DOI:
10.1039/C9RA02247C
(Paper)
RSC Adv., 2019,
9, 17601-17611
Biocementation of soil by calcite/aragonite precipitation using Pseudomonas azotoformans and Citrobacter freundii derived enzymes†
Received
24th March 2019
, Accepted 28th May 2019
First published on 4th June 2019
Abstract
Microbial geotechnology is the use of microorganisms and/or their derivatives to alter engineering properties of soil for improving its stability, strength and stiffness. Ureases hydrolyze urea in the soil leading to CaCO3 precipitation, which binds soil particles together (biocementation). In the present study, nine Egyptian soils were screened for urease-producing bacteria, 15 isolates were obtained, and optimum urease producers were identified. Growth kinetics were measured at different pH values and in the presence of molasses as the sole carbon source. Citrobacter freundii and Pseudomonas azotoformans showed the highest extracellular urease activities of 45.5 ± 3.4 and 54.9 ± 3.5 U ml−1, respectively. Cell-free supernatants of these isolates mediated the precipitation of CaCO3 from the cementation solution (urea and CaCl2). The X-ray diffraction (XRD) of the precipitates revealed the formation of calcite and aragonite crystal forms. Sandy soil treated with the supernatants and evaluated by modified proctor and California bearing ratio (CBR) tests had significantly higher (P < 0.05) soil strength (CBR = ∼40% versus 30% for untreated soil). Scanning electron microscopy showed the CaCO3 precipitation resulting in reduction of the gaps between soil particles, hence confirming the biocementation phenomenon which is responsible for soil stabilization and the desired repairing effect on cracks. The use of urease-containing cell-free supernatant rather than the whole microorganism in biocementation lowers the risks of spreading pathogens to the environment and altering the microbial diversity at the application area.
1. Introduction
Roads are vital for economic development and improving lifestyles. Therefore, continuous efforts are exerted to find cheaper alternatives to the traditional road construction technologies. The need for soil replacement is a major hurdle facing road construction technologies nowadays which contributes greatly to the high road pavement cost. Soils with high bearing capacities can lower the construction cost considerably.1 This can be achieved by in situ treatment of the soil to improve its engineering properties, strength, stiffness and stability.1 Our recently published studies showed the potential and the applicability of enzymatic-based preparations in stabilizing the subgrade soil layer necessary in road construction.2
Microbial geotechnology is a recent discipline that considers the use of microorganisms and/or their derivatives for soil stabilization.1,3–6 Different approaches have been reported including biocementation (also known as biomineralization) and bioclogging.7 The former consists of microbial induction of calcite formation, which fills the pores between soil particles and bind them together,7–10 while the latter targets reducing the soil porosity and hydraulic activity by microbial production of pore filling materials such as polymers.6,7,10–13 Biocementation is considered a cheaper and more environment-friendly alternative to the traditional soil stabilization techniques which are done either chemically using lime, cement, epoxy and polyurethane or mechanically by compaction, in spite of their high cost, energy and time consumption.1,4,5,14–17 It also ensures high sustainability as stabilized roads by this technology can last for more than 50 years.18,19 Biocementation occurs by the action of different enzymes, the most common is through urease-associated hydrolysis of urea in the soil yielding carbonate and ammonia (eqn (1)). The resulting carbonate interacts with soil calcium forming the very slightly soluble calcium carbonate (eqn (2)).3–6,8,14,16
|
(NH2)2CO + 2H2O → CO32− + 2NH3
| (1) |
Biocementation has a wide range of applications in geotechnical engineering such as repairing cracks and concrete, treatment of cement surface, consolidation of sand, and filling the pores between soil particles for soil stabilization.11–13,20–22 Lately, its use in natural repairing of heritage stone artworks has been reported.23
However, although numerous studies have considered the use of the whole microorganism for in situ induction of calcite formation,6,9,24,25 only few considered the use of commercially available ureases.26,27 Cell-free enzyme solution can be advantageous since it lowers the risk of spreading pathogens to the environment or altering the microbial diversity at the application site.28,29
In the present study, different soils were screened for potential urease-producing bacteria. The isolates were characterized and identified, and their urease productivities were examined. The cell-free cultivation products were evaluated as soil stabilizers employing different analytical techniques such as scanning electron microscopy (SEM), X-ray diffraction (XRD), and the standard engineering tests; the modified proctor compaction test and California Bearing Ratio (CBR).
2. Materials and methods
2.1. Materials and culture media
Sodium acetate, urea, sodium chloride, potassium chloride, disodium hydrogen phosphate, potassium dihydrogen phosphate, sodium bicarbonate, phosphoric acid (85% w/v), sodium hydroxide, glucose, iodine, safranin, 95% ethanol and methanol were procured from El Nasr Pharmaceutical Chemicals Company (Cairo, Egypt), yeast extract from Difco (Detroit, MI, USA), and molasses from the local market. Phenol red, peptone, and agar were obtained from Fisher Scientific (Fair Lawn, NJ, USA), while beef extract and brain heart infusion (BHI) were obtained from LabM Limited (Heywood, Lancashire, UK). Crystal violet was purchased from Park Scientific Limited (Northampton, UK), Coomassie brilliant blue G-250 from Fluka Chemicals Ltd (Gillingham, UK), and bovine serum albumin from Spinreact (Girona, Spain).
2.2. Soil sampling
Nine different soils from different locations at Beni-Suef city, Egypt were sampled and used in the present study. The soil samples were designated as follows: A1, moist-deep sandy sample; B1, dry-sandy near wastewater treatment plant; B2, moist-muddy sample near the wastewater treatment plant; S1 and S2, sandy soils; while C, Y1, Y2 and Y3 were clay soils. Samples were directly stored at 4 °C and were dried in air just before use.
2.3. Bacterial isolation, maintenance and storage
Plastic cylindrical columns (12 cm length × 3 cm internal diameter) opened at the top and partially opened at the bottom were used for bacterial isolation. The columns were packed with 10 g of soil, covered with aluminium foil to protect from light and the lower opening was sealed.
For selective isolation of urease producing bacteria, urea was used as a substrate. Two enrichment solutions (ES) were prepared; ES1 containing per liter: 14 g sodium acetate, 20 g urea, 5 g molasses, and 0.5 g yeast extract, and ES2 containing the same components as ES1 but without molasses. All components were sterilized by autoclaving at 121 °C for 10 min except for urea which was filter-sterilized. ES1 was aseptically pipetted once at the top of each column, and after 3 days the columns were drained from the lower port then ES2 was pipetted once daily for 3 days.
