445–447 CrossRef CAS.
- R. L. Moore, Can. J. Microbiol., 1979, 25, 1145 CrossRef CAS PubMed.
- M. Lebkowska, A. Rutkowska-Narozniak, E. Pajor, A. Tabernacka and M. Zaleska-Radziwill, Environ. Sci. Pollut. Res., 2018, 25, 22571–22583 CrossRef CAS PubMed.
- C. Niu, J. Geng, H. Ren, L. Ding, K. Xu and W. Liang, Bioresour. Technol., 2013, 150, 156–162 CrossRef CAS PubMed.
- W. W. Li, G. P. Sheng, X. W. Liu, P. J. Cai, M. Sun, X. Xiao, Y. K. Wang, Z. H. Tong, F. Dong and H. Q. Yu, Biosens. Bioelectron., 2011, 26, 3987–3992 CrossRef CAS PubMed.
- Y. N. Zhao, X. F. Li, Y. P. Ren and X. H. Wang, RSC Adv., 2016, 6, 82301–82308 RSC.
- R. Wu, X. Tian, S. Wu, Y. Liu, Y. Jiang and F. Zhao, Chem. Res. Chin. Univ., 2015, 36, 1730–1736 CAS.
- H. Sahebjamei, P. Abdolmaleki and F. Ghanati, Bioelectromagnetics, 2007, 28, 42–47 CrossRef CAS PubMed.
- M. Lebkowska, A. Rutkowska-Narozniak, E. Pajor and Z. Pochanke, Bioresour. Technol., 2011, 102, 8777–8782 CrossRef CAS PubMed.
- Y. Yin, G. Huang, Y. Tong, Y. Liu and L. Zhang, J. Power Sources, 2012, 237, 58–63 CrossRef.
- Q. Tao and S. Zhou, Appl. Microbiol. Biotechnol., 2014, 98, 9879–9887 CrossRef CAS PubMed.
- L. Kiseleva, J. Briliute, I. V. Khilyas, D. J. Simpson, V. Fedorovich, M. Cohen and I. Goryanin, Biomed Res Int., 2015, 2015, 582471 Search PubMed.
- S. Jung and J. M. Regan, Appl. Microbiol. Biotechnol., 2007, 77, 393–402 CrossRef CAS PubMed.
- Y. F. Zhang, B. Min, L. P. Huang and I. Angelidaki, Bioresour. Technol., 2011, 102, 1166–1173 CrossRef CAS PubMed.
- S. J. Dunaj, J. J. Vallino, M. E. Hines, M. Gay, C. Kobyljanec and J. N. Rooney-Varga, Environ. Sci. Technol., 2012, 46, 1914–1922 CrossRef CAS PubMed.
- B. D. Qin, X. D. Dong, J. Y. Wang, C. Y. Sun, D. P. Guo and Y. F. Li, Environmental Technology and Resource Utilization II, 2014, vol. 675–677, pp. 592–595 Search PubMed.
- S. Ahmed, E. Rozaik and H. Abdel-Halim, Pol. J. Environ. Stud., 2016, 25, 503–510 CrossRef CAS.
- H. Moon, I. S. Chang and B. H. Kim, Bioresour. Technol., 2006, 97, 621–627 CrossRef CAS PubMed.
- K. Chandrasekhar and S. Venkata Mohan, Bioresour. Technol., 2012, 110, 517–525 CrossRef CAS PubMed.
- A. Tremouli, T. Vlassis, G. Antonopoulou and G. Lyberatos, Waste Biomass Valorization, 2016, 7, 1339–1347 CrossRef CAS.
- J. C. Biffinger, J. Pietron, R. Ray, B. Little and B. R. Ringeisen, Biosens. Bioelectron., 2007, 22, 1672–1679 CrossRef CAS PubMed.
- H. J. Lin, X. Wu, C. Miller and J. Zhu, Biomass Bioenergy, 2013, 54, 170–180 CrossRef CAS.
- J. Sun, Y. Y. Hu, Z. Bi and Y. Q. Cao, J. Power Sources, 2009, 187, 471–479 CrossRef CAS.
- A. E. Franks, N. Malvankar and K. P. Nevin, Biofuels, 2010, 1, 589–604 CrossRef CAS.
- S. P. Jung, M. H. Yoon, S. M. Lee, S. E. Oh, H. Kang and J. K. Yang, Int. J. Electrochem. Sci., 2014, 9, 315–326 Search PubMed.
- P. T. Ha, B. Tae and I. S. Chang, Energy Fuels, 2008, 22, 164–168 CrossRef CAS.
- X. X. Mei, C. H. Guo, B. F. Liu, Y. Tang and D. F. Xing, RSC Adv., 2015, 5, 78136–78141 RSC.
