DOI:
10.1039/C9RA01687B
(Paper)
RSC Adv., 2019,
9, 14060-14071
Heterogeneous activation of peroxymonosulfate for bisphenol AF degradation with BiOI0.5Cl0.5†
Received
6th March 2019
, Accepted 20th April 2019
First published on 7th May 2019
Abstract
This study represents the first investigation on the application of peroxymonosulfate (PMS) for the degradation of bisphenol AF (BPAF) using halogen bismuth oxide composites (BiOI0.5Cl0.5). The hierarchical BiOI0.5Cl0.5 was successfully synthesized and systematically characterized with multifarious techniques including XRD, SEM, FTIR and XPS to investigate the morphology and physicochemical properties of the samples. Several parameters affecting the degradation efficiency including catalyst dosage, PMS loading, and pH value were elucidated. Inorganic ions such as HCO3− showed significant inhibition in the BiOI0.5Cl0.5/PMS process due to the quenching effect. The effect of various water matrices including tap water and surface water on the removal of BPAF was studied to indicate that the present reaction system shows great potential for cleaning BPAF waste water. Furthermore, the production of sulfate radicals and hydroxyl radicals was validated through radical quenching and ESR tests, thus a possible oxidation mechanism was proposed. Overall, these results reveal that the activation of PMS by the BiOI0.5Cl0.5/PMS system is an efficient and promising advanced oxidation technology for the treatment of BPAF-contaminated waters and wastewaters.
1. Introduction
In recent years, bisphenols (BPA, BPAF and BPS) have been reported to be endocrine disruptor chemicals (EDCs), causing a variety of human health issues,1,2 which can imitate the estrogen of natural hormones to bring about various adverse effects on humans even at low exposure levels.3,4 BPA is a commonly used chemical with production estimated at above 4.5 million tons per year.5 Various methods have been proposed to remove BPA from wastewater nowadays, including adsorption,6 microbiological biodegradation,7 photocatalytic degradation8–10 and chemical oxidation.11,12 However, research on the degradation of its analogues is rare.
BPAF, an analogue of BPA, is being considered as a replacement for BPA. BPAF has been found in environmental water and solid samples, as well as in indoor dust, beverages, and food worldwide.13–15 BPAF has a similar chemical structure to BPA and is mainly used for professional elastomers synthesis cross linking and curing agent to improve their chemical and thermal properties. However, the introduction of fluorine improved the degradation resistance.16 Many studies showed that BPAF negatively affect some physiological processes in humans and animals,17 but the research on the degradation of it is rare, thus in the present study, BPAF was chosen as the target for degradation and removal.
Recently, advanced oxidation processes (AOPs) based on persulfate (PS) or peroxymonosulfate (PMS) have been attracted great attention. It has been confirmed that sulfate radicals (·SO4−˙) provide an effective way for removing reluctant organic compounds in the water,18,19 due to the following reasons: (1) ·SO4−˙ possesses a longer half-life than OH˙; (2) ·SO4−˙ radicals (E0 = 2.5–3.1 V) exhibit higher oxidation potentials than OH˙ radicals (E0 = 1.8–2.7 V) and (3) ·SO4−˙ radicals are more selective towards destruction of aromatic molecules.20,21 In recent years, lots of researchers have focused their attention on the oxone (potassium peroxymonosulfate, PMS), which is an eco-friendly and promising source to generate ·SO4−˙ radicals.22–27
On the other hand, bismuth oxyhalides (BiOX, X = Cl, Br and I) have been admitted as excellent adsorbents and photocatalysts for the removal of organic pollutants in the water.28–31 The band gaps of BiOX can be within 1.7–3.4 eV by varying the halides and the composition ratios of the two halides (BiOXαY1−α).32 Owing to the high separation rate of photo-induced electron–hole pairs, the past ten years have witnessed tremendous efforts in utilizing BiOCl as a promising catalyst for photocatalytic applications under visible light irradiation, including pollutants removal, N2 fixation, CO2 reduction and water splitting.33 However, most of the photocatalytic oxidation reaction based on BiOX usually needs several hours or more for pollutant removal. Therefore, it is necessary to promote technology combined with another antioxidant. To our best knowledge, bismuth oxyhalide has not been tested as an activator of peroxide for water purification. There is a handful of research has been reported that the interaction between the PMS and BiOX for organic wastewater treatment.34
Hence, in the present study, we represent the first investigation on the potential application of BiOI0.5Cl0.5 combined with PMS. We synthesized hierarchical BiOI0.5Cl0.5 using the solvothermal method. BPAF, which is commonly used in the chemical and textile industries were selected as typical targets. Multiple characterizations including XRD, SEM, FTIR, XPS and ESR were applied to evaluate the physicochemical performance of BiOI0.5Cl0.5 and reaction mechanism. In addition, several key parameters including catalyst dosage, PMS loading, the initial pH of BPAF solution and common anions were investigated. Besides, the realistic conditions were also investigated for the system using tap water and surface water conditions. Finally, a possible reaction mechanism was proposed based on the scavenger experiments.
2. Materials and methods
2.1 Materials and reagents
Bi(NO3)3·5H2O, KI and KCl were purchased from Kelong Chemical Reagent Co. (Chengdu, China). Bisphenol AF (BPAF) was purchased from Sigma-Aldrich Co., Ltd (Shanghai, China). Peroxymonosulfate (PMS) was obtained from Aladdin China. All other chemical reagents were analytical grade and used without further purification. The typical solutions used were prepared using Milli-Q water system (18.2 MΩ cm−1).
2.2 Synthesis of composites bismuth oxyhalides
The three catalysts were synthesized as the modified procedure reported by previous studies:29 8.731 g Bi(NO3)3·5H2O was dissolved in 180 mL ethylene glycol under intense stirring for 30 min to obtain solution A. Then, 2.989 g KI (for BiOI), 1.344 g KCl (for BiOCl) or 1.494 g KI/0.672 g KCl (for BiOI0.5Cl0.5) was dissolved in 180 mL water to obtain solution B, respectively. Afterwards, the solution B was added rapidly into the solution A under intense stirring for 2 h at the ambient temperature. The obtained mixture was calcined at 180 °C for 24 h in a stainless-steel autoclave equipped with a Teflon lining. Finally, the mixture was separated and washed three times respectively with ethanol and water, then dried at 60 °C in vacuum drying oven until use.
2.3 Experimental procedure
All experiments were carried out in a borosilicate glass beaker of 500 mL capacity at ambient temperature (25 °C ± 1 °C). A 200 mL reaction solution was prepared of BPAF solution at concentration of 10 mg L−1 without pH adjustment or with adjusting initial pH using 0.1 mol L−1 HCl and NaOH solution. In each test, a set of prepared BiOI0.5Cl0.5 was dispersed in the solution, all the suspensions were magnetically stirred for 10 min in the dark before adding a certain amount of PMS, in order to attain the adsorption–desorption equilibrium between the BiOI0.5Cl0.5 and the BPAF molecules. At given time intervals, aliquots of the suspension (about 1.5 mL) were sampled, filtered by a 0.22 μm filter before the concentration detection, quenched immediately by adding 60 μL 0.5 mol L−1 Na2S2O3 solution into the 2 mL auto sampler vials, its volume for PMS quench was 100
:
1. Before the time of zero, a pre-adsorption process for BPAF removal was accomplished.
The concentration of BPAF (λ = 270 nm) was detected by a Water e2695 HPLC equipped with UV-visible detection (Waters 2489) at the column temperature of 35 °C. And the mobile phase was maintained at a flow rate of 1.0 mL min−1 with a constant ratio (methanol/0.1% ammonium acetate = 70/30). The total organic carbon (TOC) of samples were measured by an Elementar liquid TOC II analyzer.
The degradation BPAF process approximately followed pseudo-first-order kinetics by data fitting, thus overall rate law for the BPAF degradation can be expressed as eqn (1):
|
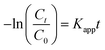 | (1) |
where the
C0 is initial concentration of BPAF,
Ct is the concentration of BPAF at time
t, and
Kapp (min
−1) is the observed reaction rate constant.
