DOI:
10.1039/C9RA01605H
(Paper)
RSC Adv., 2019,
9, 16701-16712
Efficient acylation of gastrodin by Aspergillus oryzae whole-cells in non-aqueous media†
Received
3rd March 2019
, Accepted 20th May 2019
First published on 29th May 2019
Abstract
Gastrodin, a bioactive compound extracted from the plant source of Gastrodia elata Blume, has a wide range of therapeutic effects on central nervous system (CNS) diseases, but suffers from poor brain permeability and short half-life in plasma. In this study, fatty acid esters of gastrodin were successfully synthesized by a whole cell-based biocatalytic method. Aspergillus oryzae cells showed different catalytic activities in the organic solvent systems tested. Tetrahydrofuran was confirmed as the most suitable pure organic solvent, with the highest substrate conversion of 98.0%. Addition of ionic liquids (ILs) into pyridine dramatically accelerated the reaction with conversion increased from 5.9% to 84.2%, and also changed the selectivity of the cells, mainly due to the use of IL-containing systems altering cell permeability and contact of the enzymes with solvent molecules possessing different polarities. The ester products were characterized by 13C-NMR and ESI-MS as gastrodin monoester and diester.
1. Introduction
The incidence of central nervous system (CNS) disorders has grown dramatically with the increase in lifespan, placing a huge economic burden on both families and healthcare systems around the world. CNS disorders generally refer to gradual and progressive degeneration of the structure and function of CNS, such as Alzheimer's disease (AD), Parkinson's disease (PD) and epilepsy.1 In 2016, the global number of individuals suffering from dementia was 43.8 million, increased from 20.2 million in 1990.2 It has been forecasted that, by 2050, the worldwide prevalence of AD alone will grow to 106.8 million cases as the world's population ages and grows.3 Efforts have been made to clarify their pathogenesis. However, neither a consensus about the underlying mechanisms of these diseases is reached, nor are there effective therapies for their treatments.4 The increasing prevalence and complexity of their pathogenesis make treatment of CNS diseases an urgent but daunting task. Natural products, most of which are derived from medicinal herbs, are interesting for development of novel drugs in CNS disorders. Gastrodin is a natural phenolic glycoside extracted from Gastrodia elata Blume and has been reported to have broad beneficial effects on CNS diseases, because of its bioactivities such as modulating neurotransmitters, antioxidative, anti-inflammatory and suppressing microglial activation,5–9 Both in vivo and in vitro studies demonstrated its potential therapeutic effects in treating AD by protecting neurons against amyloid beta peptide.5 Although gastrodin can penetrate the blood–brain barrier (BBB) and blood–cerebrospinal–fluid barrier, the maximum concentration of gastrodin in the brain is generally much lower than that in the blood. Clinical data showed that gastrodin could be quickly absorbed with a short absorption half-life perhaps due to its high hydrophilicity. Since its elimination is so quick, and its distribution in brain is low, its exposure time in brain is short, greatly limiting its therapeutic efficiency. To overcome these drawbacks, high-dose supplementation is usually adopted. In addition, previous reports showed that a gastrodin derivative bearing lipophilic groups had better ability than gastrodin itself to improve dementia induced by scopolamine with prolonged duration time of action in the pharmacokinetics in rats.10,11 These derivatives may mainly function as a prodrug, hydrolyzed into gastrodin after penetrating into the brain.12 However, the modification of gastrodin still remains largely unexplored.
In recent decades, biocatalysis, which uses enzymes or whole cells as natural catalysts, has been used as an important strategy in medicinal chemistry because of its high efficacy, specific selectivity, mild reaction conditions, and environmentally-friendliness.13,14 The main obstacle blocking the wide application of enzyme-mediated biocatalysis is the limited number of commercialized enzymes and their high-cost. Whole-cells are promising catalysts that differ from pure enzymes, as they are more stable and efficient because of the protective effect of the natural cell structures. Furthermore, the use of whole cells avoids complex separation and purification steps for pure enzyme production, greatly lowering the cost of the whole process.15,16
In the present study, a whole cell based biocatalytic method was developed for selective acyl modification of gastrodin. A strain of Aspergillus oryzae, widely used in food industry, was used as the whole-cells catalyst because of its high lipase activity observed in our previous study. To facilitate the acylation reaction, various non-aqueous media, including traditional organic solvents and ionic liquid (IL)-containing systems were evaluated as reaction media. To better understand the mechanisms of the solvent effects on the whole-cell mediated reaction, influences of the solvents on the membrane permeability, polysaccharide dissolving in cell wall, and surface morphologies of cells were analyzed.
2. Methods and materials
2.1. Microorganism and chemicals
Aspergillus oryzae GIM 3.4826 was purchased from Guangdong Culture Collection Center (Guangzhou, China). Gastrodin (97% purity) and vinyl propionate (VP, 98% purity) were supplied by TCI (Tokyo, Japan). 1-Ethyl-3-methylimidazolium tetrafluoroborate ([EMIM][BF4], 97% purity), 1-butyl-3-methylimidazolium tetrafluoroborate ([BMIM][BF4], 97% purity), 1-hexyl-3-methylimidazolium tetrafluoroborate ([HMIM][BF4], 97% purity), 1-methyl-3-octylimidazolium tetrafluoroborate ([OMIM][BF4], 98% purity), 1-butyl-3-methylimidazolium hexafluorophosphate ([BMIM][PF6], 97% purity), 1-hexyl-3-methylimidazolium hexafluorophosphate ([HMIM][PF6], 97% purity), 1-methyl-3-octylimidazolium hexafluorophosphate ([OMIM][PF6], 95% purity) were purchased from Aladdin Industrial Corporation (Shanghai, China). All other chemicals were supplied from other commercial sources.
2.2. Cell culture conditions and preparation of whole-cell catalysts
Aspergillus oryzae cells were initially activated in potato dextrose agar (PDA) culture medium at 28 °C for 60 h, and spore suspension was obtained by dissolving spores with distilled water. The spore suspension (3%, v/v) were then transferred to 500 mL flasks with 300 mL culture medium containing yeast extract (5.0 g L−1), (NH4)2SO4 (5.0 g L−1), KH2PO4 (1.0 g L−1), MgSO4·7H2O (0.2 g L−1) and soybean oil (5.0 g L−1), on a rotary shaker at 37 °C and 180 rpm for 48 h. Before freeze-dried in vacuum, the mycelium were harvested by vacuum filtration and washed three times to remove the residual medium from the cell surface. Finally, the dried mycelium were milled into powder and stored at 4 °C. The experiments were performed more than triplicate.