After 48 h of incubation with ES2, 1 ml of the effluent from each column was collected in a sterile Falcon tube. The obtained solutions were spun down and the pellets were washed with 5 ml of phosphate buffer saline (PBS) containing per liter: 80 g NaCl, 2 g KCl 14.4 g Na2HPO4, and 2.4 g KH2PO (pH 7.4). The resulting pellets were resuspended in 5 ml of the PBS buffer and serially diluted. Finally, 100 μl of the serially diluted solution were aseptically spread on the surface of urea agar plates (5 g l−1 NaCl, 2 g l−1 KH2PO4, 1 g l−1 glucose, 20 g l−1 urea, 0.012 g l−1 phenol red, 0.2 g l−1 peptone, and 20 g l−1 agar, pH 6.8). The plates were incubated at 30 °C for 1–3 days. The resulting colonies that turned the urea-agar plates into pink were picked and streaked over nutrient agar (0.5 g l−1 peptone, 0.3 g l−1 beef extract and 1.5 g l−1 agar, pH 6.8) and incubated at 30 °C for 1–3 days.
For long-term storage, each bacterial isolate was transferred to 10 ml medium containing 5 g l−1 NaCl, 2 g l−1 KH2PO4, 1 g l−1 glucose, 20 g l−1 urea, and 0.2 g l−1 peptone, pH 6.8 and 10 ml BHI, respectively. The cultures were incubated in a shaking incubator at 30 °C and 160 rpm overnight. The resulting cultures were mixed with sterile glycerol to a final concentration of 20% v/v and stored at −80 °C.9 Sporosarsina pasteurii (DSM33) was obtained from DSMZ (Deutsche Sammlung von Mikroorganismen und Zellkulturen, Germany) and used as a reference strain.
2.4. Identification of the ureolytic bacterial isolates
2.4.1. Identification of the bacterial isolates by 16S rRNA gene sequencing. The DNA of the different pure isolates was extracted using ZR Fungal/Bacterial DNA MiniPrep (Zymo Research, Orange, CA, USA). The 16S rRNA genes were amplified by PCR using universal primers (IDT, San Jose, USA), 8F: 5′AGAGTTTGATCCTGGCTCAG3′,9,30 1525R: 5′ AAGGAGGTGATCCAGCC3′;9,31 785F: 5′GGATTAGATACCCTGGTA3′, 805R: 5′ GACTACCAGGGTATCTAATC3′; and 27F 5′ AGAGTTTGATCMTGGCTCAG 3′, 1492R: 5′GGTTACCTTGTTACGACTT3′.32The reaction mixture (25 μl) contained 12.5 μl MyTaq Red Master Mix (Bioline Reagents Ltd, London, UK), 1 μl forward primer (8 μM), 1 μl reverse primer (8 μM), 2 μl template DNA and 8.5 μl of sterile water. The PCR reaction was carried out using Primus 25 advanced® thermocycler (PEQLAB Biotechnologie GmbH, Erlangen, Germany) under the following conditions: initial denaturation at 95 °C for 3 min, followed by 30 cycles of denaturation at 95 °C for 30 s, primer annealing at 50 °C for 1 min and extension at 72 °C for 90 s, followed by final extension at 72 °C for 10 min. The amplicon was separated by gel electrophoresis (Labnet's ENDURO™, Edison, NJ, USA) using 1% (w/v) agarose gel, stained with ethidium bromide and visualized using UV trans-illuminator (Vilber Lourmat Deutschland GmbH, Eberhardzell, Germany) using hyperladder 1 kb (Bioline reagents Ltd, London, UK). The samples that showed a single clear amplification band on the agarose gel were purified from the reaction mixture using DNA Clean & Concentrator™-5 (Zymo Research, Orange, CA, USA) and the concentration of the purified amplicons were measured using Nanodrop 2000, Thermo Fisher Scientific (Waltham, MA, USA). Subsequently, the samples were sequenced in the forward and reverse directions (Macrogen Inc., Seoul, Republic of Korea). BioEdit (version 7.2.5) was used to edit the sequences and assemble the contigs. Sequences were aligned by ClustalW (DNA weight matrix IUB, Gap opening penalty 15, Gap extension penalty 6.66).
2.4.2. Colorimetric identification and characterization by VITEK technique. The isolates were identified using VITEK technique (VITEK 2 compact is an automated microbiology system for identification of different microorganisms using colorimetric reagent cards) at Animal Health Research Institute (AHRI) (Giza, Egypt). Each card has 64 wells, each contains a substrate that measures a specific metabolic activity.33
2.5. Determining the optimum pH for growth of ureolytic isolates
The effect of the pH on microbial growth was studied using 50 mM of three different buffers; K2HPO4/KH2PO4 (pH 6, 7 and 8), Tris–HCl (pH 9), and NaHCO3/NaOH (pH 10). The experiment was done in a Sunrise microtiter plate reader (Tecan Group Ltd., Männedorf, Switzerland) incubated at 30 °C. The program was set to measure the optical density at 600 nm (OD600 nm) at 30 min intervals for 22.5 h with intermittent shaking before each measurement (shaking duration 5 s, normal intensity).
The inoculum was prepared in 10 ml BHI broth medium inoculated with a single colony of the desired isolate and placed in a shaker incubator at 30 °C and 150 rpm overnight. The experiment was started by mixing 90 μl of the BHI with 5 μl of the freshly prepared inoculum and 10 μl of the suitable buffer to reach the desired pH in a microtiter plate.
2.6. Evaluation of molasses as substrate
Molasses (1% v/v) was evaluated as sole carbon source in the culture media. Molasses was added to the media containing per liter: 5 g NaCl, 2 g KH2PO4, 20 g urea, and 0.2 g peptone. The ability of the microorganism to grow on molasses was monitored for the different isolates using a microtiter plate reader incubated 30 °C.
2.7. Assay of extracellular urease activity
Each isolate was cultivated in 10 ml liquid broth in 50 ml Falcon tube containing per liter: 5 g NaCl, 2 g KH2PO4, 1 g glucose, 20 g urea, 0.2 g peptone (pH 6.8) and incubated at 30 °C and 160 rpm overnight. The culture was then centrifuged at 4 °C and 14
000 × g for 10 min using a cooling centrifuge 3-30K (Sigma, Shropshire, UK).
The urease activity was analyzed by reacting the ammonium ions liberated from hydrolysis of urea (Berthelot reaction) with sodium nitroprusside giving blue indophenol dye which can be measured colorimetrically between 530 nm and 570 nm.
