DOI:
10.1039/C9RA03343B
(Paper)
RSC Adv., 2019,
9, 26224-26229
Structure confirmation and thermal kinetics of the inclusion of cis-jasmone in β-cyclodextrin
Received
5th May 2019
, Accepted 1st August 2019
First published on 21st August 2019
Abstract
In this study, inclusion complex of cis-jasmone in β-CD (β-CD-CJ) was synthesized to improve cis-jasmone stability. The structure and thermal kinetics of the inclusion complex was investigated by Fourier transform infrared spectroscopy (FTIR), thermogravimetric analysis (TG) and differential scanning calorimetry (DSC). DSC studies showed that the stability of cis-jasmone after β-cyclodextrin encapsulation was improved. The dissociation kinetics of β-CD-CJ at different heating rates was studied by TG, and the activation energy E of β-CD-CJ thermal decomposition kinetic parameters was defined by Flynn–Wall–Ozawa method. The results showed that the average activation energy E was 121.16 kJ mol−1.
1. Introduction
β-Cyclodextrin (β-CD) is a cone-shaped cavity polymer consisting of seven glucopyranose units linked by α-(1,4) bonds.1 The cone-shaped cavity of β-CD allows the accommodation of the different classes of molecules to form inclusion complexes for the purpose of increasing solubility and stability.2–7 The inclusion complexes are constituted by a host molecule and a variety of guest molecules. In addition to slight deformation, the size and shape of the available cavity of the host molecules and the structural features of the guest molecules are almost unchanged.8 The capability of cyclodextrins for complex formation depends on the guest molecule's size, which should fit inside the cavity of cyclodextrin, and on thermodynamic interactions between cyclodextrins guest molecule, and the solvent.9 Therefore β-CD is typically used as a host molecule encapsulating agent for food additives, flavorings, vitamins and pharmaceutical development.8,10 After being encapsulated by β-CD, the physical, chemical and biological properties of molecules may be modified. For instance, β-CD acts as a flavor sealant to protect against oxidation, or reduce unwanted taste.11 Inclusion complex of trans-cinnamaldehyde in β-CD is blended with chitosan to treat fresh-cut melon, which can effectively maintain the hardness and color of melon, reduce weight loss and prolong shelf life.12 Inclusion complexes between drug and (β-CD) can increase the apparent aqueous solubility and enhance bioavailability of the drug.13 Carvalho et al. used two different techniques to prepare inclusion complexes between FZD and β-cyclodextrin (β-CD), and physicochemical analysis revealed the inclusion complexes could be used in the pharmacotherapy of leishmaniasis in dogs infected with L. amazonensis.14 What's more, β-CD is a safe natural inclusion material.15,16 The inclusion complex of cyclodextrin and perfume molecules can improve the water solubility of perfume molecules, increase the stability of perfume molecules, and improve the availability of perfume molecules. Extend the fragrance period and improve the quality of the product.17 Cis-jasmone is widely available in jasmine, orange blossom, bergamot, sea tong, kiwi, honeysuckle, magnolia, lotus root, and tobacco.18–21 It is repellent to aphids and reduces secondary metabolites of pest's development.22–24 Moreover, cis-jasmone is the most important contribution to the scents of perfumes and cosmetics.25 Therefore, cis-jasmone has received more and more attention. However, the scent of the cis-jasmone is extremely volatile and their fragrance is easily lost. The instability limits the application of cis-jasmone. The complexity of cis-jasmone in β-CD (β-CD-CJ) was rarely reported in the literature before, let alone the thermal dissociation kinetics of them. The aim of this study was to prepare and characterize β-CD-CJ, providing a way to increase the stability of the cis-jasmone. In this work, β-CD-CJ was prepared under controlled experimental conditions.26 Then the inclusion complex was characterized by FTIR. Finally, the thermal dissociation kinetics of the inclusion complex was evaluated.
2. Materials and methods
2.1. Materials
Cis-jasmone, 97%, was purchased from Henan Xinzheng Gold Leaf Spice Co., Ltd (China) and was without further purification. β-Cyclodextrin was purchased from Tianjin Kermel Chemical Reagent Co., Ltd (China).