2.3. General procedure for whole-cell catalyzed acylation of gastrodin
In a typical experiment, 1 mL reaction solvents containing 20 mM gastrodin, 600 mM VP, and 40 mg mL−1 Aspergillus oryzae whole-cell catalyst were added into a 5 mL Erlenmeyer flask (rubber serum cap), and incubated at 40 °C with a shaking speed of 180 rpm for 24 h. Aliquots (50 μL) were collected at specified time intervals from the reaction mixture, diluted 20 times with methanol, and analyzed using HPLC. To identify the product structure, the scaled-up reaction mixture was centrifuged to remove the cell pellet. The reaction liquid was purified with methanol via reduced pressure distillation and separated into different components with thin-layer chromatography (TLC). After crystallization under vacuum drying, two products were obtained. The experiments were performed triplicate. The data are presented as mean ± SD.
2.4. Membrane permeability, polysaccharide dissolution and SEM analysis of cell incubated in different IL-containing systems
In a typical experiment, the Aspergillus oryzae whole-cell catalysts (40 mg mL−1) were incubated in different IL-containing systems at 180 rpm and 40 °C for 72 h. The supernatants were removed from the reaction mixture for measurement of protein content and polysaccharide content. The protein contents in the supernatants were used as indicator to measure the permeability of cell membrane and were detected using the BCA Protein Assay Kit purchased from Dalian Meilun Biotechnology Co., Ltd. The polysaccharide contents in the supernatants were used to measure the soluble degree of IL-containing systems to the cell wall of Aspergillus oryzae and were determined by phenol sulfuric acid method. The cells were freeze-dried in vacuum and analyzed by SEM using a ZEISS EVO 18 scanning electron microscope (ZEISS Corp., Germany). The experiments were performed triplicate. The data are presented as mean ± SD.
2.5. Determination of viscosity in IL-containing systems
The viscosity data of tested ILs was recovered from IL Thermo Database (“NIST Ionic Liquids Database (IL Thermo) https://ilthermo.boulder.nist.gov/”).
The predicted viscosity of the binary system was calculated according to the ideal Grunberg–Nissan equation:
|
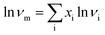 | (1) |
where
νm is the kinematic viscosity of the binary system, and
xi and
νi are the mole fraction and the corresponding kinematic viscosity of component i, respectively.
17
2.6. HPLC analysis
The reaction mixture was analyzed by RP-HPLC on a 4.6 mm × 250 mm (5 μm) Zorbax SB-C18 column (Agilent Technologies Co. Ltd., Massachusetts, USA) using a Waters 600E pump and a Waters 2475 fluorescence detector (Waters Corp., Massachusetts, USA) at excitation wavelength of 268 nm, absorption wavelength of 290 nm. A mixture of methanol and water (40/60, v/v) was used as the mobile phase with a flow rate of 0.9 mL min−1. The retention times for gastrodin and gastrodin mono-propionate, gastrodin di-propionate were 3.251 min, 6.086 min and 9.230 min, respectively. The gastrodin solubility was determined by HPLC analysis using its saturated solutions in different solvents at 25 °C. The conversion of the reaction, initial rate and monoester selectivity were calculated using the following eqn (2)–(4), respectively. The experiments were performed triplicate. The data are presented as mean ± SD. |
Conversion (%) = (S0 − St)/S0 × 100%
| (2) |
|
V0 (mmol L−1 h−1) = (C0 − Ct)/t
| (3) |
|
Monoester selectivity (%) = SPi/Stotal × 100%
| (4) |
where S0 is the peak area of gastrodin before reaction; St is the peak area of gastrodin after reaction for t hours; C0 is the concentration of gastrodin before reaction (mM); Ct is the concentration of gastrodin after reaction for t hours (mM); Stotal is the sum of peak areas of all products; and SP is the peak area of the product i (Pi).
2.7. Structural characterization of products
The acylation positions of the products were determined by 13C-NMR (Bruker AVANCE Digital 400 MHz Nuclear Magnetic Resonance Spectrometer, Bruker Co., Germany). Chemical shifts were indicated as ppm shifts. The mass spectra of the products were obtained on an Agilent 1290/Bruker MaXis Impact Plus ESI Mass Spectrometer with a spray voltage of 4.5 kV (Bruker Co., Germany).
Gastrodin. 13C NMR (101 MHz, DMSO) δ: 156.76 (C-4), 136.28 (C-1), 128.14 (C-2, C-6), 116.39 (C-3, C-5), 101.00 (C-1′), 77.45 (C-5′), 77.10 (C-3′), 73.72 (C-2′), 70.20 (C-4′), 62.97 (C-7), 61.18 (C-6′).
Gastrodin mono-propionate (GME). 13C NMR (151 MHz, MeOD) δ: 156.90 (C-4), 135.45 (C-1), 129.38 (C-2, C-6), 116.35 (C-3, C-5), 100.93 (C-1′), 76.47 (C-3′), 74.01(C-5′), 73.48 (C-2′), 70.33 (C-4′), 63.39 (C-6′), 63.32 (C-7), 174.58 (C
O), 28.83 (CH3CH2CH2–), 8.00 (CH3–). ESI–MS (m/z): 365.1207 (M + Na)+.
Gastrodin di-propionate (GDE). 13C NMR (101 MHz, DMSO) δ: 157.38 (C-4), 130.02 (C-1, C-2, -6), 116.50 (C-3, C-5), 100.40 (C-1′), 76.75 (C-3′), 74.13 (C-5′), 73.53 (C-2′), 70.39 (C-4′), 65.48 (C-7), 63.79 (C-6′), 174.06 (C
O), 173.97 (C
O), 27.29 (CH3CH2CH2–), 27.29 (CH3CH2CH2–), 9.41 (CH3–). ESI-MS (m/z): 421.1469 (M + Na)+.