The test was done by mixing 50 μl of the standard urease enzyme solution (10
000 U l−1) or the sample with 2.5 μl of urea solution (500 mg l−1) and incubating the mixture for 5 min at 37 °C. Subsequently, 250 μl of the coloring reagent (100 mmol l−1 phenol and 0.2 mmol l−1 sodium nitroprusside) and 250 μl of the alkaline reagent (150 mmol l−1 sodium hydroxide and 15 mmol l−1 sodium hypochlorite) were added, respectively. The mixture was mixed well and incubated for another 10 min at 37 °C. Finally, the absorbance was measured at 570 nm.34 The urease activity in the supernatant was estimated from the standard curve equation. The supernatant of Sp. pasteurii DSM33 was used as a positive control and the culture media was used as blank.
Isolates with optimal urease activities (six strains) were studied and compared with that of Sp. pasteurii DSM 33 as a control. The first inoculum prepared by adding 100 μl of each strain to 10 ml of BHI broth and then incubated at 30 °C in a shaker incubator overnight at 160 rpm. The resulting culture was used to inoculate 100 ml of BHI broth that was incubated at 30 °C in the shaker incubator overnight at 160 rpm and then was transferred to 1 l of cultivation broth. The cultivation media contained per liter: molasses 1% v/v, NaCl 5 g, KH2PO4 2 g, urea 20 g, peptone 0.2 g and was incubated at 30 °C and 160 rpm overnight. Nystatin 150 IU ml−1 and 25 μg ml−1 of chloramphenicol were added to prevent contamination. The cell-free supernatants were tested for urease activity and total protein concentration.
2.8. Evaluation of the geotechnological potential of the ureolytic isolates in soil stabilization
2.8.1. Calcium carbonate precipitate formation and composition. The test was done by adding the cell-free supernatant to cementation solution containing 500 mM urea (sterilized by filtration) and 500 mM CaCl2 (sterilized by autoclaving) to form a precipitate in a test tube. The precipitation level in each case was determined and compared. The precipitate was then dried and examined using XRD 202964 PANALYTICAL Empyrean (the Netherlands) with Cu anode (40 mA, 45 kV).
2.8.2. Particle size analysis of soils (sieve analysis). The dry sandy soil sample was weighed according to ASTM D 422-63.35 Each empty sieve and the bottom pan that were used in the analysis were also weighed. All sieves should be clean and ordered in ascending order (sieve 4 at the top and sieve 200 at the bottom). The bottom pan was placed below sieve 200. The soil sample was poured carefully into the top sieve and the cap was placed over it. The sieves stack was placed in the mechanical shaker and was shaken for 10 min. The sieves stack was then removed from the shaker and each sieve with its retained soil was weighed and recorded. As well, the bottom pan with its retained fine soil was weighed.
2.8.3. Soil treatment and examination by XRD and SEM. For each cell-free supernatant, 10 g of sand were put in a 50 ml Falcon tube and sterilized by autoclaving at 121 °C for 15 min. The supernatant and cementation solution were mixed 1
:
1 dropwise. The mixture was incubated at 30 °C overnight, and the soil samples were then dried at 50 °C. A typical sand soil without treatment was used as a negative control for the treatment. The treated soil samples were dried and examined by XRD as described earlier (Section 2.8.1) and SEM in Central Metallurgical Research Institute (CMRDI) Helwan, Egypt using FESEM Quanta FEG 250 (The Netherlands). SEM was used to examine the precipitation of calcium carbonate around the sandy soil particles.
2.8.4. Modified proctor test. Six liters of cell-free cultivation broth of strains DSM33, Y2b, and C, respectively, were prepared to test their stabilizing effect on sandy soil. The sand soil was premixed with cultivation broth then cementation solution was added. The compaction test was performed according to the standard procedure (ASTM D1557).36 Firstly, 5 layers of soil placed in a mould of known dimensions, and then 25 blows were applied on each layer using 10.00 lbf rammer dropped from 18.0 inch distance. Finally, the soil was subjected to a total compactive effort of about 56
000 ft-lbf ft−3. Briefly, the sandy soils were mixed with different weights of water using a standardized cylindrical mould; the samples were then subjected to compaction of controlled magnitude. The dry density of each sample was determined, and a graph was plotted showing the relation between the maximum dry density and the water content. From that curve, the optimal moisture content (γdmax) can be calculated.
2.8.5. California bearing ratio (CBR). A load of 4 kg at a rate of 1.25 mm min−1 using standard plunger was applied on each soil sample and penetration depth was determined.37 A graph between stress (load) in pounds applied on each soil sample and penetration depth in inch square was plotted. CBR is presented as a ratio since it relates the soil strength to a standard known for its high mechanical pressure i.e. limestone.
2.9. Statistical analysis
The presented data are the average of at least two independent replicates ± standard deviation. Significant differences among treatment means were tested by one-way ANOVA followed by the Student's t test to examine each pair of means using Microsoft Excel Analysis ToolPak Add-in, with a significance level of 0.05.
3. Results
3.1. Bacterial isolation and identification
Soil samples from different locations at Beni-Suef were screened for ureolytic bacteria. Fifteen isolates were recognized by their ability to change the color of the pH indicator in the culture medium to pink accompanied by a characteristic ammonia odor. The different isolates were examined for colony morphology on nutrient agar, microscopic cell morphology and Gram stain. Also, the 16S rRNA genes of the different isolates were amplified, sequenced, and the sequences were blasted against NCBI gene database. The most relevant strains were identified by percentage identity (Table 1). The 16S rRNA genes of the Type strains were obtained from EzBioCloud database to generate a phylogenetic tree. Phylogenetic analysis of the identified isolates was done by MEGA X 10.0.4 using neighbor joining statistical method. Test of phylogeny was done by Bootstrap method; the number of bootstrap replications was adjusted to 500 while evolutionary distances were calculated using the maximum composite likelihood method (Fig. 1).
