DOI:
10.1039/C9RA01338E
(Paper)
RSC Adv., 2019,
9, 15448-15456
An innovative biotransformation to produce resveratrol by Bacillus safensis†
Received
22nd February 2019
, Accepted 8th May 2019
First published on 17th May 2019
Abstract
Resveratrol is considered as a potential food supplement, cosmetic ingredient and nutraceutical. In this study, resveratrol was produced by biotransformation successfully. In detail, a β-glucosidase producing strain was isolated and identified as Bacillus safensis, and it could convert polydatin to resveratrol efficiently and rapidly. Further research showed that the conversion rate to resveratrol reached 93.1% in 8 h at 37 °C. The production of resveratrol was confirmed by HPLC, LC-MS and 1H-NMR to identify its structure and it was verified to possess antibacterial properties especially against Escherchia coli. To illustrate the resveratrol transformation mechanism, several glucosidases from B. safensis CGMCC 13129 were expressed and analyzed. The results showed that BGL4 and BGL5 had higher transformation activity compared with other tested glucosidases. This research provides a novel approach to produce resveratrol, and would promote the application of resveratrol in health-promoting pharmaceutical and food products.
1. Introduction
Resveratrol (trans-3,5,4′-trihydroxystilbene), a polyphenolic compound of the stilbene family, has been found in many plants including grapes, peanuts, blueberries, cassia seed and knotweed.1,2 It has attracted a lot of attention owing to its beneficial properties,3 such as defense against pathogen infection, antitumor, anti-inflammatory, antidiabetic, antithrombotic, and antiaging.4,5 In recent years, resveratrol has been applied as an over-the-counter nutritional supplement in health products, energy drinks and cosmetics products.6 With increasing emphasis on resveratrol, the demand is expected to constantly increase in the future.
Due to its low yield in plants and high production cost, the amount of resveratrol available is clearly limited.7 At present, resveratrol is mainly produced by plant extraction, chemical synthesis and biotransformation.8 In skin and flesh of grape, the resveratrol contents were only 0.5 mg kg−1 and 0.25 mg kg−1, respectively.9 The resveratrol yield extracted from resveratrol-producing plants is usually unideal and confined by the long growth cycle of plants.10 Keylor reported a chemical synthesis to produce resveratrol. The yield was 5.1% and there were 13 linear synthetic steps in the total syntheses.11 However, the chemical synthesis is usually limited by the use of large amount of organic solvents, which not only increase the cost but also be considered as a potential threat to the environment. Besides, chemical synthesis is also hindered by poor water solubility and limited chemical stability of resveratrol.12
Owing to cost-effectiveness and environmental friendly, the most widely research on resveratrol producing is biotransformation.13 Che developed an enzymatic system for producing resveratrol from glucose and the yield was 224.4 μg L−1.14 Zhou proved polydatin in P. cuspidatum was able to be converted to resveratrol by polydatin-β-D-glucosidase.15 While β-glucosidase has the ability to hydrolyze glycosidic bonds to release glucose and glucosyl residues.16 This peculiarity allows β-glucosidase to specifically transform polydatin to resveratrol. Furtherly, microbial production possesses more advantages including rapid growth, easy of cultivation and high-level production.17 Until now, researches of microbial transformation mainly focus on grey mold for producing resveratrol.18,19 However, compared to mold, using bacterial for biotransformation may provide more excellent properties such as shorter production cycles, low cost, high conversion efficiency and easy control of reaction conditions.20 Therefore, bacteria should be considered as a potential candidate for resveratrol production.
The aim of this study was to produce resveratrol by bacteria transformation successfully. In this study, a bacteria strain which produced β-glucosidases was screened, characterized and named as B. safensis CGMCC 13129. Polydatin could be directly converted to resveratrol through enzyme produced from CGMCC 13129. The conversion activity was also analyzed in this study, and after optimized the biotransformation conditions, the conversion rate reached 93.1%. To explore the mechanism why this strain has high biotransformation activity, different glucosidases from this strain were cloned and analyzed, and two glucosidases encoded by bgl4 and bgl5 were found the more important enzymes involved in this biotransformation. This was the first report of resveratrol biotransformation by bacteria. And this research would provide significant support in developing resveratrol application though improving resveratrol yield by bacteria transformation.