3. Results and discussion
3.1. Whole-cell catalyzed acylation of gastrodin in organic solvents
Reaction medium plays a crucial part in biocatalytic reaction, influencing both the substrate solubility and the catalytic behaviors of biocatalysts. Since the presence of water may lead to hydrolysis of both the ester products and the vinyl ester, non-aqueous media, such as traditional organic solvents, were adopted as reaction media. Herein, seven organic solvents with different polarities from −1.30 to 4.5 were tested for the reaction (Table 1). Gastrodin had high solubilities in high polar solvents such as DMSO (1007.0 mmol L−1) and DMF (734.2 mmol L−1), while in less polar organic solvents, the solubility of gastrodin was relatively low (2.5–52.0 mmol L−1). In acetonitrile and 2-MeTHF, gastrodin was hardly dissolved. Thus, these two solvents were unsuitable for using as reaction media. The acylation of gastrodin hardly occurred in strong polar organic solvents such as DMSO and DMF, maybe because solvents with strong polarity inactivated the enzymes of the whole cells by easily stripping essential water from enzymes.18,19 The maximal conversion (98.0%) of gastrodin was obtained in THF, followed by using 2-methyl-2-butanol (62.8%). The products were purified and characterized by 13C-NMR and ESI–MS. It was found that the Aspergillus oryzae whole-cells can catalyze the acylation at two hydroxyl groups of gastrodin, the 6′-OH of the sugar moiety and the 7-OH of the gastrodin aglycon, resulting in the formation of gastrodin monoester (GME) and diester (GDE). The organic solvents evidently influenced the selectivity of the whole-cells. In THF, the cells preferred to catalyze the 6′-OH of the sugar moiety. Thus, the monoester selectivity in THF reached 78.0%, while in 2-methyl-2-butanol, the diester GME was the dominant product (54.3%). No tri-esters or multiple esters were detected in the reaction systems tested. In addition, no evident dependences of the biocatalytic activity and selectivity of the cells on log
P values of the solvents were found, indicating that the catalytic behaviors of the biocatalysts might also be related to the nature of the solvent itself. Taking both substrate solubility and conversion into account, THF was considered as the most suitable organic solvent for acylation of gastrodin.
Table 1 Effect of organic solvents on the catalytic activity of Aspergillus oryzae in the acylation of gastrodin
Organic solvent |
log P |
Solubility (mmol L−1) |
Conversion (%) |
Monoester selectivity (%) |
Solvent with very low gastrodin solubility was not designed for subsequent reaction. No product detected. |
DMSO |
−1.30 |
1007.0 ± 2.74 |
NDb |
ND |
DMF |
−1.00 |
734.2 ± 2.07 |
ND |
ND |
Acetonitrile |
−0.33 |
7.1 ± 0.09 |
—a |
— |
THF |
0.49 |
47.7 ± 0.24 |
98.0 ± 0.12 |
78.0 ± 0.02 |
Pyridine |
0.71 |
865.6 ± 0.62 |
5.9 ± 0.15 |
>98 |
2-Methyl-2-butanol |
1.15 |
52.0 ± 0.09 |
62.8 ± 0.16 |
54.3 ± 0.63 |
2-MeTHF |
1.85 |
2.5 ± 0.04 |
— |
— |
Petroleum ether |
3.00 |
<1.0 |
— |
— |
2,2,4-Trimethylpentane |
4.50 |
<1.0 |
— |
— |
3.2. Effect of several key factors on whole-cell catalyzed acylation of gastrodin in organic solvents
For a better understanding of the catalytic behaviors of the whole cells in organic solvents, as well as further improving the reaction efficiency, the effects of several crucial reaction factors on biocatalytic acylation of gastrodin were examined.
As shown in Fig. 1A, the conversion of gastrodin increased with the increase in whole-cell dosage. When the dosage reached 50 mg mL−1, the conversion reached the maximum of 99.7%. Further increasing the dosage was not useful to increase the reaction conversion, as the amount of catalyst exceeded the needs of the reaction. The initial rate of the reaction kept rising within the range tested, increased from 3.8 mmol L−1 h−1 to 6.7 mmol L−1 h−1, mainly due to the fact that there was a large amount of substrate molecules in the initial stage, and the more catalysts were added, the more substrate molecules were converted per unit time. A dosage of whole-cells higher than 50 mg mL−1 did not influence the reaction equilibration, but shortened the time required to reach equilibration. As shown in Fig. 1B, the substrate conversion continuously increased in the temperature ranges of 20–50 °C, reaching its peak (98.8%) at 50 °C. The initial rates of the reaction increased sharply in the range of 20–40 °C, from 3.24 mmol L−1 h−1 to 6.25 mmol L−1 h−1, and then slowly increased from 40 °C to 55 °C. Generally, the temperature affects both catalytic activity and the stability of the biocatalysts. Increase in reaction temperature enhances the probability of collision between the substrate and catalyst, and increases the mass transfer efficiency between the reaction medium and cell membrane, and increases the diffusion of substrate molecules to the active sites of enzymes. However, high temperature may lead to inactivation of the proteins. Temperature-tolerance range of enzymes greatly depends on the types of enzymes. The Aspergillus oryzae cells show relatively strong temperature tolerance, maintaining their catalytic activities at 55 °C. Fig. 1C shows the significant impact of water content on this acylation reaction. The conversion and initial rate kept decreasing along with the increase in water contents from 0% to 5%. The conversion dropped from 97.9% to 0%, and the initial rate showed the same trend, decreasing sharply to 0 mmol L−1 h−1. As a crucial factor for lipase-involved non-aqueous reaction, water is essential for maintaining the three-dimensional structure of enzyme molecules, but excess water promotes the decomposition of the product and acyl donor, and water cluster around the enzyme hinders the approach of substrate towards the enzyme, decreasing the reaction efficiency. The initial water content in pure THF is adequate for the cells to show their catalytic activity. Additional water is unnecessary for the reaction. Fig. 1D shows a positive relationship between molar ratio of the two substrates and the reaction efficiency. When the molar ratio of gastrodin to VP increased from 1
:
1 to 1
:
40, substrate conversion and initial rate increased drastically (substrate conversion increased from 10.0% to 99.0%; initial rate increased from 0.15 to 4.8 mmol L−1 h−1). Because acylation of gastrodin is a two-substrate involved reaction, excess of one substrate obviously promotes reaction efficiency. In addition, considering the side reaction of hydrolysis of vinyl ester,20 use of the acyl donor above its stoichiometric point in the reaction is needed. Fig. 3S† showed the time course of the biocatalytic reaction. It can be found that the monoester in THF is gradually increasing during the whole process, with a monoester selectivity of 78.0% in 24 h.