Table 1 Identification of the ureolytic isolates by 16S rRNA gene sequencing, colony morphology, Gram stain and microscopical cell morphology
Code |
Isolate |
Location of isolation |
16S rRNA %identitya |
Isolate accessionb |
Colony morphology on nutrient agar |
Gram stain |
Cell morphology |
GenBank accession number of strains with high percentage identity to the 16S rRNA gene sequences. GenBank accession number of the deposited 16S rRNA sequences of the different isolates. |
A1 |
Bacillus cereus |
Moist-sandy deep sample |
99/KX694390.1 |
MH734761 |
Large creamy |
+ |
Rod |
B1 |
Uncultured bacterium |
Dry-sandy near waste water treatment |
95/HQ811465.1 |
|
Large creamy |
+ |
Rod |
B2 |
Enterococcus faecalis |
Moist-muddy sample near the water treatment plant |
96/KJ783396.1 |
MH734762 |
Medium mucoid, change color of Bile Esculin agar to black |
+ |
Cocci |
S1a |
Aerococcus urinaeequi |
Sandy soil from east of the Nile desert |
100/KF817696.1 |
MH734765 |
Small transparent |
+ |
Cocci |
S1b |
Bacillus nealsonii |
97/JQ579625.1 |
MH734766 |
Small white |
+ |
Rod |
S2a |
Uncultured bacterium |
91/KP843082.1 |
MH734767 |
Small white |
− |
Rod |
S2b |
Desemzia incerta |
98/KF924215.1 |
MH734768 |
Small transparent |
− |
Rod |
Y2a |
Bacillus thuringiensis |
By the Nile shore |
98/KP790042.1 |
MH734771 |
Medium creamy |
+ |
Rod |
Y2b |
Citrobacter freundii |
99/CP024673.1 |
MH734772 |
Small white |
− |
Coliform |
PO4 |
Bacillus cereus |
Clay soil from an agricultural land |
97/KT719668.1 |
MH734764 |
Medium creamy |
+ |
Rod |
C |
Pseudomonas azotoformans |
97/KU143978.1 |
MH734763 |
Small yellow, broth give green fluorescence on UV |
− |
Rod |
Y1a |
Bacillus thuringiensis |
99/KX301308.1 |
MH734769 |
Small white |
+ |
Rod |
Y1b |
Bacillus thuringiensis |
97/KR708902.1 |
MH734770 |
Medium yellow |
+ |
Rod |
Y3a |
Bacillus thuringiensis |
99/KR708902.1 |
MH734773 |
Medium creamy |
+ |
Rod |
Y3b |
Uncultured bacterium |
78/DQ816236.1 |
|
Small white |
+ |
Rod |
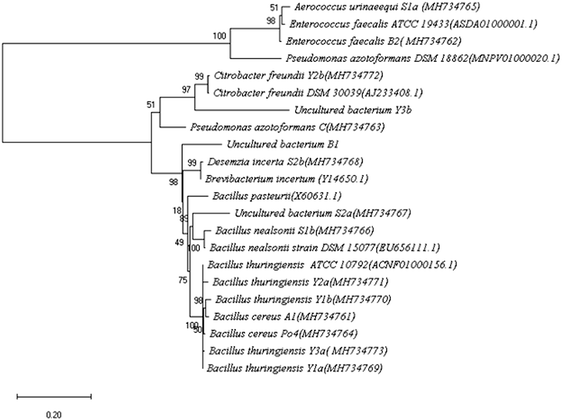 |
| Fig. 1 Phylogenetic analysis of the different bacterial isolates described in the current work based on the 16S-rRNA gene sequence relatedness using neighbor joining analysis. Numbers on the nodes represented the bootstrap values of 500 replicates. Scale bar below the tree indicates nucleotide substitutions per sites. | |
The isolates were also subjected to the automated biochemical identification technique; VITEK (ESI Tables 1–3†). The obtained results helped in confirming the identity for most of the pre-identified strains by 16S rRNA gene sequencing. Among the 15 bacterial isolates, almost 50% were identified as Bacillus. Others include Pseudomonas, Citrobacter, Enterococcus, Aerococcus, and Desemzia.
3.2. Optimum pH for growth and evaluation of molasses as C-source
The different isolates were grown at pH ranging from 6 to 10 (ESI Fig. 1†) and the maximum specific growth rate μmax was calculated from the plot of ln(OD) versus time. The optimum pH values for growth were extracted and summarized in Table 2. The different isolates showed different pH optima mainly around 6–7. However, Citrobacter freundii (Y2b) and strain A1 (identified as Bacillus cereus) showed pH optima of 8 and 9, respectively. Pseudomonas azotoformans showed the highest μmax of 0.39 h−1 at pH 6 (duplication time of 1.82 h). Its culture showed a distinctive blue fluorescence when exposed to UV light probably due to the production of the fluorescent pigment, pyoverdine (previously called fluorescein) (Yamamoto et al., 2000; Scales et al., 2014). Sugar cane molasses was evaluated as a potential C-source for microbial growth. All the strains showed clear ability to utilize molasses as main carbon source.
Table 2 The optimum pH for growth on Brain Heart Infusion medium (BHI) for the different isolates
Code |
Isolate |
Optimum pH |
A1 |
Bacillus cereus |
9 |
B1 |
Uncultured bacterium |
7–8 |
B2 |
Enterococcus faecalis |
7–8 |
S1a |
Aerococcus urinaeequi |
6 |
S1b |
Bacillus nealsonii |
6 |
S2a |
Uncultured bacterium |
6 |
S2b |
Desemzia incerta |
6, 7, 9 |
Y2a |
Bacillus thuringiensis |
7 |
Y2b |
Citrobacter freundii |
8 |
PO4 |
Bacillus cereus |
7 |
C |
Pseudomonas azotoformans |
6 |
Y1a |
Bacillus thuringiensis |
6 |
Y1b |
Bacillus thuringiensis |
7 |
Y3a |
Bacillus thuringiensis |
6 |
Y3b |
Uncultured bacterium |
7 |
3.3. Extracellular urease activities
The urease activity was measured colorimetrically in the cell-free supernatants. Among the 15 isolates, strains C, B2, S2b, Y2b, Y3a and Y3b showed remarkable extracellular urease activities (Table 3); which were significantly higher than that of the reference DSM33. C. freundii (Y2b) and Ps. azotoformans (C) had the highest activities of 45.4 ± 3.4 and 54.9 ± 3.5 U ml−1, respectively. Their activities were significantly higher (P < 0.05) than that obtained with the reference strain (13.6 ± 0.3 U ml−1) by 3–4 times. Therefore, the supernatants from these two isolates were used in the subsequent experiments using the reference strain as a positive control.
Table 3 Urease activity in cell-free culture supernatants
Sample |
Urease activity (U ml−1) |
Significant difference from the reference DSM33 (P < 0.05). Significant difference from the reference DSM33 (P < 0.1). |
DSM 33 |
13.6 ± 0.3 |
B2 |
24.0 ± 1.2a |
S2b |
5.5 ± 1.4a |
Y3a |
45.0 ± 7.3b |
Y3b |
39.8 ± 1.6a |
Y2b |
45.4 ± 3.4a |
C |
54.9 ± 3.5a |
3.4. Urease-mediated precipitation of CaCO3 from cementation solution
For testing the ability of using ureases in biocementation, cell-free supernatants from the different isolates were added to a cementation solution containing CaCl2 and urea and the precipitation of CaCO3 was observed. In all cases, a white precipitate was observed and increased gradually over time. The density of the precipitate was different for the different solutions (ESI Fig. 2†). For Sp. pasteurii the precipitate was compact and had a small packed volume (2 cm after 30 min and 0.5 cm after 5 days). On the other hand, Ps. azotoformans formed a fluffy precipitate with a bigger packed volume (4 cm after 30 min and 2 cm after 5 days), and C. freundii precipitate had an intermediate density and packed volume (3 cm after 30 min and 1 cm after 5 days).