2.2. Preparation of β-CD-CJ
β-CD-CJ was prepared by adding the cis-jasmone to a saturated β-CD solution in a molar ratio (β-CD: cis-jasmone) of 1
:
2, at 60 °C for 6 h, then slowly reduced the temperature of the reaction to 4 °C. After 24 h, the reaction solution was filtered, then the solid was washed with distilled water and ethanol, respectively. Afterward the solid was dried under vacuum and stored in an airtight glass vessel. This method resembles that previously described in literature.27
2.3. Methods
The samples (β-CD, cis-jasmone, β-CD-CJ, a mixture of β-CD and cis-jasmone (molar ratio 1
:
1)) were analyzed by FTIR (Nicolet iS50), samples and spectroscopic-grade KBr were compressed into a 1 mm wafer. For each sample scanning range was from 4000 to 400 cm−1 and a resolution of 4 cm−1.
Thermal analysis was carried out using a simultaneous thermal analyzer (NETZSCH STA 449 F3, Germany). Spectroscopic pure Al2O3 was taken as a reference substance, and Ar2 was used as protective gas (flow rate 60 mL min−1) and purge gas (flow rate 20 mL min−1). Thermal degradation temperature ranged from 30 to 900 °C. The mass of each sample used for DSC and TG analysis was approximately 10 mg. The heating rate of the precursor materials (β-CD, cis-jasmone), a mixture of β-CD and cis-jasmone (MCJ) and β-CD-CJ were 10 K min−1. Furthermore, two heating rates, 5 K min−1 (Φ1) and 20 K min−1 (Φ3), have been used to measure thermogravimetric of β-CD-CJ.
3. Results and discussion
3.1. Structure identification
The infrared characterization curves of the samples are illustrated in Fig. 1 curves (a), (b), (c) and (d) are infrared characteristic peaks of β-CD, cis-jasmone, MCJ and β-CD-CJ, respectively. Among the Fig. 1, it is clear that the infrared characteristic peaks of cis-jasmone and β-CD can be found in the curve (c), indicating that MCJ is just a simple mixture of β-CD and cis-jasmone. Compared with the curve (c), the number of peaks shown in the curve (d) is reduced, the peak strength is weakened, and the shape of the peak becomes wider. Moreover, the characteristic peak of cis-jasmone carbonyl group had an obviously decrease in the intensity of the peak at 1698 cm−1 and 1645 cm−1, 1698 cm−1 for C
O stretching vibration this data is similar to that previously described in literature,28 1645 cm−1 for C
C stretching vibration, indicating that these part of the carbonyl group and carbon–carbon double bond are likely to be inserted into the cone-shaped cavity of β-CD.
 |
| Fig. 1 FTIR for: (a) β-CD; (b) cis-jasmone; (c) MCJ; (d) β-CD-CJ. | |
3.2. Thermal analysis
3.2.1. TG analysis. The TG and DTG curves of samples for the heating rate of 10 K min−1 are indicated from Fig. 2–5. The curves profile of Fig. 2 suggests that the process of thermal decomposition of cis-jasmone occurred apparently in two steps. From 30 to 200 °C, cis-jasmone exhibited a sharp decrease with a mass loss of 97.33%. The second stage exhibited a slow mass loss process with mass loss of 2.15%. Within the temperature of 30–200 °C, the DTG profile of cis-jasmone exhibited two peaks temperature (Tp,1 = 135 °C, Tp,2 = 175.5 °C).
 |
| Fig. 2 TG and DTG curves of cis-jasmone. | |
 |
| Fig. 3 TG and DTG curves of β-CD. | |
 |
| Fig. 4 TG and DTG curves of MCJ. | |
 |
| Fig. 5 TG and DTG curves of β-CD-CJ. | |
The relationship between temperature change and mass loss of β-CD is illustrated in Fig. 3. It revealed that three stages of the thermal degradation of β-CD can be observed, with temperature increasing. The first stage occurred in the temperature range of 30 to 300 °C, and the mass loss of β-CD was about 23.09%. At this stage, there was one peak temperature on the top of the DTG curve (Tp,1 = 100 °C). The second stage mass loss started at 300 °C and ended at 375 °C. This was the main mass loss stage as indicated and total mass loss was 74.6%. The DTG profile of β-CD depicted one obvious peak temperature (Tp,2 = 322.5 °C). From 375 °C to 900 °C, the TG curve became flat, which showed the residual carbonaceous material of the former steps.
The MCJ had undergone four major mass loss stages was presented in Fig. 4. From 30 to 345 °C, MCJ had three consecutive mass loss processes, with a total mass loss of 74.6%. During this process, the DTG curve of MCJ shows two evident peak temperatures (Tp,1 = 195 °C, Tp,2 = 325 °C).