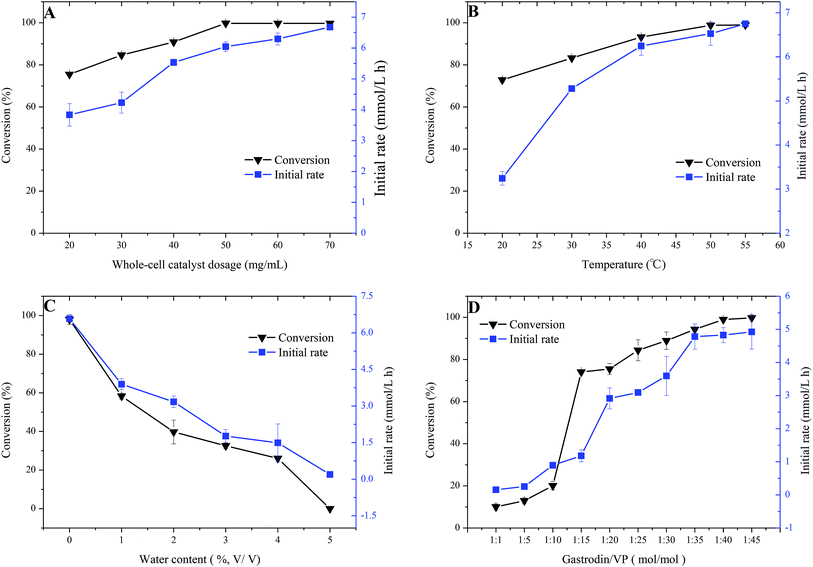 |
| Fig. 1 Effects of whole-cell dosage (A), temperature (B), water content (C) and molar ratio of substrate to acyl donor (D) on the acylation of gastrodin catalyzed by Aspergillus oryzae. (A) The reaction was carried out in THF (1 mL) with gastrodin (20 mM), water content (0), molar ratio of substrate to VP (1 : 30) at 40 °C and 180 rpm for 24 h. (B) The reaction was carried out in THF (1 mL) with gastrodin (20 mM), whole-cell dosage (40 mg mL−1), water content (0), molar ratio of substrate to VP (1 : 30) at 180 rpm for 24 h. (C) The reaction was carried out in THF (1 mL) with gastrodin (20 mM), whole-cell dosage (40 mg mL−1), molar ratio of substrate to VP (1 : 30) at 40 °C and 180 rpm for 24 h. (D) The reaction was carried out in THF (1 mL) with gastrodin (20 mM), whole-cell dosage (40 mg mL−1), water content (0), at 40 °C and 180 rpm for 24 h. | |
Hence, the whole-cell catalyzed reaction can be carried out with the highest substrate conversion of 99.7% and initial rate of 6.25 mmol L−1 h−1 under the conditions of 50 mg mL−1 whole-cell catalyst, 50 °C, molar ratio of gastrodin to VP, 1
:
40, without extra water in THF.
3.3. Whole-cell catalyzed acylation of gastrodin in IL–organic solvent binary systems
Previously reports showed that some ILs, such as [BMIM][PF6] and [BMIM][BF4], could markedly boost the activity and the stability of enzymes and whole cells.21–23 The concentrations of polar substrate could also be improved by using ILs.24 Various ILs were explored as reaction media for the acylation of gastrodin catalyzed by Aspergillus oryzae cells in this research. Considering the very high viscosity of ILs, IL–organic solvent binary systems were tested as reaction medium. THF, the best organic solvent shown in Table 1, and pyridine, which had high gastrodin solubility, were chosen as co-solvents for ILs. All the tested BF4− and PF6− based ILs can be well mixed with THF and pyridine, forming homogeneous phases. And the control experiments showed that no acylation of gastrodin occurred in any IL–organic solvent mixtures without adding the whole-cell catalysts. Results in Fig. 2A showed that all the seven IL–pyridine binary systems tested evidently improved the substrate conversion of the reaction compared to that of pure pyridine. The maximum conversion of 54.5% was obtained in [HMIM][PF6]–pyridine system, which was approximately 9 times higher than that in pure pyridine. The addition of ILs to THF, however, gave reduced substrate conversion (Fig. 2B). The minimum value was only 10.9% in [EMIM][BF4]–THF system, much lower than that in pure THF (98.0%).
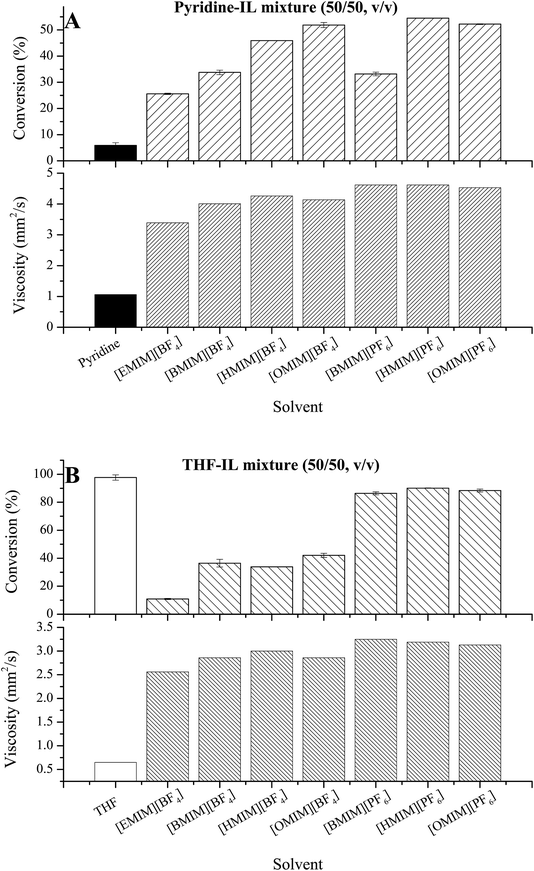 |
| Fig. 2 Effects of IL addition into pyridine (A) and THF (B) on the viscosity of the reaction systems and acylation of gastrodin. The reaction was carried out in solvent with gastrodin (20 mM), whole-cell dosage (40 mg mL−1), molar ratio of substrate to VP (1 : 30) at 40 °C and 180 rpm for 24 h. | |
As pyridine was a poor solvent for whole-cell biocatalysis (Table 1), the improved reaction efficacy by mixing pyridine with ILs as reaction media was first considered as the effect of the added ILs to the cell-bound or intracellular lipases and could be explained by the following two aspects: first, compared to pure pyridine, the number of pyridine molecules in the binary system was half of that in pure solvent, indicating that the binary system resulted in less water depletion from the surface of enzyme, leading to less inactivation of enzyme. Second, among the seven ILs added, because of the stronger hydrophobicity, the PF6− based ILs were generally more suitable for biocatalysis than BF4− based ILs. And the same anion-based ILs with long chain lengths in their cations were better for the reaction than those with short chain lengths, probably because of the increased hydrophobicity. Whole-cell catalysis differs from enzymatic catalysis in several aspects. Unlike pure enzymes, the substrate needs to be transferred from the reaction medium to cellular system to combine with enzymes, and the products have to be released from cytoplasm. During the whole process, mass transfer plays a very important role. One of the factors for mass transfer is the viscosity of the whole reaction medium. The addition of ILs increased the viscosity of reaction systems, as ILs had a higher viscosity than THF and pyridine. At first, we wondered whether the decrease in reaction efficiency by addition of ILs to THF could be the result of the increased viscosity of reaction systems. Thus, we tried to found the relation between the viscosity of different ILs and the substrate conversion. Fig. 2A and B showed a higher viscosity of PF6− based ILs than that of BF4− based ILs, but a higher substrate conversion in most of the former ILs than that in the latter. These results indicate that the solvent viscosity may affect the reactions but not the key factor decreasing the reaction efficiency in THF–IL mixtures. Other key factors for mass transfer include the permeability of cell membranes. A previous research showed that the reaction efficiency of whole-cell catalysis was usually 1–2 times slower than that of pure enzymes because of the mass transfer resistance using whole cells.25 Thus, for the impact of IL-containing system on reaction efficiency, it was hypothesized that the added ILs improved the permeability of cells by altering the cell wall and membrane, reduced mass transfer resistance and further increased the reaction efficiency. Hence, it needs further investigate the influence of ILs on the reaction and explore the permeability of cell membrane caused by organic solvent–IL binary systems.