Fig. 2 presents the XRD profile of the different precipitates. Two patterns were observed, the first belongs to calcite that is characterized by a prominent peak at 29.4 (2θ angle) (ESI Table 4A†) and was found in solutions treated with supernatant from reference strain (Sp. pasteurii DSM 33), while, aragonite with prominent peaks at 26.2 and 45.9 (2θ angel) (ESI Table 4B†) was observed in cementation solution treated with supernatants from C. freundii and Ps. azotoformans, respectively (Chu et al., 2013).
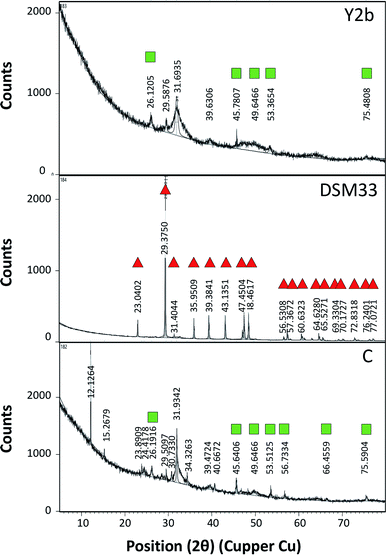 |
| Fig. 2 X-ray diffraction (XRD) profile of precipitate formed after mixing the cell-free supernatant obtained from C. freundii (Y2b), Ps. azotoformans (C) and the reference strain Sp. pasteurii (DSM33), respectively, with cementation solution. The peaks related to calcite is marked with red triangle while that related to aragonite is marked with green squares. | |
3.5. Stabilization of soil with cell-free supernatant
3.5.1. Soil characterization. Particle-size analysis (sieve analysis) of the test soil was done for determining particle size distribution, physical properties of untreated soil, and its classification according to the designation of the AASHTO system and is presented in Table 4 and ESI Fig. 3.†
Table 4 Particle size distribution and physical properties of untreated soil
Type of soil |
Sand |
(1) Gravel (%) of passing 3-in and retained on no. 4 sieve |
1.77 |
(2) Sand (%) of passing No.4 and retained on no. 200 sieve |
84.82 |
(a) Coarse sand, % passing no. 4 sieve and retained on no. 10 sieve |
2.76 |
(b) Medium sand, % passing no. 10 sieve and retained on no. 40 sieve |
24.62 |
(c) Fine sand, % passing no. 40 sieve and retained on no. 200 sieve |
57.44 |
(3) Silt size,0.074 to 0.005 mm |
8.90 |
(4) Clay size, smaller than 0.005 mm |
4.50 |
Coefficient of uniformity, cu |
7.00 |
Coefficient of curvature, cc |
1.85 |
Specific gravity Gs |
2.64 |
Liquid limit (LL) |
— |
Plastic limit (PL) |
— |
Plasticity index (PI) |
— |
Maximum dry density γdmax (ton m−3) |
1.92 |
O.M.C (%) |
9.70 |
pH |
8 |
Soil classification USCS |
Well graded sand |
AASHTO classification |
(A – 2–4) |
3.5.2. Modified proctor test. The proctor compaction test was performed for soils treated with the cell-free supernatant of Sp. pasteurii DSM 33, C. freundii and Ps. azotoformans. The obtained results showed slight decrease in the maximum dry density of the treated samples compared to the untreated soil. Optimum moisture and dry density results for the fine sand soil after being treated with the different supernatants, and differences in the maximum dry density (γdmax) between typical sand and treated soils are shown in Table 5.
Table 5 Optimum moisture content and dry density results of untreated sand and treated sand with three different bacterial growth supernatants, DSM33, Y2b and C
|
Typical sand |
DSM33 |
Y2b |
C |
Maximum dry density (γdmax) (ton m−3) |
1.92 |
1.86 |
1.88 |
1.87 |
Moisture content (%) |
9.70 |
11.80 |
10.80 |
10.00 |
3.5.3. California bearing ratio (CBR). The optimum moisture content for each sample is used in the determination of the CBR values for the fine sand soil after being treated with culture supernatant of Sp. pasteurii DSM 33, C. freundii and Ps. azotoformans. CBR tests were done to evaluate improvement in soil strength. The obtained results showed a significant improvement (P < 0.05) in the CBR values, where sandy soil treated with Sp. pasteurii DSM33 showed the highest improvement in un-soaked CBR value (49 ± 0.9%), compared to 42 ± 0.4% and 40 ± 1.0% for Ps. azotoformans and C. freundii samples, respectively, and 30 ± 0.3% for the untreated sand soil (Fig. 3).
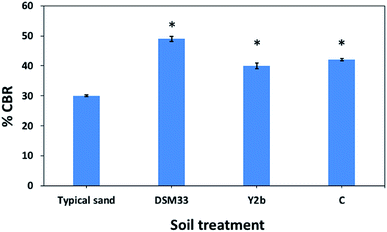 |
| Fig. 3 Percentage California bearing ration (%CBR) values for the fine sand soil and after being treated with culture supernatant of C. freundii (Y2b), Ps. azotoformans (C) and the reference strain Sp. pasteurii (DSM33). The (*) indicates a significant difference from the typical sand soil as well as from other treatments (P < 0.05). | |
3.5.4. XRD analysis. Soil (untreated and treated with cell-free supernatants) was analyzed by XRD. Fig. 4 presents the XRD profile of typical sand without any treatment with a prominent peak at 27.7 (2θ angle) characteristic for SiO2, and that for the sand treated with cell-free supernatants from DSM33 (positive control), C. freundii and Ps. azotoformans. Since the XRD profile includes peaks of sand and calcium carbonate crystals, the interference between the peaks of the two diagrams made it difficult to clearly identify the crystal form. Calcite formation is expected in soils treated with strains C. freundii and DSM33, while aragonite with a characteristic peak at 45.9 (2θ angle) is expected in soil treated with Ps. azotoformans.