The TG and DTG curves of the β-CD-CJ were shown in Fig. 5. As can be seen from the TG curve, there were three stages of thermal decomposition, the first step of decomposition occurred from 30 to 227.5 °C, there was almost no loss of mass, meaning that β-CD-CJ was almost not decomposed. The second mass loss step began at 227.5 and ended at 360 °C, the TG curves depict a very sharp decrease loss process with mass loss of 76.3% of original mass. In this stage, the DTG curve of β-CD-CJ had only one peak temperature (Tp,1 = 327 °C). After 360 °C, TG curve became stable and the mass loss was about 4.1% of the original mass.
The results indicated that the thermal stability of cis-jasmone was improved after encapsulation, because the decomposition reaction of β-CD-CJ began at 227.5 °C, in contrast to cis-jasmone which thermal decomposition started at 30 °C. It was observed that the mass loss of decomposition of β-CD-CJ (76.3%) was lower than that of cis-jasmone (97.33%), indicating the inclusion effect was effective.
3.2.2. DSC analysis. The results shown in the TG, DTG and DSC curves coincide with each other. The onset temperatures and peak temperatures of the samples were recorded by DSC curves. Fig. 6 and 7 show that the onset temperatures of thermal decomposition of cis-jasmone and β-CD were 137.5 °C, 90.8 °C, and the Tpeak of cis-jasmone and β-CD measured by DSC was 185 and 297.7 °C, respectively. The DSC results for MCJ and β-CD-CJ are shown in Fig. 8 and 9, respectively. DSC measured the thermal decomposition onset temperature of MCJ was 103.5 °C, and the Tpeaks were 198.6 and 280.2 °C, respectively. However, β-CD-CJ has only one Tpeak temperature of 307.1 °C. This further demonstrated that β-CD-CJ was not a mixture of cis-jasmone and β-CD, but an inclusion complex of cis-jasmone in β-CD.
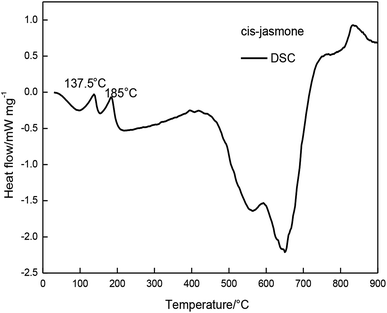 |
| Fig. 6 DSC curve of cis-jasmone. | |
 |
| Fig. 7 DSC curve of β-CD. | |
 |
| Fig. 8 DSC curve of MCJ. | |
 |
| Fig. 9 DSC curve of β-CD-CJ. | |
3.3. Kinetic analyses
The TG curves of thermal decomposition of β-CD-CJ with various heating rates were shown in Fig. 10. That can be seen from the figure, with the heating rate increasing, the TG curve moves to the right. The reason for it because the different heating rates affects mass loss rates of the β-CD-CJ, as well as high heating rates effectively facilitates the thermal decomposition reaction of the inclusion complex.
 |
| Fig. 10 TG curves of β-CD-CJ in different heating rate. | |
The simple thermal decomposition reaction equation24 is as follows:
|
ln ln(1/(1 − a)) = −E/RT + b
| (1) |
When the reaction order is 1, the plot of ln
ln(1/(1 − a)) against 1/T is a straight line. When the reaction order is less than 1, the lower half of the straight line is shifted to the left, otherwise, it moves to the right.
The kinetics of any solid-state decomposition reaction can be defined by the Flynn–Wall–Ozawa method29,30 as shown in the following:
|
log Φ = log(−AE/Rf(a)) − 2.315 − 0.4567(E/RT)
| (2) |
When n = 1, eqn (2) can be converted into the following form:
|
log Φ = log(−AE/R![[thin space (1/6-em)]](https://www.rsc.org/images/entities/char_2009.gif) ln(1 − a)) − 2.315 − 0.4567(E/RT)
| (3) |
where
b is a constant,
T is temperature (K),
a is mass loss rate,
E is activation energy (J mol
−1),
R is gas constant (8134 J mol
−1 K
−1) and
Φ is the heating rate (K min
−1),
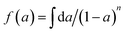
, (
n is the number of reaction order). If the plot of ln
Φ against 1/
T is linear, the activation energy
E and pre-exponential factor
A can be calculated from the slope and intercept, respectively.
Fig. 11 is obtained according to eqn (1). As shown, ln
ln(1/(1 − a)) and 1/T exhibited straight lines relationship, indicating the thermal decomposition of β-CD-CJ is a first-order reaction.