3.4. Effect of IL concentration on Aspergillus oryzae catalyzed acylation of gastrodin
It is generally accepted that a suitable concentration of ILs is able to appropriately increase the permeability of cell membranes of microorganisms and further improve reaction efficiency.26 To further investigate the mechanism of ILs in the whole-cell catalyzed acylation, the effect of ILs content on the acylation of gastrodin was investigated by using [HMIM][PF6] as a model IL to form IL-containing systems. As displayed in Fig. 3, the increase in IL content gradually increased the viscosity of the two IL–organic solvent systems, leading to different trends in substrate conversion. In [HMIM][PF6]–THF system (Fig. 3B), the conversion decreased along with the increase in the IL content, while in [HMIM][PF6]–pyridine systems, the conversion of the reaction gradually increased from 5.9% to 84.2% (Fig. 3A). These results were almost in accordance with those observed in Fig. 2, supporting our conclusions and hypothesis that viscosity of the reaction system was not a crucial factor for the whole-cell biocatalysis. In addition, the added ILs may affect the reaction by improving the permeability of cell structure, fundamentally altering the mass transfer resistance and increasing the reaction efficiency.
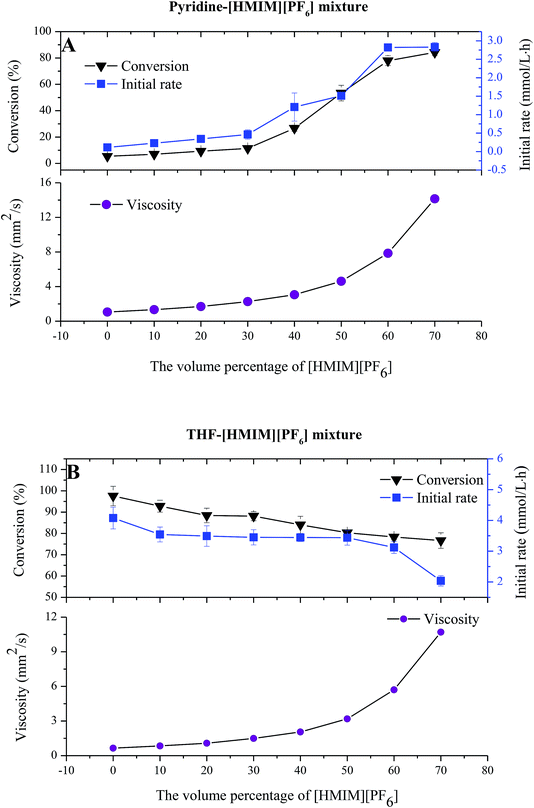 |
| Fig. 3 Effects of IL concentration in pyridine–[HMIM][PF6] (A) and THF–[HMIM][PF6] mixtures (B) on the viscosity of the reaction systems and acylation of gastrodin. The reaction was carried out in solvent with gastrodin (20 mM), whole-cell dosage (40 mg mL−1), molar ratio of substrate to VP (1 : 30) at 40 °C and 180 rpm for 48 h. | |
3.5. Effect of IL-containing system on the protein leakage of whole-cells
To further clarify the influence of ILs on the reaction, cell membrane permeability was investigated by using the protein leakage as direct indicator after cells were incubated for 72 h in different IL-containing systems. As shown in Fig. 4, the protein leakage in pure pyridine and pure THF was 591.2 μg mL−1 and 672.5 μg mL−1, respectively. Addition of ILs to either pyridine or THF resulted in a lower protein leakage (Fig. 4A and B) than that in pure pyridine or THF. This effect was associated with types of ILs. In the same anion based ILs, an increasing trend in the protein leakage was found with increase in the chain length of cations of the ILs used.
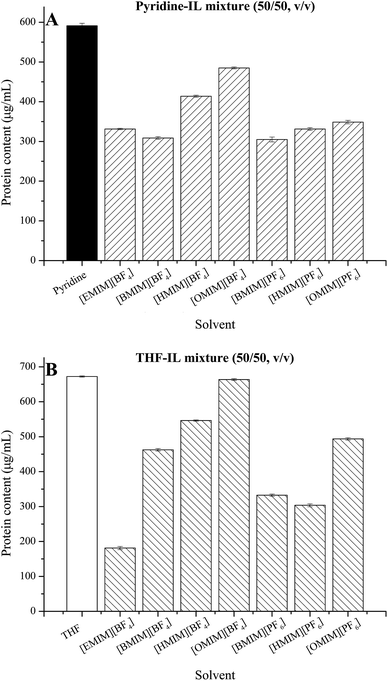 |
| Fig. 4 Effect of ILs on the protein leakage of cells in pyridine–IL (A) and THF–IL mixtures (B). | |
The results indicated that the use of pyridine and THF as reaction medium both increased the permeability of cell membranes. The mechanism underlying the significant difference in reaction efficiency between the two types of solvents, as well as the two types of IL-containing mixtures, may involve both the permeability of membranes and the effects of solvents on the cell-bound or intracellular enzymes. For the pure organic solvent systems, on one hand, lipophilic hydrocarbons accumulate in the membrane lipid bilayer, affecting the structural and functional properties of these membranes. As a result of accumulated hydrocarbon molecules, the membrane loses its integrity, and shows an increase in its permeability.27 The improved permeability of cell membrane may facilitate the mass transfer of substrates/products in/out of the cells, contacting with the enzymes. On the other hand, the increased permeability of cell membrane also facilitates the transfer of solvent molecules into the cells. When the solvents with high polarity, such as pyridine, enter into cells, they interact with the cell-bound or intracellular lipases and inactivate the enzymes by stripping off the essential waters, resulting in decreased reaction efficiency. On the contrary, less polar solvents such as THF are more compatible with the enzyme, and the biocatalytic reaction may proceed efficiently.