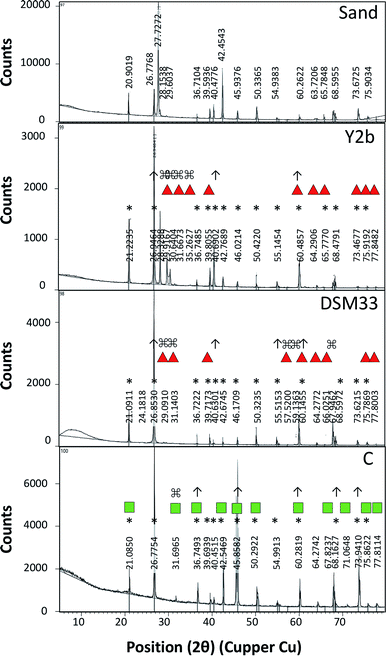 |
| Fig. 4 X-ray diffraction (XRD) profile of typical sand, and sandy soil treated with different urease-containing cell-free supernatant from C. freundii (Y2b), Ps. azotoformans (C) and the reference strain Sp. pasteurii (DSM33). The peaks related to calcite is marked with red triangle while that related to aragonite is marked with green squares. The peaks related to the untreated sand soil is marked with (*). The peaks of the treated soil that were increased in height is makred with (↑) while those that were absent in untreated sandy soil is marked with (⌘). | |
3.5.5. SEM analysis. SEM showed the precipitation of CaCO3 crystals on the surface and in between soil particles and revealed the resulting reduction in the gaps between soil particles after treatment (Fig. 5).
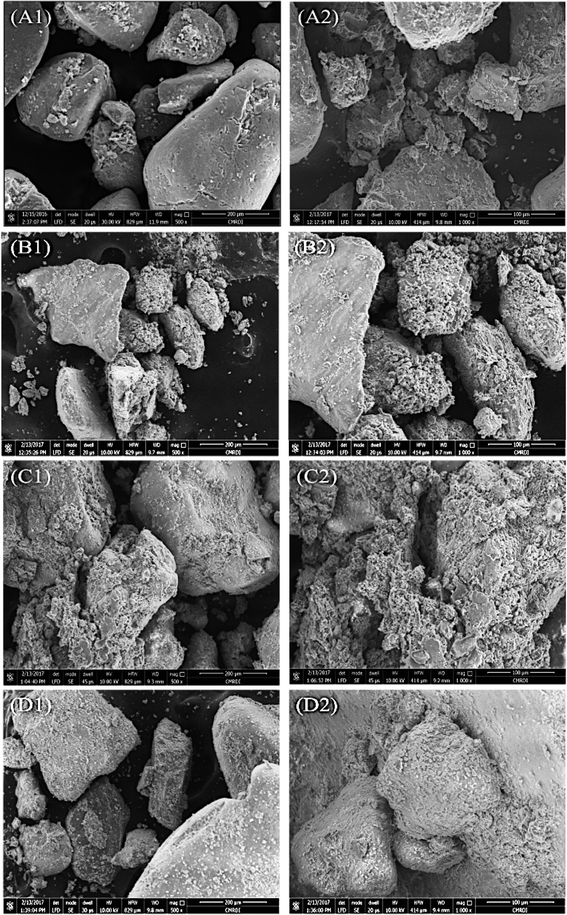 |
| Fig. 5 Scanning electron micrographs (1 = 500× magnification, 2 = 1000× magnification) of typical sandy soil (A1 and A2), and sandy soil treated with DSM33 broth supernatant (B1 and B2), C supernatant (C1 and C2) and Y2b supernatant (D1 and D2). The scale bar length is 200 μm in A1–D1, and is 100 μm in A2–D2. | |
4. Discussion
Urease producing microorganisms are important for biocementation in geotechnological applications. Therefore, different types of soil were screened for ureolytic bacteria. Soil samples were mainly collected from environmental niches where urea is expected to be present at high levels; sandy and muddy soils near wastewater treatment plant, and clay soil from agricultural land. Sequential enrichment steps using urea-rich media were applied to support the growth of urease-producers over others. From nine soil samples, 15 isolates were obtained. Phenotypic and genotypic characterizations were employed to identify the different isolates. Besides 16S rRNA gene sequencing, VITEK technique was used which recognizes the biochemical activities including excreted enzymes and ability to assimilate different sugars by the different isolates leading to their identification.
Among the different isolates, Ps. azotoformas and some Bacillus spp. have been reported earlier as potential microorganisms for soil stabilization.7,12,32,38 Ps. azotoformans and B. thurengeneisis are classified as safe microorganisms (risk group 1). The former is used in plant growth enhancement and soil stabilization by biocementation,12,39 while the latter is used as a bio- pesticide and insecticide.40,41 On the other hand, C. freundii; a denitrifying bacterium that is incorporated in nitrogen cycles and is used in bioremediation of nitrate and arsenic from contaminated water,42,43 as well as Enterococcus faecalis are considered opportunistic pathogens.40,44,45 Bacillus cereus is a pathogen causing food poisoning and gastrointestinal infection characterized by emesis and diarrhea.46,47
For field applications, microbial growth rate, culture media cost and extracellular urease activities are important factors to be considered. The effect of pH and cane molasses on microbial growth was investigated. Cane is the major source of sugar in Egypt and cane molasses is considered as a major secondary product. Most of the bacterial isolates were able to grow on molasses indicating that it can be a potential C-source. A number of commercially available soil stabilizing solutions contain molasses as a cheap component of the culture media for microbial growth and probably a thickening agent for soil stabilization.48,49 Molasses contains sucrose as the main carbon source plus small amounts of glucose and fructose resulted from sucrose hydrolysis during processing.50 The ability of the microorganism to grow on molasses has many advantages with regards to possible scaling up.
The extracellular urease activity of the different isolates was tested. C. freundii and Ps. azotoformans were the highest producers even significantly higher than that of the reference strain Sp. pasteurii. Bachmeier et al. 2002 reported that Sp. pasteurii produces intracellular urease with little amount excreted to the solution,51–53 which might explain the observed low activity of this strain in the cell-free supernatant.