2.4 Characterization and analytical procedures
To determine the morphology of the as-prepared samples, scanning electron microscopy (SEM) measurements were run using a JSM-7500F (JEOL, Japan) at 20 kV. The crystal structural characterization of BiOI0.5Cl0.5 was conducted by powder X-ray diffraction spectrometry (XRD) using an Empyrean diffractometer advance instrument between 10 and 80° (2θ) (PANalytical B.V., Holland). Fourier Transform Infrared Spectrometer (FTIR) results were conducted using a Nicolet 6700 FTIR spectrometer (Thermo Scientific, United States), at the range of 400 to 4000 cm−1 wave numbers. The elemental composites and chemical states of BiOI0.5Cl0.5 were further elucidated with X-ray photoelectron spectroscopy (XPS) using a XSAM800 (Kratos, UK).
3. Results and discussion
3.1 Characterization of the synthesized samples
XRD was applied to characterize the chemical composition and crystalline structure of the synthesized BiOI0.5Cl0.5, the patterns of the samples are presented in Fig. 1. It is clearly that BiOX can be indexed to tetragonal phase structure BiOI (JCPDS card no. 73-2062, the cell parameters of a = 3.984 Å) and BiOCl (JCPDS card no. 73-2060, the cell parameters of a = 3.883 Å), indicating a high purity of the products. Moreover, the XRD peaks of the BiOI0.5Cl0.5 sample is weaker and broader than the pure BiOI and BiOCl, demonstrating a smaller particle size and lower crystallinity to some extent.35 The major peaks are positioned at 2θ = 11.1°, 25.8°, 29.9°, 32.0° and 32.6°. The shift of (001), (101), (102) and (110) planes are the strong evidence to determine the intercalated layer products, indicating that the as-prepared BiOI0.5Cl0.5 composite is not merely a mixture of BiOI and BiOCl.36
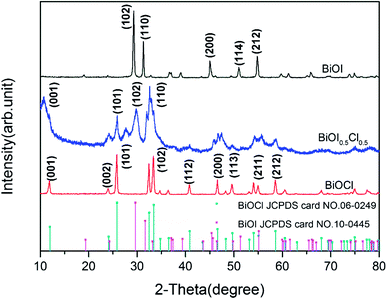 |
| Fig. 1 XRD patterns of BiOI, BiOCl and BiOI0.5Cl0.5. | |
The low- and high-magnification SEM images of synthesized BiOI0.5Cl0.5 are presented in Fig. 2. Obviously, the prepared BiOI0.5Cl0.5 samples are dominated with the hierarchical nanoplates.36 The nanoplate like structure of the catalysts is mainly seems to consists of quite uniform plates with width of about 100 nm and thickness of about 20 nm, these observations are in accordance with the reported literature.37 The formation of 2-dimension nanoplates structure can be ascribed to the internal structure of BiOI0.5Cl0.5, where [Bi2O2]2+ layers are interleaved by two slabs of X−˙ atoms, leading to the anisotropic growth at certain axis.38 In addition, the element mapping clearly illustrates the homogeneous distribution of O, Bi, I and Cl species in the prepared BiOI0.5Cl0.5 samples composite (as shown in Fig. S1†).
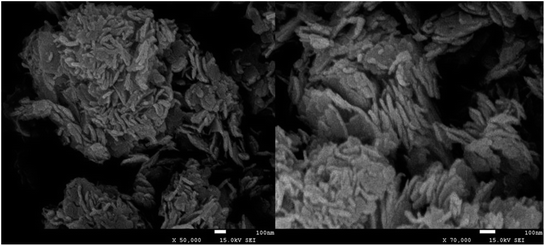 |
| Fig. 2 SEM images of synthesized BiOI0.5Cl0.5. | |
FT-IR spectra was applied to investigate and compare the functional groups on BiOI, BiOCl and BiOI0.5Cl0.5. As shown in Fig. 3, characteristic absorption peaks at 528, 1375 and 1650 cm−1 are observed in BiOI. The peak at 528 cm−1 is associated with the Bi–O stretching mode, while the absorption peaks at 1375 cm−1 could be assigned as the asymmetry and symmetric stretching vibration peaks for Bi–I band.39 Similar characteristic peaks emerge at 1116 cm−1 for Bi–Cl band in BiOCl structure. The band at 3435 cm−1 and 1628 cm−1 for BiOI0.5Cl0.5, BiOCl and BiOI are the stretching of O–H and flexural vibrations of O–H in the free water.40 No characteristic adsorption peaks of the ionic liquid are found in the FT-IR spectra, indicating that the ionic liquid can be easily removed from the surface of the synthesized BiOI0.5Cl0.5 by washing with alcohol and water. Besides, significant changes are observed between the fresh and used BiOI0.5Cl0.5 at 776 cm−1, 1082 cm−1 and 1305 cm−1 illustrated in Fig. 3, which might be formed by the adsorption of BPAF degradation products and other radicals. The peak at 1082 cm−1 corresponded to C–O–C stretching vibration,41 and the respective shift in 776 cm−1 and 1305 cm−1 peaks confirmed the interaction among BiOI0.5Cl0.5, PMS and BPAF, indicating that the degradation process takes place on the surface of the BiOI0.5Cl0.5.
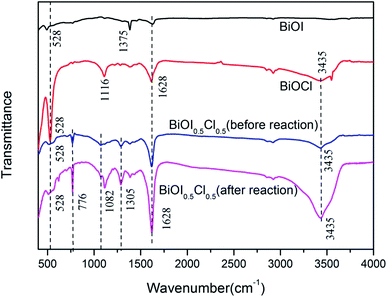 |
| Fig. 3 FTIR spectra of the synthesized BiOI0.5Cl0.5, BiOI and BiOCl. | |
XPS spectra is considered as an effective analysis to obtain further insight into the elemental composition and chemical states of the prepared samples. As shown in the Fig. 4(a), the coexistence of Bi 4f, I 3d, Cl 2p and O 1s peaks indicated the catalysts consisted of Bi, I, Cl and O elements. The carbon peak resulted from the adventitious carbon from atmosphere, which was used to correct the shift of the binding energy at 284.6 eV.42 There are two strong peaks at 158.9 eV and 164.3 eV (illustrated in Fig. 4(b)) contributing to Bi 4f7/2 and Bi 4f5/2, which demonstrates the presence of Bi–O bonding with trivalent oxidation state.43 Fig. 4(c) shows that the centered peaks at 617.8 eV and 629.4 eV were well corresponded to the inner electron of I 3d5/2 and I 3d3/2, respectively. The binding energies of 197.7 eV and 199.3 eV associated with Cl 2p3/2 and Cl 2p1/2 (illustrated in Fig. 4(d)), which showed the existence of Cl− in BiOI0.5Cl0.5.44 The O 1s peaks can be deconvoluted into two bands at 529.7 and 531.0 eV (illustrated in Fig. 4(e)), assigned to Bi–O and I–O bands.45,46 What's more, the peaks of O 1s change to higher binding energy value and I 3d change to lower binding energy in the catalyst after reaction, suggesting that the degradation process takes place on the surface of the BiOI0.5Cl0.5.47