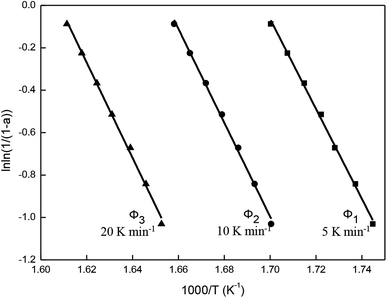 |
| Fig. 11 The relation of [ln ln(1/(1 − a))] and [1/T] in different heating rate. | |
The values of temperatures (T) at the same mass loss (a) on three TG curves of the β-CD-CJ are summarized in Table 1. By substituting the values of Φ and T in Table 1 into eqn (3), the slope and the intercept at the different mass loss rate were achieved by the linear least squares method with [log
Φ] versus [1/T]. The results received were presented in the Fig. 12 and the Table 2. Judging from the results of Table 2, the average activation energy of β-CD-CJ was 121.16 kJ mol−1. The lower activation energy value indicated that there was no strong chemical bond between β-CD and the guest molecule cis-jasmone. The host molecule and the guest molecule were mainly based on van der Waals force.31,32
Table 1 Temperatures corresponding to the same mass loss in different heating rate
a |
T (K) |
5 (K min−1) |
10 (K min−1) |
20 (K min−1) |
0.30 |
573.15 |
588.1 |
605.1 |
0.35 |
575.65 |
590.6 |
607.6 |
0.40 |
578.65 |
593.1 |
610.1 |
0.45 |
580.65 |
595.6 |
613.1 |
0.50 |
583.15 |
598.1 |
615.6 |
0.55 |
585.65 |
600.6 |
618.1 |
0.60 |
588.15 |
603.1 |
620.6 |
 |
| Fig. 12 Plots of the kinetics analysis based on the Ozawa method. | |
Table 2 The values of the kinetic parameters computed by the Flynn–Wall–Ozawa method
Sample |
a |
Slope |
Intercept |
E (kJ mol−1) |
A |
β-CD-CJ |
0.30 |
−6.53228 |
12.09999 |
118.89 |
6.48 × 1012 |
0.35 |
−6.58786 |
12.14706 |
119.90 |
8.65 × 1012 |
0.40 |
−6.75153 |
12.37249 |
122.88 |
1.68 × 1013 |
0.45 |
−6.59913 |
12.06948 |
120.10 |
1.00 × 1013 |
0.50 |
−6.65454 |
12.11579 |
121.11 |
1.28 × 1013 |
0.55 |
−6.71019 |
12.1621 |
122.13 |
1.63 × 1013 |
0.60 |
−6.76606 |
12.20841 |
123.14 |
2.06 × 1013 |
Average activation energy E (kJ mol−1) |
121.16 |
|
4. Conclusion
In FTIR analysis, the structure of the inclusion complex was monitored by the changes of the carbonyl indexes which indicated the structural characteristics of cis-jasmone after encapsulation. The results showed that the carbonyl peak intensity of the inclusion complex was significantly lower than the carbonyl peak intensity of cis-jasmone.
TG and DTG curves showed that the mass loss of the β-CD-CJ was less than cis-jasmone. DSC curves demonstrated that the β-CD-CJ has only one Tpeak temperature of 307.1 °C.
The results of the kinetic analysis indicated that the thermal decomposition of β-CD-CJ was dominated by a first-order thermal decomposition reaction process. The average activation energy for the process was 121.16 kJ mol−1. The average activation energy in the range of 40 kJ mol−1 to 400 kJ mol−1 belongs to chemical reaction control process.33
In a nutshell, cis-jasmone could form a stable inclusion complex with β-CD was revealed by FTIR, TG/DTG and DSC, and the thermal stability of cis-jasmone was improved by encapsulation, which provided some theoretical basis for the application of cis-jasmone.
Conflicts of interest
There are no conflicts to declare.
Acknowledgements
This work was supported by Ministry of Agriculture and Rural Affairs of the People's Republic of China (No. 2017YFD0200808); Hubei China Tobacco Industry Limited Liability Company (2018420000340440); Fujian Tobacco Company Longyan City Company (201835080027071).
References
- P. Dąbrowska and W. Boland, ChemBioChem, 2007, 8, 2281–2285 CrossRef PubMed.
- R. A. Rajewski and V. J. Stella, J. Pharm. Sci., 1996, 85, 1142–1169 CrossRef CAS PubMed.
- L. M. Pinto, L. F. Fraceto, M. H. Santana, T. A. Pertinhez, S. O. Junior and E. de Paula, J. Pharm. Biomed. Anal., 2005, 39, 956–963 CrossRef CAS PubMed.
- D. Duchêne, C. Vaution and F. Glomot, Drug Dev. Ind. Pharm., 1986, 12, 2193–2215 CrossRef.