- J. Wu, W. Chen, Y. Yan, K. Gao, C. Liao, Q. Li and X. Wang, Bioelectrochemistry, 2017, 117, 9–14 CrossRef CAS PubMed.
- K. Venkidusamy, M. Megharaj, M. Marzorati, R. Lockington and R. Naidu, Sci. Total Environ., 2016, 539, 61–69 CrossRef CAS PubMed.
- H. Wang and Z. J. Ren, Biotechnol. Adv., 2013, 31, 1796–1807 CrossRef CAS PubMed.
- D. R. Lovley and N. S. Malvankar, Environ. Microbiol., 2015, 17, 2209–2215 CrossRef CAS PubMed.
- S. K. Chaudhuri and D. R. Lovley, Nat. Biotechnol., 2003, 21, 1229–1232 CrossRef CAS PubMed.
- B. Logan, S. Cheng, V. Watson and G. Estadt, Environ. Sci. Technol., 2007, 41, 3341–3346 CrossRef CAS PubMed.
- Y. Hindatu, M. S. M. Annuar and A. M. Gumel, Renewable Sustainable Energy Rev., 2017, 73, 236–248 CrossRef CAS.
- S. A. Cheng and B. E. Logan, Electrochem. Commun., 2007, 9, 492–496 CrossRef.
- N. W. Zhu, X. Chen, T. Zhang, P. X. Wu, P. Li and J. H. Wu, Bioresour. Technol., 2011, 102, 422–426 CrossRef CAS PubMed.
- J. Zhang, J. Li, D. D. Ye, X. Zhu, Q. Liao and B. A. Zhang, Int. J. Hydrogen Energy, 2014, 39, 19148–19155 CrossRef CAS.
- P. Liang, H. Y. Wang, X. Xia, X. Huang, Y. H. Mo, X. X. Cao and M. Z. Fan, Biosens. Bioelectron., 2011, 26, 3000–3004 CrossRef CAS PubMed.
- Y. Z. Zhang, G. Q. Mo, X. W. Li, W. D. Zhang, J. Q. Zhang, J. S. Ye, X. D. Huang and C. Z. Yu, J. Power Sources, 2011, 196, 5402–5407 CrossRef CAS.
- L. Rago, N. Monpart, P. Cortes, J. A. Baeza and A. Guisasola, Water Sci. Technol., 2016, 73, 1129–1135 CAS.
- X. Wang, Y. J. Feng, J. Liu, H. Lee and N. Q. Ren, J. Chem. Technol. Biotechnol., 2011, 86, 590–594 CrossRef CAS.
- X. J. Li, Y. Li, X. Zhao, L. Weng and Y. Li, J. Soils Sediments, 2017, 18, 1003–1008 CrossRef.
- S. Oh, B. Min and B. E. Logan, Environ. Sci. Technol., 2004, 38, 4900–4904 CrossRef CAS PubMed.
- S. Xia, J. Li and R. Wang, Ecol. Eng., 2008, 32, 256–262 CrossRef.
- O. Adelaja, T. Keshavarz and G. Kyazze, Int. Biodeterior. Biodegrad., 2017, 116, 91–103 CrossRef CAS.
- J. M. Morris, S. Jin, B. Crimi and A. Pruden, Chem. Eng. J., 2009, 146, 161–167 CrossRef CAS.
- J. Y. Lee, N. T. Phung, I. S. Chang, B. H. Kim and H. C. Sung, FEMS Microbiol. Lett., 2003, 223, 185–191 CrossRef CAS PubMed.
- B. E. Logan, C. Murano, K. Scott, N. D. Gray and I. M. Head, Water Res., 2005, 39, 942–952 CrossRef CAS PubMed.
- S. Jung and J. M. Regan, Appl. Microbiol. Biotechnol., 2007, 77, 393–402 CrossRef CAS PubMed.
- J. R. Kim, S. H. Jung, J. M. Regan and B. E. Logan, Bioresour. Technol., 2007, 98, 2568–2577 CrossRef CAS PubMed.
- H. Rismani-Yazdi, A. D. Christy, B. A. Dehority, M. Morrison, Z. Yu and O. H. Tuovinen, Biotechnol. Bioeng., 2007, 97, 1398–1407 CrossRef CAS PubMed.
- P. T. Ha, B. Tae and I. S. Chang, Energy Fuels, 2008, 22, 164–168 CrossRef CAS.
- D. A. de Carcer, T. H. Phuc, J. K. Jang and I. S. Chang, Appl. Microbiol. Biotechnol., 2011, 89, 605–612 CrossRef CAS PubMed.
- N. J. Eyiuche, S. Asakawa, T. Yamashita, A. Ikeguchi, Y. Kitamura and H. Yokoyama, BMC Microbiol., 2017, 17, 145 CrossRef PubMed.
|
This journal is © The Royal Society of Chemistry 2019 |
Click here to see how this site uses Cookies. View our privacy policy here.