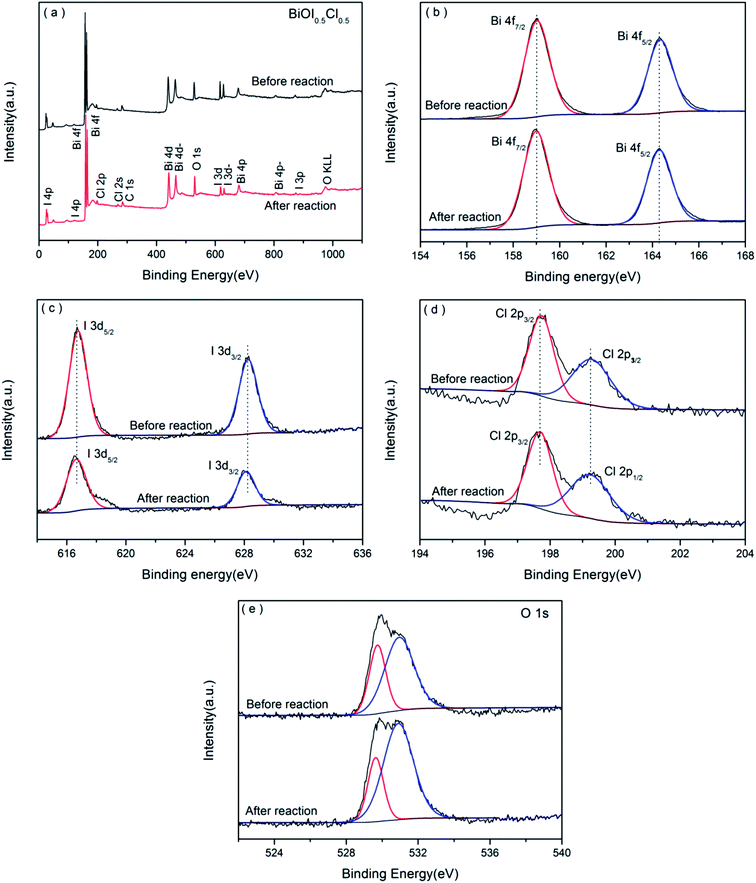 |
| Fig. 4 XPS spectra of catalyst before and after reaction: (a) a typical XPS survey spectrum, and high-resolution XPS of (b) Bi 4f, (c) I 3d, (d) Cl 2p and (e) O 1s. | |
3.2 Degradation efficiency of BPAF in PMS/BiOI0.5Cl0.5 process
The synthesized BiOI0.5Cl0.5 was employed at a dosage of 0.5 g L−1. The removal of BPAF by single PMS, single BiOI0.5Cl0.5 or PMS/BiOI0.5Cl0.5 is shown in Fig. 5. The pH of the initial solutions was about 7.50 ± 0.05. As can be seen, in the absence of BiOI0.5Cl0.5, the degradation of BPAF by PMS was negligible within investigated time scale. Comparatively, the adsorption by single BiOI0.5Cl0.5 contributed 27.3% removal of BPAF. It can be seen that the adsorption capacity increased quickly in the first 2 min and then the adsorption equilibrium was reached within 10 min. In contrast, BPAF could be appreciably degraded in PMS/BiOI0.5Cl0.5 system with 99.1% removal rate at 60 min. It was obvious that peroxymonosulfate had a positive influence on the degradation of BPAF, indicating the generation of ROS (reactive oxygen species) via activation of PMS. 43.9% mineralization was achieved after 60 min reaction, when the nearly complete removal of BPAF was observed, indicating that the significant amounts of reaction by-products were refractory to be oxidized.48
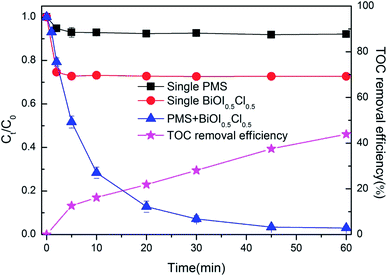 |
| Fig. 5 Removal of BPAF in different reaction systems ([BPAF] = 10 mg L−1, PMS/BPAF = 5/1, BiOI0.5Cl0.5 dosage = 0.5 g L−1, T = 298 K, initial pH = 7.50). | |
Besides, control experiments were carried out to compare the BPAF removal efficiency for various processes at BiOI/PMS and BiOCl/PMS processes within 60 min. As shown in Fig. S2,† the synthesized BiOI0.5Cl0.5 showed the highest activity for activation of PMS to produce sulfate radicals and 99.1% BPAF removal was achieved within 60 min, while BiOI and BiOCl possessed a lower activity and provided 31.3% and 48.3% BPAF removal respectively in the same time. The nitrogen adsorption–desorption test was conducted using the BET method, with the results presented in Fig. S3,† the inset shows the BJH pore size distribution for the prepared samples. BiOI0.5Cl0.5 has a large surface area (44.94 m2 g−1) and pore volume (0.454 cm3 g−1), which is larger than that of individual BiOI (2.499 m2 g−1) or BiOCl (4.992 m2 g−1).36 From the results of this experiment, we get the conclusion that the structure and surface chemistry of the BiOX would of course be expected to affect its activation of peroxides and thus the overall degradation effectiveness and the synthesized BiOI0.5Cl0.5 has the superior activation.49
In the removal process, both the adsorption of BiOI0.5Cl0.5 and the oxidation of PMS activated by BiOI0.5Cl0.5 were simultaneous for BPAF removal. In this work, all the suspensions were magnetically stirred for 10 min in the dark before adding a certain amount of PMS, in order to attain the adsorption–desorption equilibrium between the BiOI0.5Cl0.5 and the BPAF molecules.
3.3 Effect of reaction parameters
3.3.1 Effect of catalyst dosages. The influence of different catalyst dosages on the catalytic degradation of BPAF by PMS/BiOI0.5Cl0.5 system was performed in 0.1–1.0 g L−1 range. Before adding the PMS, the adsorption efficiencies of BPAF by varying the BiOI0.5Cl0.5 dosage were detected in Fig. S4,† the adsorption capacity all increased quickly in the first 2 min and then the adsorption equilibrium was reached within 10 min. Besides, with the improvement of catalyst dosage from 0.5 g L−1 to 1.0 g L−1, the adsorption of BPAF just increased about 4.88%.As shown in Fig. 6(a), the BPAF removal efficiency enhanced from 30.98% to 98.24% with an increase in the loading of prepared sample from 0.1 to 1.0 g L−1 within 45 min reaction time. Furthermore, based on a great agreement with pseudo-first-order kinetic model, Fig. 6(b) shows the plot of reaction rate constant (Kapp) increases from 0.0082 to 0.0959 min−1 with enhancing catalyst dose in the system. However, with extra BiOI0.5Cl0.5 dosages of 1.0 g L−1, the degradation of BPAF slightly changes comparing to the chemical oxidation process with 0.5 g L−1 catalyst dosage addition. The observation can be justified by two convincing reasons.50,51 First of all, the higher of catalyst provides an overly active site of premenstrual syndrome and BPAF adsorption, then the PMS molecule breaks down into more free sulfate radicals. On the other hand, it might be due to the fact that there is a limit to the amount of PMS and the saturated active sites on the catalyst surface are superfluous, which is accordant with the trend of reaction rate constant. In this section, considering the maximum removal of BPAF, the most suitable catalyst dosage is 0.5 g L−1 for the further oxidation experiments.