- G. Dollo, D. O. Thompson, P. L. Corre, F. Chevanne and R. L. Verge, Int. J. Pharm., 1998, 164, 11–19 CrossRef CAS.
- S. L. Xin, W. H. Ming, Z. Z. Qiang and Q. Q. Liu, Bull. Chem. Soc. Jpn., 2007, 80, 2313–2322 CrossRef.
- W. Eli, W. Chen and Q. Xue, J. Inclusion Phenom. Macrocyclic Chem., 2000, 36, 439–445 CrossRef CAS.
- F. K. Heinz and J. Szejtli, Cyclodextrin Inclusion Complexes, 1988, pp. 79–185 Search PubMed.
- E. M. M. D. Vall, Process Biochem., 2004, 9, 1033–1046 CrossRef.
- V. M. Rao and V. J. Stella, J. Pharm. Sci., 2003, 92, 927–932 CrossRef CAS PubMed.
- J. S. Yu, F. D. Wei, W. Gao and C. C. Zhao, Spectrochim. Acta, Part A, 2002, 58, 249–256 CrossRef.
- J. N. Patel, D. M. Rathod, N. A. Patel and M. K. Modasiya, Int. J. Pharm. Life Sci., 2012, 2, 1459–1469 Search PubMed.
- S. P. Moreira, W. M. Carvalho and A. C. Alexandrino, Int. J. Food Sci. Technol., 2015, 10, 2192–2203 Search PubMed.
- S. G. Carvalho, L. A. Siqueira, M. S. Zanini and A. P. dos Santos Matos, Res. Vet. Sci., 2018, 119, 143–153 CrossRef CAS PubMed.
- S. Z. Zhang and X. H. Wang, Microbiology, 1988, 4, 65–66 Search PubMed.
- J. W. Lei, X. F. Wu and X. G. Cui, China J. Chin. Med., 2011, 1, 59–61 Search PubMed.
- B. Yang and G. Yang, Sci. Technol. Food Ind., 2007, 1, 210–212 Search PubMed.
- L. Ruzicka and M. Pfeiffer, Helv. Chim. Acta, 1933, 16, 1208–1214 CrossRef CAS.
- T. Koch, K. Bandemer and W. Boland, Helv. Chim. Acta, 1997, 80, 838–850 CrossRef CAS.
- J. B. Harborne, Phytochemistry, 1995, 39, 954 Search PubMed.
- J. T. Knudsen, L. Tollsten and L. G. Bergström, Phytochemistry, 1993, 33, 253–280 CrossRef CAS.
- M. A. Birkett, C. A. M. Campbell and K. Chamberlain, Proc. Natl. Acad. Sci. U. S. A., 2000, 97, 9329–9334 CrossRef CAS PubMed.
- T. J. A. Bruce, J. L. Martin, J. A. Pickett, B. J. Pye, L. E. Smart and L. J. Wadhams, Pest Manage. Sci., 2010, 59, 1031–1036 CrossRef PubMed.
- J. A. Pickett, M. A. Birkett and T. J. A. Bruce, Pestic. Outlook, 2005, 14, 96–98 Search PubMed.
- V. T. Karathanos, I. Mourtzinos, K. Yannakopoulou and N. K. Andrikopoulos, Food Chem., 2007, 101, 652–658 CrossRef CAS.
- M. M. Meier, M. T. B. Luiz, B. Szpoganicz and V. Soldi, Thermochim. Acta, 2001, 1, 153–160 CrossRef.
- G. P. Luo, M. R. Yan, H. N. Meng and C. Chen, China Food Addit., 2019, 30, 70–76 Search PubMed.
- J. Men, L. Wang, Y. H. Li, T. Li, M. Tang and F. C. Gu, Chem. Ind. Eng., 2006, 23, 215–219 CAS.
- Y. He and T. Sun, Food Sci., 2006, 2, 25–28 Search PubMed.
- T. Ozawa, Bull. Chem. Soc. Jpn., 1965, 38, 1881–1886 CrossRef CAS.
- S. J. Tian, G. X. Xi, Q. T. Cheng, X. D. Lou and H. J. Li, J. Therm. Anal. Calorim., 1998, 53, 825–833 CrossRef CAS.
- M. R. Caira, S. A Bourne and E. Mvula, J. Therm. Anal. Calorim., 1999, 56, 1329–1334 CrossRef CAS.
- G. Q. Hu, P. P. Wu, B. H. Xie and S. J. Zhang, J. Zhengzhou Univ., 2014, 35, 73–76 CAS.
|
This journal is © The Royal Society of Chemistry 2019 |