This proposed mechanism can be applied to the reactions performed in two types of IL–organic solvent mixtures. Many studies proved that ILs interacted with the lipid bilayer in cell membrane and even caused decrease in bilayer thickness.28–30 Moreover, this effect is related to hydrophobicity of both anions and cations of ILs,31 considering the anions, the hydrophobicity sequence was PF6− > BF4− (ref. 32) and the hydrophobicity for cations strengthened with the increase in cationic alkyl chain length (EMIM < BMIM < HMIM < OMIM). ILs has long alkyl chain is more easily to be included within the phospholipid bilayer structure due to their similar structures. However, their effects on cell membrane were lower than those of the organic solvents pyridine and THF. Thus, the cells showed lower permeability in the IL-containing systems tested than in pure pyridine or THF. For pyridine–IL solvents, the inactivation effect of pyridine is a key factor influencing the reaction efficiency. The addition of the ILs reduced the cell-membrane permeability and thus reduced the contact of pyridine molecules with cell-bound or intracellular enzymes, alleviating the inactivation effect caused by pyridine. In addition, the selected ILs bearing BF4− or PF6− anions are compatible with enzymes. Thus, all the tested IL–pyridine system improved the reaction efficiency. For the IL–THF solvents tested, the cell permeability decreased and the corresponding mass transfer resistances increased, resulting in decreased reaction efficacy.
3.6. Polysaccharide dissolving effect of IL-containing systems on the cell wall
Aspergillus oryzae, a typical filamentous fungus widely used for industrial purposes, has a supportive cell wall outside the membrane. This glycocalyx boundary comes into direct contact with the reaction solvents. Fig. 5 shows the polysaccharide dissolving effects of different IL-containing systems on cell walls by using total sugar contents of the solvent mixtures as an indicator. Similar to that observed in their effects on cell membranes, pyridine and THF both showed higher capabilities in dissolving cell walls than those of the IL-containing systems with higher reducing sugar contents of 4938.2 μg mL−1 and 4826.3 μg mL−1, respectively. Unlike the influence of IL-containing systems on the cell membrane permeability, the polysaccharide content in either THF–IL systems or pyridine–IL systems showed an approximately decreasing trend with the increased hydrophobicity of the ILs, indicating different influence mechanism of solvents on the cell wall. Generally, fungal cell walls have a thick, inner layer of polysaccharide fibers and a thin outer layer of mixed glycans. Mizutani et al. reported that the polysaccharides in the cell wall of A. oryzae composed of α-1,3-glucan, β-1,3-glucan, galactomannan, chitin, β-1,4 glucan and a small amount of proteins.33–35 Organic solvents have the capacity to dissolve polysaccharides and have been used for removing cell wall from cells.36 Dissolution of natural macromolecular polysaccharide in ILs, such as cellulose37,38 and chitosan,39 has been largely explored. These capabilities are directly correlated with hydrogen bond (H-bond) basicity and viscosity of the ILs. The ILs with high H-bond basicity resulted in high solubility of polysaccharides, because the H-bond basicity of ILs strengthened their interactions with hydroxyl groups of polysaccharide, acting as H-bond donors.32 When the chain length of alkyl groups or the symmetry of cations increased, the dissolution rate of polysaccharide components in the cell wall in IL-containing systems decreased because of the increase in viscosity and the decrease in hydrogen bond acidity.40 The main functions of polysaccharides in cell walls are protection and support. Hence, the addition of ILs, especially those with long-chain alkyl groups in cations, may reduce the interactions of glycocalyx boundary with reaction solvents, protecting the cell structures from disruption.
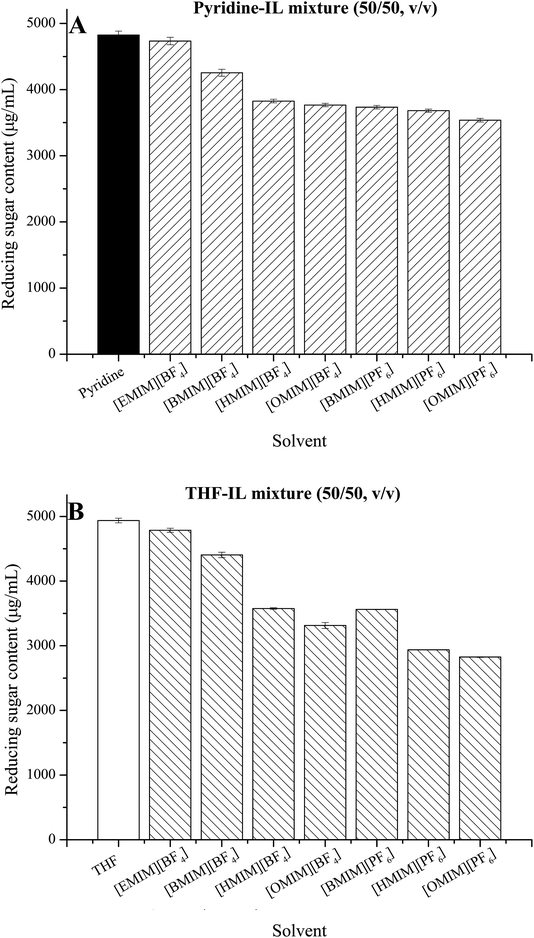 |
| Fig. 5 Effect of ILs on the polysaccharide dissolving of cells in pyridine–IL (A) and THF–IL mixtures (B). | |
3.7. Effect of ILs-containing systems on the surface morphology of Aspergillus oryzae
The leakage of cellular contents and dissolution of polysaccharides in the cell wall definitely affect the morphology of cell. Subsequently, the surface morphologies of fungal cells incubated in different binary systems were analyzed by SEM. As shown in Fig. 6 (the photos in the small windows is a partial sketch of the cell, in order to make the change in cell surface more clear), after incubation in pyridine (control) for 72 h, the cells showed severe wrinkling, while the cell incubated in THF showed less wrinkling compared to that in pyridine. The added ILs in pyridine obviously decreased the degree of wrinkling of cells (Fig. 6A1–H1), especially when incubated in pyridine–[HMIM][PF6]. The addition of ILs to THF has little effect on the cell morphologies (Fig. 6A2–H2). The SEM analysis confirmed that strongly polar organic solvents such as pyridine led to distortion of cell shape and wrinkling of cell surfaces,41 as the water molecules inside the cells gradually migrated into the polar pyridine by passive diffusion. The addition of ILs, especially the water immiscible ILs, into polar solvent increased the hydrophobicity of the whole reaction systems, reduced the water migration from cells into the outer environment and maintained the natural morphology of the cells.