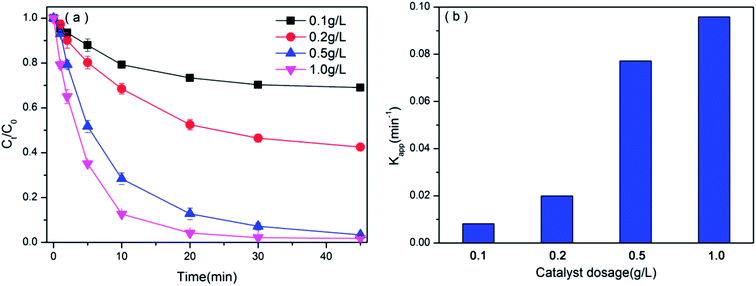 |
| Fig. 6 (a) The degradation efficiencies of BPAF by varying the BiOI0.5Cl0.5 dosage ([BPAF] = 10 mg L−1, PMS/BPAF = 5/1, BiOI0.5Cl0.5 dosage = 0.1–1.0 g L−1, T = 298 K, initial pH = 7.50) and (b) the degradation rate of pseudo-first-order Kapp versus catalyst dosage. | |
3.3.2 Effect of PMS loading. BPAF removal in PMS/BiOI0.5Cl0.5 system was performed with 0.5 g L−1 of catalyst and various loadings of PMS during 45 min reaction, the effect of molar of PMS/BPAF on the catalytic degradation efficiency is shown in Fig. 7(a). Increasing molar ratio of PMS/BPAF from 1 to 5 continually enhances the BPAF degradation efficiency. When PMS/BPAF equals 5, BPAF degradation efficiency could reach 96.61% at 45 min. Nevertheless, with the further increasing molar ratio of PMS/BPAF to 10 or 20, the degradation efficiency is not promoted any more, which can be confirmed by the reaction rate constant dropped from 0.0772 min−1 to 0.0445 min−1 in Fig. 7(b). The reason might own to the invalid PMS scavenging, self-quenching of sulfate and hydroxyl radicals is possible when excessive PMS is added (eqn (2) and (3)).52,53 Moreover, the reaction (eqn (4)) and the recombination (eqn (5) and (6)) between both sulfate and hydroxyl radicals also play an important role in decreasing the catalytic performance of process.12,50 Hence, the optimal molar ratio of PMS/BPAF is 5. |
HSO−5 + ·SO−4 → ·SO−5 + H+
| (2) |
|
HSO−5 + ·OH → ·SO−5 + H2O
| (3) |
|
·SO−4 + ·OH → HSO−4 + 1/2O2
| (4) |
|
·SO−4 + ·SO−4 → 2SO2−4 or S2O2−8
| (5) |
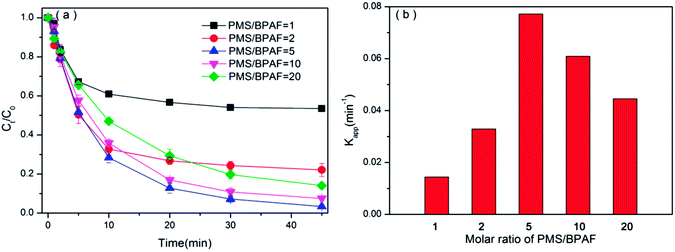 |
| Fig. 7 (a) The degradation efficiencies of BPAF by varying the molar ratio of PMS/BPAF ([BPAF] = 10 mg L−1, BiOI0.5Cl0.5 dosage = 0.5 g L−1, PMS/BPAF = 1–20, T = 298 K, initial pH = 7.50.) and (b) the degradation rate of pseudo-first-order Kapp versus the molar ratio of PMS/BPAF. | |
3.3.3 Effect of solution pH. As BPAF degradation by PMS/BiOI0.5Cl0.5 system is an aqueous reaction, and natural wastewater has inconstant pH values, pH value is one of the most important influence aspects on chemical reaction rate. Hence, it is necessary to investigate the influence of pH on the degradation efficiency of BPAF. It must be noted that the addition of acidic PMS into an unbuffered aqueous solution will lead to a significant decrease in the solution pH. As shown in the Fig. 8(a), one of the pH values of 7.50 was the initial pH of the solution, the effect of initial solution pH on BPAF removal was examined in the range of 4.00 to 10.00. The k1 at pH0 = 6.04 and pH0 = 8.00 is 0.0662 and 0.0729 min−1, respectively, which is close to the k1 at initial pH 7.50 as 0.0772 min−1, suggesting that PMS remained stable under the relatively neutral conditions. However, when the solution became more acidic at pH0 = 4.00, the degradation extent and kinetics were significantly decreased as k1 became 0.0487 min−1, which might be caused by the slower PMS activation at relatively low pH values. Previous studies have shown that PMS is believed to be more stable under acidic conditions.54 With the increase of the solution pH, the dominant PMS species changes from HSO5−˙ to SO52−˙.55 In addition, the degradation rate of BPAF increased significantly from pH 8.00 to 9.98, at the alkaline condition, the base activation of PMS could significantly accelerate BPAF degradation process, which was similar to the previous study on the degradation of benzoic acid using the UV/PMS process.56 In this study, the isoelectric point of BiOI0.5Cl0.5 was obtained as 5.6 V (illustrated in Fig. S5† through zeta potential detection), above which the surface charge was negative and below which the catalyst surface was positive. On the other hand, the pKa of BPAF values of 9.13 and 9.74.57 Therefore, the excellent performance of alkaline pH can be attributed to several factors. Firstly, it was easier to activate a larger fraction of SO52−˙ ions compared to HSO5−˙ with the base activation of PMS. Secondly, the electrostatic repulsion existed between the catalyst surface and BPAF when pH greater than 9.74, thus the suppression effect of increasing BPAF removal was very weak at the alkaline condition from pH 8.00 to 9.98. Finally, the strong hydroxyl radical at basic pH from sulfate (eqn (7)) also accelerated BPAF degradation efficiency.58 |
OH− + ·SO−4 → ·OH + SO2−4
| (7) |
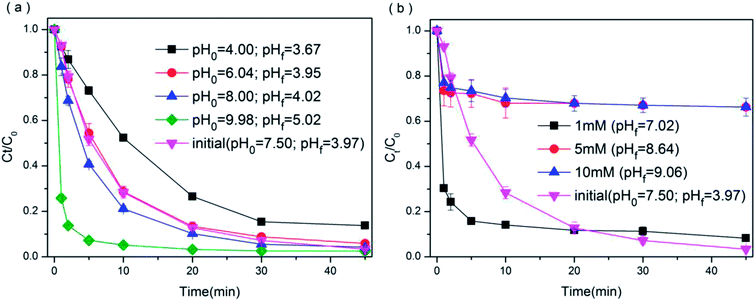 |
| Fig. 8 Effect of initial solution pH (a) and bicarbonate ion concentration (b) on BPAF removal efficiency ([BPAF] = 10 mg L−1, BiOI0.5Cl0.5 dosage = 0.5 g L−1, PMS/BPAF = 5, [HCO3−] = 0–10 mM, T = 298 K). | |
3.3.4 Effect of the various anions. To investigate the effect of the various anions in the degradation of BPAF in PMS/BiOI0.5Cl0.5 system, 0–10 mM Cl−, NO3−, SO42−˙ and HCO3− were selected. Compared with Cl−, NO3−˙ and SO42− as shown in Fig. S6,† a significantly inhibition effect of BPAF degradation was observed when dosing HCO3−˙ shown in Fig. 8(b), and the inhibition was enhanced with increasing HCO3−˙ concentration. It is well known that bicarbonate strongly influence solution pH. Of note, once HCO3−˙ ions were added to the solution, carbonate would be formed and the solution eventually reached the highly pH remained at 7.0–9.0 because of dynamic equilibrium. According to previous studies, HCO3−˙ is an effective scavenger for sulfate radicals (·SO4−) and hydroxyl radicals (·OH) (eqn. (8)–(11)), which can lead to an inhibitory effect on contaminant degradation.59 When added 1 mM HCO3−˙ to the solution, the reaction system was changed to alkaline circumstance, which created excellent reaction condition for the activation of PMS, resulting in the significant increase of the reaction rate,60 and the result was similar to the image of pH = 9 in Fig. 8(a). While with the introduction of high dosage of HCO3−˙ (5 mM and 10 mM), the BPAF removal was inhibited distinctly, because the HCO3−˙ would act as a scavenger for a large proportion of sulfate radicals (·SO4−) and hydroxyl radicals (·OH). |
HCO−3 + ·SO−4 → ·HCO3 + SO2−4
| (8) |
|
CO2−3 + ·SO−4 → ·CO−3 + SO2−4
| (9) |
|
HCO−3 + ·OH → ·CO−3 + H2O
| (10) |
|
CO2−3 + ·OH → ·CO−3 + OH−
| (11) |
3.3.5 Effect of water matrices. To investigate the realistic conditions, the effect of various water matrices including tap water and surface water (from the Minyuan Lake in Sichuan University) on the removal of BPAF was studied using the PMS/BiOI0.5Cl0.5 system, as shown in Fig. S7,† the pH of tap water and surface water is 8.05 and 8.83, respectively. The degradation rate of BPAF was very fast in the first 5 min. However, the removal of BPAF in the PMS/BiOI0.5Cl0.5 system was restrained about 10% in tap water and surface water, which was presumably related to the high TOC levels of tap water (5.57 mg L−1) and surface water (6.87 mg L−1). The main constituents of TOC were involved in hydrophobic, transphilic and hydrophilic DOCs,61 where they can compete with BPAF for ·OH and ·SO4−˙ radicals. Moreover, it is notable that in spite of the higher TOC levels in the two water samples, the PMS/BiOI0.5Cl0.5 system showed high catalytic performance on BPAF removal, indicating that the present reaction system shows the great potential of cleaning BPAF wastewater on the basis of advanced oxidation process.