2. Materials and methods
2.1 Materials, reagents and culture medium
B. safensis CGMCC 13129 was screened from planting polygonum cuspidatum soil collected from Suizhou City, Hubei Province, and isolated by dilution plate technology. E. coli JM109, E. coli BL21 (DE3) and the plasmid pET28a were all preserved in our laboratory. The screening medium contained 1% (w/v) tryptone, 0.5% (w/v) yeast extract, 1% NaCl (w/v), 0.3% geniposide (w/v, 50% purity) and 2% agar (w/v). The seed medium consisted of 1% tryptone (w/v), 0.5% yeast extract (w/v) and 1% NaCl (w/v). The transformation medium was composed of 0.5% beef extract (w/v), 1% tryptone (w/v), 0.5% NaCl (w/v) and polygonum cuspidatum (50% purity). Methanol (chromatographically purity), acetonitrile (chromatographically purity), geniposide (50%), semi-finished herbs of polygonum cuspidatum (50%), the reference substance of resveratrol (98%) were purchased from Nanjing Dilger Medical Technology Co. Ltd (Nanjing, China). All other chemicals and reagents were analytical grade and purchased from commercial sources (Sinopharm Chemical Reagent Co. Ltd.).
2.2 Strain screening
2.2.1 Screening of β-glucosidases-producing strains. Approximately 1 g of soil sample was dissolved in saline at different dilutions (10−1 to 10−7) by using ten-fold dilution method. Diluted solution (100 μL) was taken from three suitable dilutions (10−5, 10−6, 10−7) and applied onto the screening plate. We screened the bacteria using the property that blue pigment was formed by amino acids reaction with genipin, which was produced from hydrolysis of geniposide by β-glucosidases.21 After incubation for 12 h at 37 °C, the growing bacterial colonies were observed and single colonies were further purified by streak plate method.
2.2.2 Screening of β-glucosidases-producing strains with high conversion activities. Due to polydatin can be converted to resveratrol with β-glucosidase,22 we further screened to obtain the strains with high conversion activities. The bacterial were inoculated in biotransformation culture medium and then incubated at 37 °C for 12 h. The conversion rate was defined as the ratio of amount of substance of product to substrate. According to evaluate the conversion rate, strains with high conversion activity were screened out.
2.3 Classification of strain
2.3.1 Morphological identification. The β-glucosidase producing strain, which having the ability to convert polydatin to resveratrol was identified through its colony morphology property. The purified strain was spread onto the nutrient agar medium. After cultivation for 12 h, the colony morphologies were observed through Gram straining method23 by optical microscope (MODEL ECLIPSE Ci-S, Nikon, Japan) and scanning electron microscope (X-MaxN, OXFORD Instruments, UK).24
2.3.2 16S rDNA identification and phylogenetic analysis. The strain was identified with the 16S rDNA sequencing. Extracted DNA was used as template for polymerase chain reaction (PCR). The standard method presented by Sambrook25 was used for the extraction of bacterial DNA and designed PCR primers 27F (5′AGAGTTTGATCCTGGCTCAG 3′) and 1492R (5′TACGGTTACCTTGTTACGACTT 3′) for amplification of 16S rDNA gene. PCR amplification was performed according to the PrimeSTAR HS DNA polymerase protocol (TaKaRa, Dalian, China). PCR profile comprised initial denaturation at 95 °C for 5 min, followed by 30 amplification cycles of denaturation at 95 °C for 10 s, annealing at 54 °C for 45 s and extension at 72 °C for 90 s, and a final extension at 72 °C for 10 min. The purified PCR products were sequenced by Genewiz Inc. (South Plainfield, NJ, USA). Compared with sequences data from the National Center of Biotechnology information (NCBI),26 the nucleotide sequences of the isolate were checked by BLAST analysis using the NCBI.27 Its phylogeny was constructed using the neighbour-joining method28 in MEGA7.29
2.4 Biotransformation process analysis
2.4.1 Preliminary analysis. A single colony was further cultured in seed medium to logarithmic growth phase, and then transferred 2% seed liquid into biotransformation medium, cultured at 37 °C, 220 rpm. The viable cells were counted every 2 hours as well as the growth curves were plotted, and then conversion rate with time was calculated. The fermentation broth was centrifuged at 4 °C with 5000 rpm. The supernatant was removed and methanol was added for purification.30 After centrifugation, the supernatant were separated. Then methanol was distilled off using a rotary evaporator (R-300, Buchi, Switzerland), and resveratrol was obtained.