The biocementation capacity of the cell-free supernatants was also investigated. XRD analysis was done to investigate the formation CaCO3 crystals and microstructural changes. Ureolytic bacteria can induce the formation of crystalline CaCO3 in three different polymorphic forms that can be identified by XRD i.e. calcite, vaterite and aragonite.54 The different forms have the same chemical formula but with different crystal structure. The XRD data of standard CaCO3 in the form of calcite (JCPDS no. 47-1743), vaterite (JCPDS no. 33-0268) and aragonite (JCPDS no. 41-1475) is shown in ESI Table 4 and Fig. 4.†
Zamarreno et al. (2009) showed that some Pseudomonas strains can induce precipitation of CaCO3 in two forms; calcite and vaterite.55 On the other hand, some strains of Acinetobacter were able to induce formation of vaterite more than calcite.55 Using the cell-free supernatants, calcite and aragonite were the main crystalline forms observed. The mechanisms of the formation of these forms are not fully understood, however, several reasons include biotic and abiotic factors might contribute to favoring of one type compared to the other.28,54,56,57 Calcite formation is favored under crystal aging conditions.58 The low extracellular urease activity of the reference strain compared to that of Ps. azotoformans and C. freundii might explain this. The solution pH also influences the type of crystals formed. Vaterite formation occurs at pH between 8.5 and 10 with the initial relative supersaturation between 6.5 and 8.5 in presence of high Ca2+ concentration and low temperature,59,60 while aragonite occurs at pH below 11 at low temperature.61
The studies that investigated engineering properties of urease-stabilized soils are scarce, especially in terms of using standard engineering protocols such as CBR test. This test is important to indicate how far the applicability of urease-stabilized soils can come to reality. It evaluates the mechanical strength of a given soil by measuring the pressure required for a plunger to penetrate the soil under specified parameters. The more resistant the soil, the higher the CBR; crushed limestone has the highest possible CBR value (100%). Moreover, modified proctor compaction test was used which is common for a given soil to determine the optimal moisture content at which the soil will be densest and achieve its maximum dry density. The obtained maximum dry density values for the treated soils were less compared to the control sample due to increase in the optimum moisture content. Increasing the optimum moisture content in the treated soil is always accompanied with reduction in the maximum dry density when the precipitate is fine particles – CaCO3 precipitate – since the specific gravity of the soil and additives is higher than that of water.62 The precipitation of calcium carbonate crystals between soil particles as seen by SEM confirms the biocementation phenomena of these supernatants and might be responsible for the desired repairing effect on cracks and soil stabilization.
5. Conclusion
C. freundii and Ps. azotoformans are potential microorganisms for applications in microbial geotechnology. The cultivation products obtained from the bacterial isolates had the ability to increase the bearing capacities of sand soils and possibly be applied for repairing building cracks. The use of only cultivation products rather than the microorganisms implies the ease and suitability for field applications, since there is no risk of spreading microorganisms or altering the microbial diversity. Such technique; after optimization can be used as a simple alternative for road pavement in villages that are not accessible for large mechanical road pavement tools. Comparing these solutions with others available commercially is highly recommended.
Author contributions
Y. Gaber is the PI of the research fund, proposed the research idea and participated in writing the paper. T. Dishisha designed the experiments, contributed in the analysis of the data and wrote the paper. H. Abdel-Aleem performed the experiments and contributed in writing of the manuscript. A. Saafan supervised H. Abdel-Aleem, contributed in the analysis of data. Abduallah A. AbouKhadra performed the proctor and CBR experiments and participated in writing. All authors revised the manuscript and approved its final form.
Conflicts of interest
The authors declare no conflict of interest.
Acknowledgements
Initiatives and National Campaigns, Academy of Scientific Research and Technology (ASRT), 101 Kasr El-Ainy St. Cairo, 11516, Egypt, is acknowledged for funding the project titled “Low Cost Technology for Roads Construction” Contract No. 24/2014 in collaboration with Beni-Suef University.
References
- L. Cheng, M. Shahin, R. Cord-Ruwisch, M. Addis, T. Hartanto and C. Elms, presented in part at 7th International Congress on Environmental Geotechnics: iceg2014, Australia, November, 2014 Search PubMed.
- A. AbouKhadra, A. F. Zidan and Y. Gaber, Cogent Engineering, 2018, 5, 1–11 CrossRef.
- V. S. Whiffin, PhD thesis, Murdoch University, 2004.
- L. A. Van Paassen, PhD thesis, Delft University of Technology, 2009.
- S. Fauriel and L. Laloui, Comput. Geotech., 2012, 46, 104–120 CrossRef.
- A. Al Qabany and K. Soga, Geotechnique, 2013, 63, 331 CrossRef.
- V. Ivanov and J. Chu, Rev. Environ. Sci. Bio/Technol., 2008, 7, 139–153 CrossRef CAS.
- V. S. Whiffin, L. A. van Paassen and M. P. Harkes, Geomicrobiol. J., 2007, 24, 417–423 CrossRef CAS.
- M. B. Burbank, T. J. Weaver, B. C. Williams and R. L. Crawford, Geomicrobiol. J., 2012, 29, 389–395 CrossRef CAS.
- Q. Zhao, L. Li, C. Li, M. Li, F. Amini and H. Zhang, J. Mater. Civ. Eng., 2014, 26, 04014094 CrossRef.
- W. S. Sidik, H. Canakci, I. H. Kilic and F. Celik, Geomech. Eng., 2014, 7, 649–663 CrossRef.
- S. H. Nonakaran, M. Pazhouhandeh, A. Keyvani, F. Z. Abdollahipour and A. Shirzad, World J. Microbiol. Biotechnol., 2015, 31, 1993–2001 CrossRef PubMed.
- L. S. Wong, J. Cleaner Prod., 2015, 93, 5–17 CrossRef CAS.
- L. Cheng and R. Cord-Ruwisch, Ecol. Eng., 2012, 42, 64–72 CrossRef.
- D. Kim, K. Park and D. Kim, Materials, 2013, 7, 143–156 CrossRef PubMed.
- K. Piriyakul and J. Iamchaturapatr, Adv. Mater. Res., 2013, 747, 660–663 Search PubMed.
- L. Cheng, M. Shahin and R. Cord-Ruwisch, Geotechnique, 2014, 64, 1010–1013 CrossRef.
- J. DeJong, K. Soga, E. Kavazanjian, S. Burns, L. Van Paassen, A. Al Qabany, A. Aydilek, S. Bang, M. Burbank and L. F. Caslake, Geotechnique, 2013, 63, 287 CrossRef.
- A. Gurbuz, Y. D. Sari and Z. N. Yuksekdag, Geomicrobiol. J., 2015, 32, 853–859 CrossRef CAS.
- V. Achal, A. Mukherjee, P. Basu and M. S. Reddy, J. Ind. Microbiol. Biotechnol., 2009, 36, 981–988 CrossRef CAS PubMed.
- V. Achal and X. Pan, Curr. Microbiol., 2011, 62, 894–902 CrossRef CAS PubMed.
- B. Martinez, J. DeJong, T. Ginn, B. Montoya, T. Barkouki, C. Hunt, B. Tanyu and D. Major, J. Geotech. Geoenviron. Eng., 2013, 139, 587–598 CrossRef CAS.
- F. Jroundi, M. Schiro, E. Ruiz-Agudo, K. Elert, I. Martín-Sánchez, M. T. González-Muñoz and C. Rodriguez-Navarro, Nat. Commun., 2017, 8, 279 CrossRef PubMed.