3.4 Recyclability of catalyst to degrade the BPAF
In order to evaluate the reusability of BiOI0.5Cl0.5 sample, the catalyst was collected by filtration from aqueous solution, washed by ethanol and ultrapure water, then reused for degradation tests. Fig. S8† shows four recycling runs of the BPAF degradation, it was noticeable that the regeneration efficiency (defined as (1 − Ct/C0)%) remained about 47.5% after 4 cycles. The removal of BPAF were decreased 14.53%, 42.05% and 49.10% after each recycling, respectively. This activity decrease in the regeneration efficiency is possible due to the remarkable amounts of both BPAF molecules and by-products are adsorbed on the surface of catalyst, then the catalytic surface activity and the rate of mineralization are limited. Furthermore, some pores on the catalyst surface were blocked, due to the adsorption of BPAF and intermediates from degradation.62
3.5 The mechanism of BiOI0.5Cl0.5 to active PMS for degradation of BPAF
3.5.1 Identification of the reactive species. For the PMS/BiOI0.5Cl0.5 system, it was expected that hydroxyl and/or sulfate radicals can be produced and then react with BPAF molecules. To identify the oxidation of the reaction system, ESR experiment using 5,5-dimethy-1-pyrroline (DMPO) as a radical spin trapping agent were performed. As shown in Fig. 9, the characteristic signals of 5,5-dimethy-2-pyrroline-N-oxyl (DMPOX) with heptet were detected at 3 min, which were formed by the oxidation of DMPO,63 indicating that some strong ROS were produced in the reaction system. Previous studies had proposed transformation pathway of DMPO to DMPOX.64,65 The fact that we did not yet detect ·OH/·SO4−˙ radicals via the ESR test, which did not mean that they were absent. In addition, the signals of DMPOX with heptet were more obvious after adding NaF, which proved that the surface radicals played an important role in the process of degradation of BPAF.
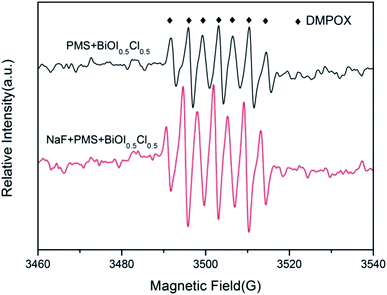 |
| Fig. 9 ESR spectra of BiOI0.5Cl0.5/PMS and BiOI0.5Cl0.5/PMS/NaF system (BiOI0.5Cl0.5 dosage = 0.5 g L−1, PMS = 0.3 mM, [NaF] = 0.03 mol L−1, T = 298 K). | |
To distinguish contribution of hydroxyl and/or sulfate radicals to BPAF degradation, the radical intermediates were probed via radical quenching tests: excess tert-butyl alcohol (TBA) and methanol (MA) were added into the reaction solution as radical scavengers. MA, a kind of alcohol containing an α-hydrogen, can react with ·SO4−˙ and ·OH at significant rates (k·SO4−/MA = 1.6–7.7 × 107 M−1 s−1, k·OH/MA = 1.2–2.8 × 109 M−1 s−1) while TBA is an specific scavenger for ·OH (k·OH/TBA = 3.8–7.6 × 108 M−1 s−1).66 Fig. 10(a) showed that the inhibiting effects of BPAF degradation were not obvious when MA or TBA was added into the BiOI0.5Cl0.5/PMS suspensions. 14.57% and 9.21% of the BPAF degradation were inhibited by MA and TBA, respectively. The reason may be that hydrophilic MA and TBA are superior to competing for free radicals in the liquid phase rather than for large reaction with active radicals on the surface of the catalyst. However, from the inner figure of Fig. 10(a), k1 value decreased from 0.0772 to 0.435 min−1, revealing that the PMS activation was inhibited by TBA and ·OH may be involved in BPAF degradation. What's more, in the presence of methanol, k1 value decreased to 0.0359 min−1. Therefore, the above results suggest that the methanol adequately suppressed PMS activation and the sulfate and hydroxyl radicals had significant effect on the degradation of BPAF, the primary active species was surface bound ·OH/·SO4− in the catalytic oxidation process. Furthermore, the degradation of the PMS in BiOI0.5Cl0.5/PMS and BiOI0.5Cl0.5/PMS/BPAF system was detected. As shown in the Fig. S9,† the degradation rate of PMS increased 18.31% after adding BPAF, indicating that the PMS was involved in the reaction.
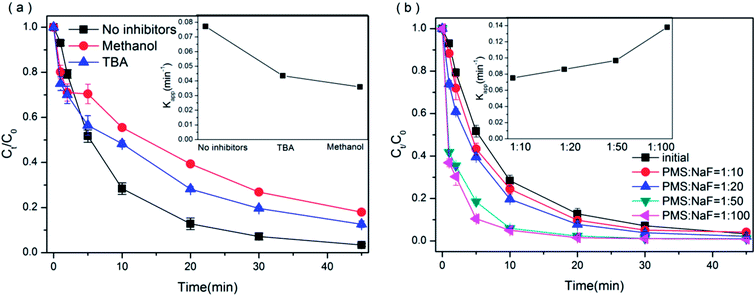 |
| Fig. 10 (a) BPAF degradation in the presence of methanol and TBA as a radical scavenger and (b) in NaF/PMS/BiOI0.5Cl0.5 system. ([BPAF] = 10 mg L−1, BiOI0.5Cl0.5 dosage = 0.5 g L−1, PMS/BPAF = 5, [methanol] = [TBA] = 0.075 mol L−1, [NaF] = 0–0.015 mol L−1, T = 298 K). | |
3.5.2 The mechanism discussion
To further analyze the role of surface bound ·OH/·SO4−˙ generated by the surface of the synthesized BiOI0.5Cl0.5, different concentrations of NaF were added to the solution. Previous researches had shown that F− in the solution could desorb ·OH bound on catalyst by forming strong fluoride-exchange.67,68 As expected in Fig. 10(b) and S9(a),† the addition of F− improved the BPAF degradation and with the more NaF dosage, the more obvious of the PMS degradation effect, the degradation rate of PMS increased 18.20% compared with BiOI0.5Cl0.5/PMS/BPAF system. While there was no obvious promotion in degradation of PMS when adding MA or TBA (shown in Fig. S9(b)†). It should be pointed out that, after F− surface modification, the reduce of surface free radicals might promote the production of radicals in bulk. The results indicated PMS mainly bonded with the active sites of the BiOI0.5Cl0.5 catalyst to generate the surface radicals, which were important rather than the radicals in bulk for BPAF degradation.