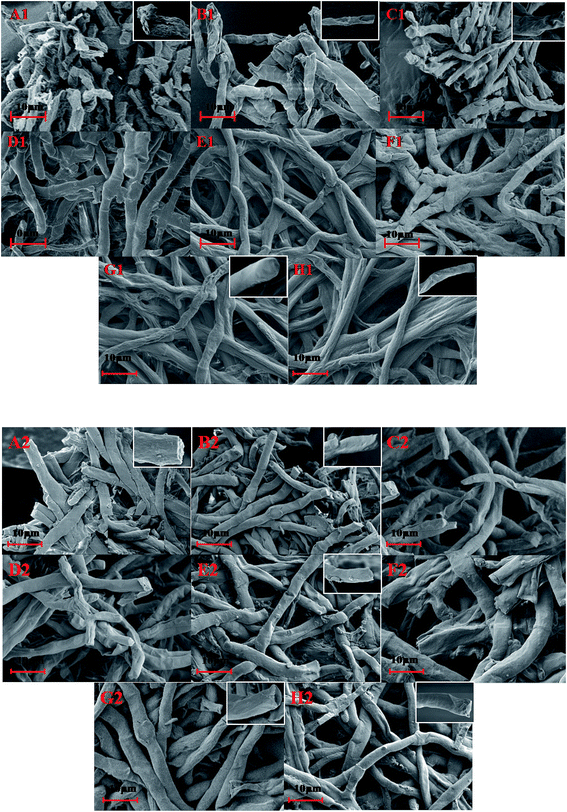 |
| Fig. 6 Effect of ILs on the morphologies of cells incubated in pyridine (A1), pyridine–[EMIM][BF4] (B1), pyridine–[BMIM][BF4] (C1), pyridine–[HMIM][BF4] (D1), pyridine–[OMIM][BF4] (E1), pyridine–[BMIM][PF6] (F1), pyridine–[HMIM][PF6] (G1), pyridine–[OMIM][PF6] (H1); THF (A2), THF–[EMIM][BF4] (B2), THF–[BMIM][BF4] (C2), THF–[HMIM][BF4] (D2), THF–[OMIM][BF4] (E2), THF–[BMIM][PF6] (F2), THF–[HMIM][PF6] (G2) and THF–[OMIM][PF6] (H2). | |
3.8. Whole cell-catalyzed acylation of gastrodin in different solvent systems
A comparison of whole-cell catalyzed acylation of gastrodin in pyridine, THF and pyridine–[HMIM][PF6] was shown in Table 2. The results clearly showed that, addition of [HMIM][PF6] to pyridine greatly increased the reaction efficiency, with the substrate conversion improved from 5.9% to 84.2%. Although the reaction efficiency in pyridine–[HMIM][PF6] is lower than that in pure THF, the solubility of gastrodin in the former is 7 times higher than that in the latter. That means the substrate concentration can be scaled up to 346.6 mmol L−1 for a homogeneous synthesis of the gastrodin esters in [HMIM][PF6]–pyridine. Interestingly, the use of pyridine–[HMIM][PF6] system also changed the selectivity of the cells, giving much higher diester selectivity of 68.3% than that in THF. Hence, pyridine–[HMIM][PF6] was also a suitable system for gastrodin modification in terms of the substrate solubility and diester selectivity. In addition, the use of pyridine–[HMIM][PF6] system reduced 70% of the volatile organic solvent as compared with the use of pure THF. Since [HMIM][PF6] was non-volatile and can be repeatedly used, the IL-containing system may be more cost-effectively and friendly to the environment.
Table 2 A comparison of acylation of gastrodin in various solvent systemsa
Solvent system |
Solubility (mmol L−1) |
V0 (mmol L−1 h−1) |
Conversion (%) |
Reaction time (h) |
Monoester selectivity (%) |
Each reaction was carried out in corresponding solvent (1 mL) with gastrodin (20 mM), Aspergillus oryzae whole-cell dosage (40 mg mL−1), VP (600 mM) at 40 °C and 180 rpm. |
THF |
47.7 ± 0.24 |
6.6 ± 0.06 |
98.0 ± 0.12 |
24 |
78.0 ± 0.02 |
Pyridine |
865.6 ± 0.62 |
0.11 ± 0.03 |
5.9 ± 0.15 |
48 |
>98 |
Pyridine–[HMIM][PF6] (30/70, v/v) |
346.6 ± 1.80 |
2.83 ± 0.17 |
84.2 ± 2.40 |
48 |
31.7 ± 0.04 |
4. Conclusions
In this study, efficient acylation of gastrodin was achieved by using Aspergillus oryzae whole-cells in THF (conversion, 98.0%) and ILs–pyridine (conversion, 84.2%) binary systems. This study not only provided a novel strategy for developing the therapeutic candidates for CNS diseases by using bioactive components of medicinal plant sources, but also offers insights into the influence of ILs on whole-cell biocatalysis. The use of different solvent systems, including organic solvents and ILs, evidently influenced the cell permeability and corresponding contact of intracellular enzymes with solvent molecules, resulting in different efficacy of reactions performed in different systems. Further investigation of the therapeutic potential of gastrodin derivatives for CNS diseases should be considered.
Conflicts of interest
None.
Acknowledgements
This work is financially supported by National Key R&D Program of China (2018YFD0400805), the National Natural Science Foundation of China (21676105, 21878107), Science and Technology Program of Guangzhou, China (201803020031), the Science and Technology Program Foundation of Guangdong Province (2015A030401025).
References
- P. Scheltens, K. Blennow, M. M. B. Breteler, B. de Strooper, G. B. Frisoni and S. Salloway, et al., Lancet, 2016, 388(10043), 505–517 CrossRef CAS.
- Collaborators GBDD, Lancet Neurol., 2019, 18, 88–106 CrossRef.
- R. Brookmeyer, E. Johnson, K. Ziegler-Graham and H. M. Arrighi, Alzheimer's Dementia, 2007, 3(3), 186–191 CrossRef PubMed.
- R. G. Canter, J. Penney and L.-H. Tsai, Nature, 2016, 539(7628), 187–196 CrossRef PubMed.
- Y. Liu, J. L. Gao, M. Peng, H. Y. Meng, H. B. Ma and P. P. Cai, et al., Front. Pharmacol., 2018, 9, 18 CrossRef PubMed.
- Z.-h. Liu, H.-t. Hu, G.-f. Feng, Z.-y. Zhao and N.-y. Mao, Sichuan Daxue Xuebao, Yixueban, 2005, 36(4), 537–540 CAS.