2.4.2 Cloning, expression and enzyme activity assay. The gene encoding glucosidases was amplified by standard polymerase chain reaction methods using B. safensis CGMCC 13129 genomic DNA as template. The cloning primer sequences were designed according to glucosidase gene from Bacillus safensis strain JPL_MERTA8-2 (NZ_LATH01000001.1), and were showed in Table S1.† The amplification was carried out under the following conditions: the first step was at 95 °C for 4 min; followed by 30 cycles of 95 °C for 10 s, 56 °C for 45 s, and 72 °C for 30 s; and the final extension was carried out at 72 °C for 10 min. The PCR product was gel purified and then ligated into pET28a. The recombinant plasmid pET28a-bgl was transformed into E. coli JM109 and was identified by restriction analysis and sequencing. Then the resulting vector was transformed into E. coli BL21 (DE3) for expression. Through enzyme activity assay and conversion activity assay, the key enzymes in the biotransformation process have been screened. The β-glucosidase activity was assayed using p-nitrophenyl-β-D-glucopyranoside (pNPG) as the substrate. The reaction mixture, which consisted of 1.9 mL of citric acid/phosphate buffer (200 mM Na2HPO4 and 100 mM citric acid pH 5.0, the preparation of buffer was indicated in Table S2†), 0.1 mL of enzyme sample and 1 mL of 3 mM pNPG, was incubated at 50 °C for 4 min. The reaction was terminated by adding 1 mL of 1 M Na2CO3. The absorbance of the released p-nitrophenol (pNP) was measured at 400 nm. One unit of enzyme activity was defined as the amount of enzyme that catalyzes the generation of 1 μmol of pNP per minute under the described assay conditions. Protein concentrations were determined using a BCA Protein Assay Kit with BSA as the standard. The specific activity was expressed as U mg−1. The conversion activity was assayed using polydatin as the substrate. The 3 mL reaction mixture which consisted of 5g L−1 polydatin and 10 U glucosidases was incubated at 50 °C for 30 min. The amount of resveratrol was detected by HPLC.
2.5 Product identification and analysis
2.5.1 Structure analysis. HPLC analysis was performed with an Agilent Venusil XBP column (5 μm, 4.6 × 250 nm). The column temperature was maintained at 35 °C and detection wavelength was 306 nm. The mobile phase consisted of a mixture of acetonitrile, water and phosphoric acid (40
:
59.82
:
0.18, v/v/v). The HPLC-MS was also carried on using Agilent Venusil XBP column with the same column temperature and detection wavelength as HPLC analysis. The mobile phase was composed by acetonitrile, water and formic acid (40
:
59.94
:
0.06, v/v/v). The 1H spectra were recorded in acetone-d6 (C3D6O) and in acetone-d6 with appropriate amount of D2O on a Bruker AV-500 spectrometer at working frequencies 400 MHz. Chemical shifts were corresponded with ppm (δ) values and coupling constants (J).31
2.5.2 Biological activity analysis. The crude extract of resveratrol was dissolved in methanol and sterilized by filtration through 0.22 mm Millipore filters. Antimicrobial tests were then carried out by the Oxford cup method.32 1 mL bacterial suspension (106 CFU mL−1) was injected into Petri plate with culture medium at 50 °C (20 mL per plate), then set aside until coagulation. Oxford cups (6 mm) were placed on the inoculated agar, and 100 μL of 5 mg mL−1 resveratrol was added with a micropipette. The diameter of inhibition zone (DIZ) was measured after 12 h at 37 °C, and methanol was used as the negative control. Tests were repeated three times and calculated the average of the inhibition zone diameter.
2.6 Optimization of biotransformation
To enhance the conversion rate of B. safensis CGMCC 13129, different parameters were selected for optimization, including temperatures (24 °C, 29 °C, 33 °C, 37 °C, 41 °C), pH levels (pH 5, 6, 7, 8, 9), substrate concentration of 0.10% (w/v), 0.15% (w/v), 0.20% (w/v), 0.25% (w/v), 0.30% (w/v) and conversion time (6 h, 8 h, 10 h, 12 h and 14 h).