Thus, a possible catalysis mechanism of PMS activation by BiOI0.5Cl0.5 for BPAF degradation are proposed. Because PMS is an acidic oxidant with standard reduction potentials for the aqueous half-reactions, which was mainly in the form of HSO5− and SO52− ions in the solution (eqn (12) and (13)). HSO5− and SO52− were adsorbed on the surface of the BiOI0.5Cl0.5 due to the electrostatic attraction, which were easily activated by Bi3+/Bi5+ couples to generate ·SO4− and ·SO5− (eqn. (14)–(16)). Under strong alkaline conditions, ·OH turns out to be the major radical, as shown in eqn (17). In addition, the formation of ·OH, which also can be attributed to reaction between ·SO4− and water (eqn (18)).69,70 Finally, ·OH and ·SO4− on surface of the catalyst attacked adsorbed BPAF organic compounds and triggered a series of further degradations. Based on the above results, a feasible mechanism for the degradation of BPAF concerning the interaction between BiOI0.5Cl0.5 and PMS is proposed in Fig. 11.
|
HSO−5 + H+ + 2e− → SO2−4 + H2O
| (12) |
|
SO2−5 + H2O + 2e− → SO2−4 + OH−
| (13) |
|
Bi3+ + 2HSO−5 → 2·SO−4 + Bi5+ + 2OH−
| (14) |
|
Bi5+ + 2HSO−5 → 2·SO−5 + Bi3+ + 2H+
| (15) |
|
2·SO−5 → 2·SO−4 + O2
| (16) |
|
·SO−4 + OH− → SO2−4 + ·OH
| (17) |
|
·SO−4 + H2O → SO2−4 + H+ + ·OH
| (18) |
|
BPAF + ·SO−4/·OH → degraded products
| (19) |
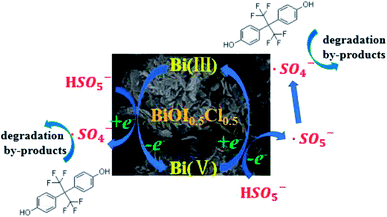 |
| Fig. 11 Proposed mechanism of BPAF degradation in the BiOI0.5Cl0.5/PMS process. | |
4. Conclusion
The obtained results showed that the synthesized catalyst had a good catalytic performance in the activation of PMS to degrade BPAF. Under optimum experimental conditions, the removal efficiencies of BPAF, TOC were found to be 99.1 and 43.9%, respectively. This degradation activity could be enhanced by increasing catalyst dose and initial solution pH. Bicarbonate ions adversely affected the degradation rate of BPAF, while chloride, nitrate and sulfate ions did not represent any effect on the removal of BPAF. In the tap water and surface water, the present reaction system still showed the great potential of cleaning BPAF wastewater. Radical scavengers' experiments verified the dominant active species generated in the BiOI0.5Cl0.5/PMS system were surface-bound ·SO4−˙ and ·OH radicals, thus a possible degradation mechanism of BPAF was proposed. In conclusion, the study provides an option and would be a promising effective technology for BPAF removal.
Conflicts of interest
There are no conflicts of interest to declare.
Acknowledgements
This work was financially supported by the National Natural Science Foundation of China (No. 51508354) and the Science and Technology Projects of Chengdu (No. 2015-HM01-00536-SF). The authors are thankful to all the anonymous reviewers for their insightful comments and suggestions.
References
- J. R. Rochester, Bisphenol A and human health: a review of the literature, Reprod. Toxicol., 2013, 42, 132–155 CrossRef CAS PubMed.
- J. R. Rochester and A. L. Bolden, Bisphenol S and F: A Systematic Review and Comparison of the Hormonal Activity of Bisphenol A Substitutes, Environ. Health Perspect., 2015, 123(7), 643–650 CrossRef CAS PubMed.
- E. M. Rodriguez, G. Fernandez, N. Klamerth, M. I. Maldonado, P. M. Alvarez and S. Malato, Efficiency of different solar advanced oxidation processes on the oxidation of bisphenol A in water, Appl. Catal., B, 2010, 95(3–4), 228–237 CrossRef CAS.
- C. Y. Wang, H. Zhang, F. Li and L. Y. Zhu, Degradation and Mineralization of Bisphenol A by Mesoporous Bi2WO6 under Simulated Solar Light Irradiation, Environ. Sci. Technol., 2010, 44(17), 6843–6848 CrossRef CAS PubMed.
- J. Michalowicz, Bisphenol A - sources, toxicity and biotransformation, Environ. Toxicol. Pharmacol., 2014, 37(2), 738–758 CrossRef CAS PubMed.
- J. R. Kim, S. G. Huling and E. Kan, Effects of temperature on adsorption and oxidative degradation of bisphenol A in an acid-treated iron-amended granular activated carbon, Chem. Eng. J., 2015, 262, 1260–1267 CrossRef CAS.
- C. Huang, P. Xu, G. M. Zeng, D. L. Huang, C. Lai and M. Cheng, et al., The rapid degradation of bisphenol A induced by the response of indigenous bacterial communities in sediment, Appl. Microbiol. Biotechnol., 2017, 101(9), 3919–3928 CrossRef CAS PubMed.
- C. Y. Wang, L. Y. Zhu, C. Song, G. Q. Shan and P. Chen, Characterization of photocatalyst Bi3.84W0.16O6.24 and its photodegradation on bisphenol A under simulated solar light irradiation, Appl. Catal., B, 2011, 105(1–2), 229–236 CrossRef CAS.
- C. Y. Wang, X. Zhang, H. B. Qiu, W. K. Wang, G. X. Huang and J. Jiang, et al., Photocatalytic degradation of bisphenol A by oxygen-rich and highly visible-light responsive Si12O17Cl2 nanobelts, Appl. Catal., B, 2017, 200, 659–665 CrossRef CAS.
- E. B. Simsek, Solvothermal synthesized boron doped TiO2 catalysts: photocatalytic degradation of endocrine disrupting compounds and pharmaceuticals under visible light irradiation, Appl. Catal., B, 2017, 200, 309–322 CrossRef.
- S. Akbari, F. Ghanbari and M. Moradi, Bisphenol A degradation in aqueous solutions by electrogenerated ferrous ion activated ozone, hydrogen peroxide and persulfate: applying low current density for oxidation mechanism, Chem. Eng. J., 2016, 294, 298–307 CrossRef CAS.
- J. Sharma, I. M. Mishra, D. D. Dionysiou and V. Kumar, Oxidative removal of Bisphenol A by UV-C/peroxymonosulfate (PMS): kinetics, influence of co-existing chemicals and degradation pathway, Chem. Eng. J., 2015, 276, 193–204 CrossRef CAS.
- Y. X. Feng, J. Yin, Z. H. Jiao, J. C. Shi, M. Li and B. Shao, Bisphenol AF may cause testosterone reduction by directly affecting testis function in adult male rats, Toxicol. Lett., 2012, 211(2), 201–209 CrossRef CAS PubMed.
- T. Ruan, D. Liang, S. J. Song, M. Y. Song, H. L. Wang and G. B. Jiang, Evaluation of the in vitro estrogenicity of emerging bisphenol analogs and their respective estrogenic contributions in municipal sewage sludge in China, Chemosphere, 2015, 124, 150–155 CrossRef CAS PubMed.
- C. Y. Liao and K. Kannan, Concentrations and Profiles of Bisphenol A and Other Bisphenol Analogues in Foodstuffs from the United States and Their Implications for Human Exposure, J. Agric. Food Chem., 2013, 61(19), 4655–4662 CrossRef CAS PubMed.
- S. K. Khetan and T. J. Collins, Human pharmaceuticals in the aquatic environment: a challenge to green chemistry, Chem. Rev., 2007, 107(6), 2319–2364 CrossRef CAS PubMed.
- Z. M. Ding, X. F. Jiao, D. Wu, J. Y. Zhang, F. Chen and Y. S. Wang, et al., Bisphenol AF negatively affects oocyte maturation of mouse in vitro through increasing oxidative stress and DNA damage, Chem.-Biol. Interact., 2017, 278, 222–229 CrossRef CAS PubMed.
- M. Ahmad, A. L. Teel and R. J. Watts, Mechanism of Persulfate
Activation by Phenols, Environ. Sci. Technol., 2013, 47(11), 5864–5871 CrossRef CAS PubMed.
- H. Zhong, M. L. Brusseau, Y. K. Wang, N. Yan, L. Quig and G. R. Johnson, In situ activation of persulfate by iron filings and degradation of 1,4-dioxane, Water Res., 2015, 83, 104–111 CrossRef CAS PubMed.
- J. Liu, J. H. Zhou, Z. X. Ding, Z. W. Zhao, X. Xu and Z. D. Fang, Ultrasound irritation enhanced heterogeneous activation of peroxymonosulfate with Fe3O4 for degradation of azo dye, Ultrason. Sonochem., 2017, 34, 953–959 CrossRef CAS PubMed.