- M. Li and S. Qian, J. Mol. Neurosci., 2016, 60(1), 21–32 CrossRef CAS PubMed.
- R. Zhu, T.-X. Huang, X.-M. Zhao, J.-M. Zhang and P. Liang, Yaoxue Xuebao, 2014, 49(6), 800–806 CAS.
- N. N. Zhou, R. Zhu, X. M. Zhao and P. Liang, Zhonghua Binglixue Zazhi, 2016, 45(11), 780–785 CAS.
- Z.-h. Liu, H. Ma, W.-p. Wang, S.-f. Xu, L. Wang and J.-g. Shi, et al., Acta Pharm. Sin. B, 2016, 51(5), 743–748 Search PubMed.
- C. Tang, L. Wang, X. Liu, M. Cheng, Y. Qu and H. Xiao, J. Ethnopharmacol., 2015, 176, 49–54 CrossRef CAS PubMed.
- C. Tang, L. Wang, J. Li, X. Liu, M. Cheng and H. Xiao, Biomed. Chromatogr., 2015, 29(12), 1913–1920 CrossRef CAS PubMed.
- M.-M. Zheng, Y. Lu, F.-H. Huang, L. Wang, P.-M. Guo and Y.-Q. Feng, et al., J. Agric. Food Chem., 2013, 61(1), 231–237 CrossRef CAS PubMed.
- A. Guldhe, B. Singh, T. Mutanda, K. Perrnaul and F. Bux, Renewable Sustainable Energy Rev., 2015, 41, 1447–1464 CrossRef CAS.
- M. D. Patil, M. J. Dev, A. S. Shinde, K. D. Bhilare, G. Patel and Y. Chisti, et al., Process Biochem., 2017, 63, 113–121 CrossRef CAS.
- H. Gao, I. W. Kim, J. H. Choi, E. Khera, F. Wen and J. K. Lee, Biochem. Eng. J., 2015, 96, 23–28 CrossRef CAS.
- F. Yang, X. Wang, H. Tan and Z. Liu, J. Mol. Liq., 2017, 248, 626–633 CrossRef CAS.
- L. A. Gorman and J. S. Dordick, Biotechnol. Bioeng., 1992, 39(4), 392–397 CrossRef CAS PubMed.
- C. Laane, S. Boeren, K. Vos and C. Veeger, Biotechnol. Bioeng., 2009, 102(1), 2–8 CrossRef CAS PubMed.
- M.-y. Yang, H. Wu, Z.-h. Lu, X.-f. Li, F.-r. Lai and G.-l. Zhao, Bioorg. Med. Chem., 2014, 24(15), 3377–3380 CrossRef CAS PubMed.
- W. Y. Lou, M. H. Zong and T. J. Smith, Green Chem., 2006, 8(2), 147–155 RSC.
- P. Lozano, T. de Diego, J. P. Guegan, M. Vaultier and J. L. Iborra, Biotechnol. Bioeng., 2001, 75(5), 563–569 CrossRef CAS PubMed.
- H. Pfruender, R. Jones and D. Weuster-Botz, J. Biotechnol., 2006, 124(1), 182–190 CrossRef CAS PubMed.
- P. Xu, P.-X. Du, M.-H. Zong, N. Li and W.-Y. Lou, Sci. Rep., 2016, 6, 1–10 CrossRef PubMed.
- R. R. Z. Chen, Appl. Microbiol. Biotechnol., 2007, 74(4), 730–738 CrossRef CAS PubMed.
- W.-Y. Lou, W. Wang, R.-F. Li and M.-H. Zong, J. Biotechnol., 2009, 143(3), 190–197 CrossRef CAS PubMed.
- J. Sikkema, J. A. de Bont and B. Poolman, Microbiol. Rev., 1995, 59(2), 201–222 CAS.
- V. K. Sharma, S. K. Ghosh, P. Mandal, T. Yamada, K. Shibata and S. Mitra, et al., Soft Matter, 2017, 13(47), 8969–8979 RSC.
- I. Kontro, K. Svedstrom, F. Dusa, P. Ahvenainen, S. K. Ruokonen and J. Witos, et al., Chem. Phys. Lipids, 2016, 201, 59–66 CrossRef CAS PubMed.
- G. Bhattacharya, R. P. Giri, H. Saxena, V. V. Agrawal, A. Gupta and M. K. Mukhopadhyay, et al., Langmuir, 2017, 33(5), 1295–1304 CrossRef CAS PubMed.
- X. Zhang, Y. Li, H. Cao, Q. Ouyang, L. Zheng and C. Li, Chem. J. Chin. Univ., 2016, 37(10), 1870–1875 CAS.
- J.-J. Yuan, Y.-X. Guan and S.-J. Yao, ACS Sustainable Chem. Eng., 2017, 5(11), 10702–10709 CrossRef CAS.
- O. Mizutani, M. Shiina, A. Yoshimi, M. Sano, T. Watanabe and Y. Yamagata, et al., Biosci., Biotechnol., Biochem., 2016, 80(9), 1781–1791 CrossRef CAS PubMed.
- M. Bernard and J. P. Latge, Med. Mycol., 2001, 39, 9–17 CrossRef CAS PubMed.
- T. Fontaine, C. Simenel, G. Dubreucq, O. Adam, M. Delepierre and J. Lemoine, et al., J. Biol. Chem., 2000, 275(36), 27594–27607 CAS.
- X. Wang, Chinese Patent, CN203112837-U, 2013 Search PubMed.
- Y. Zhang, A. Xu, B. Lu, Z. Li and J. Wang, Carbohydr. Polym., 2015, 117, 666–672 CrossRef CAS PubMed.
- M. Hummel, C. Froschauer, G. Laus, T. Roeder, H. Kopacka and L. K. J. Hauru, et al., Green Chem., 2011, 13(9), 2507–2517 RSC.
- G. Zhao, X. Lang, F. Wang, J. Li and X. Li, Biochem. Eng. J., 2017, 126, 24–29 CrossRef CAS.
- Z. Jinming, W. Jin, Y. Jian, Z. Xiaoyu, H. Jiasong and Z. Jun, Mater. Chem. Front., 2017, 1(7), 1273–1290 RSC.
- X. Xing, X.-F. Li, X. Xiao, Y. Tang and G. Zhao, ACS Sustainable Chem. Eng., 2017, 5(11), 10662–10672 CrossRef.
Footnote |
† Electronic supplementary information (ESI) available. See DOI: 10.1039/c9ra01605h |
|
This journal is © The Royal Society of Chemistry 2019 |