- G. Amarakoon and S. Kawasaki, J. Geol., 2016, 2016, 72–83 Search PubMed.
- G. Kim and H. Youn, Materials, 2016, 9, 468 CrossRef PubMed.
- H. Yasuhara, D. Neupane, K. Hayashi and M. Okamura, Soils Found., 2012, 52, 539–549 CrossRef.
- J. P. Carmona, P. J. V. Oliveira and L. J. Lemos, Procedia Eng., 2016, 143, 1301–1308 CrossRef CAS.
- W. De Muynck, N. De Belie and W. Verstraete, Ecol. Eng., 2010, 36, 118–136 CrossRef.
- J. Ettenauer, G. Piñar, K. Sterflinger, M. T. Gonzalez-Muñoz and F. Jroundi, Sci. Total Environ., 2011, 409, 5337–5352 CrossRef CAS PubMed.
- M. Hughes, G. James, N. Ball, M. Scally, R. Malik, D. Wigney, P. Martin, S. Chen, D. Mitchell and D. Love, J. Clin. Microbiol., 2000, 38, 953–959 CAS.
- D. J. Lane, B. Pace, G. J. Olsen, D. A. Stahl, M. L. Sogin and N. R. Pace, Proc. Natl. Acad. Sci. U.S.A., 1985, 82, 6955–6959 CrossRef CAS PubMed.
- A. Vahabi, A. A. Ramezanianpour, H. Sharafi, H. S. Zahiri, H. Vali and K. A. Noghabi, J. Basic Microbiol., 2015, 55, 105–111 CrossRef CAS PubMed.
- D. H. Pincus, in Encyclopedia of Rapid Microbial Methods, ed. M. J. Miller, Davis Healthcare International Publishing, LLC, River Grove, IL, USA, 2006, ch. 1, vol. 1, pp. 1–32 Search PubMed.
- M. Weatherburn, Anal. Chem., 1967, 39, 971–974 CrossRef CAS.
- ASTM D422-63, Annual Book of ASTM Standards, 2002, vol. 4 Search PubMed.
- ASTM D1557-12e1, ASTM International, West Conshohocken, PA, 2012 Search PubMed.
- ASTM, D1883-16, ASTM International, West Conshohocken, PA, 2016 Search PubMed.
- L. A. Van Paassen, C. M. Daza, M. Staal, D. Y. Sorokin, W. van der Zon and M. C. van Loosdrecht, Ecol. Eng., 2010, 36, 168–175 CrossRef.
- J. Levenfors, C. F. Welch, J. Fatehi, M. Wikstrom, S. Rasmussen and M. Hökeberg, US Pat., US8796179B2, 2014.
- M. Fernández-Ruvalcaba, G. Peña-Chora, A. Romo-Martínez, V. Hernández-Velázquez, A. B. del la Parra and D. P. de la Rosa, J. Insect Sci., 2010, 10, 186 CrossRef PubMed.
- R. C. Argôlo-Filho and L. L. Loguercio, Insects, 2013, 5, 62–91 CrossRef PubMed.
- J. Cole and C. Brown, FEMS Microbiol. Lett., 1980, 7, 65–72 CrossRef CAS.
- B. Li, X. Pan, D. Zhang, D.-J. Lee, F. A. Al-Misned and M. G. Mortuza, Ecol. Eng., 2015, 77, 196–201 CrossRef.
- B. E. Murray, Clin. Microbiol. Rev., 1990, 3, 46–65 CrossRef CAS PubMed.
- L.-H. Liu, N.-Y. Wang, A. Y.-J. Wu, C.-C. Lin, C.-M. Lee and C.-P. Liu, J. Microbiol., Immunol. Infect., 2017, 51, 565–572 CrossRef PubMed.
- A. Kotiranta, K. Lounatmaa and M. Haapasalo, Microbes Infect., 2000, 2, 189–198 CrossRef CAS PubMed.
- R. Kamar, M. Gohar, I. Jéhanno, A. Réjasse, M. Kallassy, D. Lereclus, V. Sanchis and N. Ramarao, J. Clin. Microbiol., 2013, 51, 320–323 CrossRef PubMed.
- S. Shirsavkar and S. Koranne, Electron. J. Geotech. Eng., 2010, 15, 1614–1624 CAS.
- M. Sravan and H. Nagaraj, presented in part at First International
Conference on Bio-Based Building Materials, Clermont-Ferrand, France, June, 2015 Search PubMed.
- W. W. Binkley and M. Wolform, in Advances in carbohydrate chemistry, Elsevier, 1953, vol. 8, pp. 291–314 Search PubMed.
- A. Kantzas, L. Stehmeier, D. Marentette, F. Ferris, K. Jha and F. Maurits, presented in part at Annual Technical Meeting, Alberta, Canada, June, 1992 Search PubMed.
- S. Stocks-Fischer, J. K. Galinat and S. S. Bang, Soil Biol. Biochem., 1999, 31, 1563–1571 CrossRef CAS.
- K. L. Bachmeier, A. E. Williams, J. R. Warmington and S. S. Bang, J. Biotechnol., 2002, 93, 171–181 CrossRef CAS PubMed.
- S. M. Al-Thawadi, J. Adv. Sci. Eng. Res., 2011, 1, 98–114 CAS.
- D. V. Zamarreno, R. Inkpen and E. May, Appl. Environ. Microbiol., 2009, 75, 5981–5990 CrossRef CAS PubMed.
- V. Ivanov, J. Chu and V. Stabnikov, in Biotechnologies and biomimetics for civil engineering, Springer, 2015, pp. 21–56 Search PubMed.
- D. Mujah, M. A. Shahin and L. Cheng, Geomicrobiol. J., 2017, 34, 524–537 CrossRef CAS.
- J. L. Wray and F. Daniels, J. Am. Chem. Soc., 1957, 79, 2031–2034 CrossRef CAS.
- D. Kralj, L. Brečević and A. E. Nielsen, J. Cryst. Growth, 1990, 104, 793–800 CrossRef CAS.
- H. Roques and A. Girou, Water Res., 1974, 8, 907–920 CrossRef CAS.
- C. Y. Tai and F. B. Chen, AIChE J., 1998, 44, 1790–1798 CrossRef CAS.
- N. Abbasi and M. Mahdieh, Int. J. Geo-Eng., 2018, 9, 4 CrossRef.
Footnote |
† Electronic supplementary information (ESI) available. See DOI: 10.1039/c9ra02247c |
|
This journal is © The Royal Society of Chemistry 2019 |
Click here to see how this site uses Cookies. View our privacy policy here.