- A. J. Jafari, B. Kakavandi, N. Jaafarzadeh, R. R. Kalantary, M. Ahmadi and A. A. Babaei, Fenton-like catalytic oxidation of tetracycline by AC@Fe3O4 as a heterogeneous persulfate activator: adsorption and degradation studies, J. Ind. Eng. Chem., 2017, 45, 323–333 CrossRef.
- Y. B. Wang, X. Zhao, D. Cao, Y. Wang and Y. F. Zhu, Peroxymonosulfate enhanced visible light photocatalytic degradation bisphenol A by single-atom dispersed Ag mesoporous g-C3N4 hybrid, Appl. Catal., B, 2017, 211, 79–88 CrossRef CAS.
- P. D. Hu and M. C. Long, Cobalt-catalyzed sulfate radical-based advanced oxidation: a review on heterogeneous catalysts and applications, Appl. Catal., B, 2016, 181, 103–117 CrossRef CAS.
- P. H. Shao, J. Y. Tian, X. G. Duan, Y. Yang, W. X. Shi and X. B. Luo, et al., Cobalt silicate hydroxide nanosheets in hierarchical hollow architecture with maximized cobalt active site for catalytic oxidation, Chem. Eng. J., 2019, 359, 79–87 CrossRef CAS.
- J. Kang, H. Y. Zhang, X. G. Duan, H. Q. Sun, X. Y. Tan and S. M. Liu, et al., Magnetic Ni-Co alloy encapsulated N-doped carbon nanotubes for catalytic membrane degradation of emerging contaminants, Chem. Eng. J., 2019, 362, 251–261 CrossRef CAS.
- J. Wang, X. G. Duan, Q. Dong, F. P. Meng, X. Y. Tan and S. M. Liu, et al., Facile synthesis of N-doped 3D graphene aerogel and its excellent performance in catalytic degradation of antibiotic contaminants in water, Carbon, 2019, 144, 781–790 CrossRef CAS.
- S. S. Zhu, X. C. Huang, F. Ma, L. Wang, X. G. Duan and S. B. Wang, Catalytic Removal of Aqueous Contaminants on N-Doped Graphitic Biochars: Inherent Roles of Adsorption and Nonradical Mechanisms, Environ. Sci. Technol., 2018, 52(15), 8649–8658 CrossRef CAS PubMed.
- L. Q. Ye, Y. R. Su, X. L. Jin, H. Q. Xie and C. Zhang, Recent advances in BiOX (X = Cl, Br and I) photocatalysts: synthesis, modification, facet effects and mechanisms, Environ. Sci.: Nano, 2014, 1(2), 90–112 RSC.
- W. J. Kim, D. Pradhan, B. K. Min and Y. Sohn, Adsorption/photocatalytic activity and fundamental natures of BiOCl and BiOClxI1-x prepared in water and ethylene glycol environments, and Ag and Au-doping effects, Appl. Catal., B, 2014, 147, 711–725 CrossRef CAS.
- H. J. Zhang, Y. X. Yang, Z. Zhou, Y. P. Zhao and L. Liu, Enhanced Photocatalytic Properties in BiOBr Nanosheets with Dominantly Exposed (102) Facets, J. Phys. Chem. C, 2014, 118(26), 14662–14669 CrossRef CAS.
- L. M. Sun, L. Xiang, X. Zhao, C. J. Jia, J. Yang and Z. Jin, et al., Enhanced Visible-Light Photocatalytic Activity of BiOI/BiOCl Heterojunctions: Key Role of Crystal Facet Combination, ACS Catal., 2015, 5(6), 3540–3551 CrossRef CAS.
- X. F. Chang, M. A. Gondal, A. A. Al-Saadi, M. A. Ali, H. F. Shen and Q. Zhou, et al., Photodegradation of rhodamine B over unexcited semiconductor compounds of BiOCl and BiOBr, J. Colloid Interface Sci., 2012, 377, 291–298 CrossRef CAS PubMed.
- H. Li and L. Zhang, Photocatalytic performance of different exposed crystal facets of BiOCl, Curr. Opin. Green Sustain. Chem., 2017, 6, 48–56 CrossRef.
- T. Zhang, S. Chu, J. Li, L. Wang, R. Chen and Y. Shao, et al., Efficient Degradation of Aqueous Carbamazepine by Bismuth Oxybromide-Activated Peroxide Oxidation, Catalysts, 2017, 7(11), 315 CrossRef.
- X. N. Wang, W. L. Bi, P. P. Zhai, X. B. Wang, H. J. Li and G. Mailhot, et al., Adsorption and photocatalytic degradation of pharmaceuticals by BiOClxIy nanospheres in aqueous solution, Appl. Surf. Sci., 2016, 360, 240–251 CrossRef CAS.
- Y. Y. Zhang, X. G. Sun, G. Z. Yang, Y. H. Zhu, H. Y. Si and J. M. Zhang, et al., Preparation and characterization of bifunctional BiOClxIy solid solutions with excellent adsorption and photocatalytic abilities for removal of organic dyes, Mater. Sci. Semicond. Process., 2016, 41, 193–199 CrossRef CAS.
- K. Natarajan, H. C. Bajaj and R. J. Tayade, Photocatalytic efficiency of bismuth oxyhalide (Br, Cl and I) nanoplates for RhB dye degradation under LED irradiation, J. Ind. Eng. Chem., 2016, 34, 146–156 CrossRef CAS.
- J. Cao, B. Y. Xu, B. D. Luo, H. L. Lin and S. F. Chen, Novel BiOI/BiOBr heterojunction photocatalysts with enhanced visible light photocatalytic properties, Catal. Commun., 2011, 13(1), 63–68 CrossRef CAS.
- J. X. Xia, J. Di, H. T. Li, H. Xu, H. M. Li and S. J. Guo, Ionic liquid-induced strategy for carbon quantum dots/BiOX (X=Br, Cl) hybrid nanosheets with superior visible light-driven photocatalysis, Appl. Catal., B, 2016, 181, 260–269 CrossRef CAS.
- J. Xie, Y. L. Cao, D. Z. Jia, H. Y. Qin and Z. T. Liang, Room-temperature solid-state synthesis of BiOCl hierarchical microspheres with nanoplates, Catal. Commun., 2015, 69, 34–38 CrossRef CAS.
- B. Priya, P. Raizada, N. Singh, P. Thakur and P. Singh, Adsorptional photocatalytic mineralization of oxytetracycline and ampicillin antibiotics using Bi2O3/BiOCl supported on graphene sand composite and chitosan, J. Colloid Interface Sci., 2016, 479, 271–283 CrossRef CAS PubMed.
- L. Lu, M. Y. Zhou, L. Yin, G. W. Zhou, T. Jiang and X. K. Wan, et al., Tuning the physicochemical property of BiOBr via pH adjustment: towards an efficient photocatalyst for degradation of bisphenol A, J. Mol. Catal. A: Chem., 2016, 423, 379–385 CrossRef CAS.
- M. Cui, J. X. Yu, H. J. Lin, Y. Wu, L. H. Zhao and Y. He, In situ preparation of Z-scheme AgI/Bi5O7I hybrid and its excellent photocatalytic activity, Appl. Surf. Sci., 2016, 387, 912–920 CrossRef CAS.
- G. C. Chen, M. M. Zhu and X. W. Wei, Photocatalytic properties of attached BiOCl-(001) nanosheets onto AgBr colloidal spheres toward MO and RhB degradation under an LED irradiation, Mater. Lett., 2018, 212, 182–185 CrossRef CAS.
- X. Zhang, L. Z. Zhang, T. F. Xie and D. J. Wang, Low-Temperature Synthesis and High Visible-Light-Induced Photocatalytic Activity of BiOI/TiO2 Heterostructures, J. Phys. Chem. C, 2009, 113(17), 7371–7378 CrossRef CAS.
- H. L. Lin, H. F. Ye, X. Li, J. Cao and S. F. Chen, Facile anion-exchange synthesis of BiOI/BiOBr composite with enhanced photoelectrochemical and photocatalytic properties, Ceram. Int., 2014, 40(7), 9743–9750 CrossRef CAS.
- Y. Xu, J. Ai and H. Zhang, The mechanism of degradation of bisphenol A using the magnetically separable CuFe2O4/peroxymonosulfate heterogeneous oxidation process, J. Hazard. Mater., 2016, 309, 87–96 CrossRef CAS PubMed.
- X. Cheng, H. G. Guo, Y. L. Zhang, X. Wu and Y. Liu, Non-photochemical production of singlet oxygen via activation of persulfate by carbon nanotubes, Water Res., 2017, 113, 80–88 CrossRef CAS PubMed.
- T. Q. Zhang, S. P. Chu, J. Li, L. L. Wang, R. Chen and Y. Shao, et al., Efficient Degradation of Aqueous Carbamazepine by Bismuth Oxybromide-Activated Peroxide Oxidation, Catalysts, 2017, 7(11), 315 CrossRef.
- C. Cai, H. Zhang, X. Zhong and L. W. Hou, Ultrasound enhanced heterogeneous activation of peroxymonosulfate by a bimetallic Fe-Co/SBA-15 catalyst for the degradation of orange II in water, J. Hazard. Mater., 2015, 283, 70–79 CrossRef CAS PubMed.
- Y. Feng, J. H. Liu, D. L. Wu, Z. Y. Zhou, Y. Deng and T. Zhang, et al., Efficient degradation of sulfamethazine with CuCo2O4 spinel nanocatalysts for peroxymonosulfate activation, Chem. Eng. J., 2015, 280, 514–524 CrossRef CAS.
- Y. C. Du, W. J. Ma, P. X. Liu, B. H. Zou and J. Ma, Magnetic CoFe2O4 nanoparticles supported on titanate nanotubes (CoFe2O4/TNTs) as a novel heterogeneous catalyst for peroxymonosulfate activation and degradation of organic pollutants, J. Hazard. Mater., 2016, 308, 58–66 CrossRef CAS PubMed.
- Y. X. Wang, H. Q. Sun, H. M. Ang, M. O. Tade and S. B. Wang, Magnetic Fe3O4/carbon sphere/cobalt composites for catalytic oxidation of phenol solutions with sulfate radicals, Chem. Eng. J., 2014, 245, 1–9 CrossRef CAS.
- W. L. Guo, S. N. Su, C. L. Yi and Z. M. Ma, Degradation of antibiotics amoxicillin by Co3O4-catalyzed peroxymonosulfate system, Environ. Prog. Sustainable Energy, 2013, 32(2), 193–197 CrossRef CAS.
- C. D. Qi, X. T. Liu, C. Y. Lin, H. J. Zhang, X. Li and J. Ma, Activation of peroxymonosulfate by microwave irradiation for degradation of organic contaminants, Chem. Eng. J., 2017, 315, 201–209 CrossRef CAS.
- Y. H. Guan, J. Ma, X. C. Li, J. Y. Fang and L. W. Chen, Influence of pH on the Formation of Sulfate and Hydroxyl Radicals in the UV/Peroxymonosulfate System, Environ. Sci. Technol., 2011, 45(21), 9308–9314 CrossRef CAS PubMed.
- Y. J. Choi and L. S. Lee, Partitioning Behavior of Bisphenol Alternatives BPS and BPAF Compared to BPA, Environ. Sci. Technol., 2017, 51(7), 3725–3732 CrossRef CAS PubMed.
- M. M. Ahmed, S. Barbati, P. Doumenq and S. Chiron, Sulfate radical anion oxidation of diclofenac and sulfamethoxazole for water decontamination, Chem. Eng. J., 2012, 197, 440–447 CrossRef.
- Y. Q. Zhang, J. F. Zhang, Y. J. Xiao, V. W. C. Chang and T. T. Lim, Kinetic and mechanistic investigation of azathioprine degradation in water by UV, UV/H2O2 and UV/persulfate, Chem. Eng. J., 2016, 302, 526–534 CrossRef CAS.
- J. Deng, Y. J. Ge, C. Q. Tan, H. Y. Wang, Q. S. Li and S. Q. Zhou, et al., Degradation of ciprofloxacin using alpha-MnO2 activated peroxymonosulfate process: effect of water constituents, degradation intermediates and toxicity evaluation, Chem. Eng. J., 2017, 330, 1390–1400 CrossRef CAS.
- H. K. Shon, S. Vigneswaran and S. A. Snyder, Effluent Organic Matter (EfOM) in Wastewater: Constituents, Effects, and Treatment, Crit. Rev. Environ. Sci. Technol., 2006, 36(4), 327–374 CrossRef CAS.
- A. Takdastan, B. Kakavandi, M. Azizi and M. Golshan, Efficient activation of peroxymonosulfate by using ferroferric oxide supported on carbon/UV/US system: a new approach into catalytic degradation of bisphenol A, Chem. Eng. J., 2018, 331, 729–743 CrossRef CAS.
- S. D. Stan and M. A. Daeschel, 5,5-Dimethyl-2-pyrrolidone-N-oxyl formation in electron spin resonance studies of electrolyzed NaCl solution using 5,5-dimethyl-1-pyrroline-N-oxide as a spin trapping agent, J. Agric. Food Chem., 2005, 53(12), 4906–4910 CrossRef CAS PubMed.
- M. Zalibera, P. Rapta, A. Stasko, L. Brindzova and V. Brezova, Thermal generation of stable [image omitted] spin trap adducts with super-hyperfine structure in their EPR spectra: an alternative EPR spin trapping assay for radical scavenging capacity determination in dimethylsulphoxide, Free Radical Res., 2009, 43(5), 457–469 CrossRef CAS PubMed.
- X. Chen, W. D. Oh, Z. T. Hu, Y. M. Sun, R. D. Webster and S. Z. Li, et al., Enhancing sulfacetamide degradation by peroxymonosulfate activation with N-doped graphene produced through delicately-controlled nitrogen functionalization via tweaking thermal annealing processes, Appl. Catal., B, 2018, 225, 243–257 CrossRef CAS.
- M. Nie, Y. Yang, Z. Zhang, C. Yan, X. Wang and H. Li, et al., Degradation of chloramphenicol by thermally activated persulfate in aqueous solution, Chem. Eng. J., 2014, 246, 373–382 CrossRef CAS.
- X. J. Hou, X. P. Huang, F. L. Jia, Z. H. Ai, J. C. Zhao and L. Z. Zhang, Hydroxylamine Promoted Goethite Surface Fenton Degradation of Organic Pollutants, Environ. Sci. Technol., 2017, 51(9), 5118–5126 CrossRef CAS PubMed.
- H. Sheng, Q. Li, W. H. Ma, H. W. Ji, C. C. Chen and J. C. Zhao, Photocatalytic degradation of organic pollutants on surface anionized TiO2: common effect of anions for high hole-availability by water, Appl. Catal., B, 2013, 138, 212–218 CrossRef.
- W. D. Oh, Z. Dong, G. Ronn and T. T. Lim, Surface-active bismuth ferrite as superior peroxymonosulfate activator for aqueous sulfamethoxazole removal: performance, mechanism and quantification of sulfate radical, J. Hazard. Mater., 2017, 325, 71–81 CrossRef CAS PubMed.
- X. L. Zhang, M. B. Feng, R. J. Qu, H. Liu, L. S. Wang and Z. Y. Wang, Catalytic degradation of diethyl phthalate in aqueous solution by persulfate activated with nano-scaled magnetic CuFe2O4/MWCNTs, Chem. Eng. J., 2016, 301, 1–11 CrossRef CAS.
Footnote |
† Electronic supplementary information (ESI) available. See DOI: 10.1039/c9ra01687b |
|
This journal is © The Royal Society of Chemistry 2019 |
Click here to see how this site uses Cookies. View our privacy policy here.