3. Results and discussion
3.1 Strains screening and identification
From the soil samples, 32 bacteria strains which produced β-glucosidase were isolated. According to the ratio of hydrolytic zone diameter to colony diameter (H/C), 12 strains with higher β-glucosidases activity were further screened out for the conversion experiment. A strain which had the highest β-glucosidase production ability was isolated, identified and analyzed. As shown in Fig. 1(A), due to genipin generated from geniposide hydrolysis by β-glucosidase could form blue color,33 the blue color around the colony represented the ability of producing β-glucosidase. The strain exhibited the morphological characteristics such as Gram-positive, rod-shaped, spore-forming (Fig. 1(B–D)). Based on the typical features, it was suggested that this strain could be described as Bacillus. In order to identify bacterial species of the screened strains, the partial 16S rDNA gene was amplified using 27F and 1492R primer. The sequences produced were compared with sequences from NCBI using BLAST method, and showed the similarity as Bacillus safensis. The phylogenetic tree showed the closest sequences in Fig. 2. Based on homology research,34 it indicated that the strain was similar to Bacillus safensis in the evolutionary relationship and named as Bacillus safensis CGMCC 13129. The similarity of DNA sequences between B. safensis CGMCC 13129 and B. safensis NBRC 100820 was 91%. Thus it revealed that the screened strain was identified as Bacillus safensis. So far, the strain B. safensis CGMCC 13129 was the first bacteria with ability to producing-resveratrol.
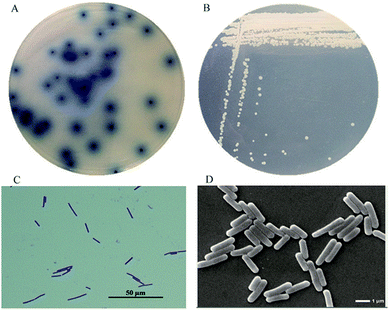 |
| Fig. 1 Morphological characteristics of CGMCC 13129. (A) Hydrolysis circle shape (B) colony morphology (C) Gram-staining analysis (D) SEM analysis. | |
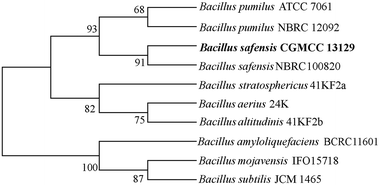 |
| Fig. 2 Phylogenetic tree. | |
3.2 Analysis of resveratrol-producing process
3.2.1 Preliminary evaluation. In order to investigate the conversion capacity of this strain which hydrolyzed polydatin to produce resveratrol, the fermentation broth of B. safensis CGMCC 13129 was observed by using microscope (Fig. 3). It was found that the turbidity of the medium changed obviously in 4 h with the number of bacteria increased rapidly. Afterwards, the turbidity changed slowly from 4 h to 12 h, while the number of aggregation compound increased significantly. These compounds distributed in fiber form initially, and then gathered into bulks. At 12 h, the large bulk was produced which should be resveratrol and it would be verified by analyzing. Besides, conversion rate and viable count was further calculated in Fig. 4. As known, the conversion capacity of bacteria is directly impacted by its number and growth state, and the vital cells count intuitively and accurately reflected the number and status of bacteria. As Fig. 4A shown, the highest conversion rate was 93% at 8 h. The number of viable bacteria significantly dropped at 6 h, while the conversion rate decreased at 8 h. The reason for this phenomenon was that the enzymes still existed in the transformation system and the conversion could be carried out. The biotransformation process was shown in Fig. 4B. And the resveratrol-containing medium was further observed by optical microscope and SEM. Fig. 5A (observed by optical microscope) and 5C (observed by SEM), which were the final state of resveratrol biotransformation, showed that some large bulks were produced and surrounded with B. safensis CGMCC 13129. The resveratrol standard was observed by microscope (Fig. 5B and D) and was similar with those producing bulks shown in Fig. 5A and C. And to explore the key enzymes in this process, glycosidases from B. safensis CGMCC 13129 were expressed and analyzed. In order to determine whether the bulk produced was resveratrol, it should be further analyzed by HPLC, LC-MS and 1H-NMR analysis.
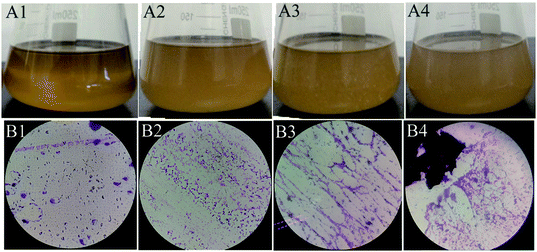 |
| Fig. 3 Observation of liquid turbidity. (A) The changes of medium turbidity at (A1) 0 h, (A2) 4 h, (A3) 8 h and (A4) 12 h. (B) The changes of producing substances at (B1) 0 h, (B2) 4 h, (B3) 8 h and (B4) 12 h. | |
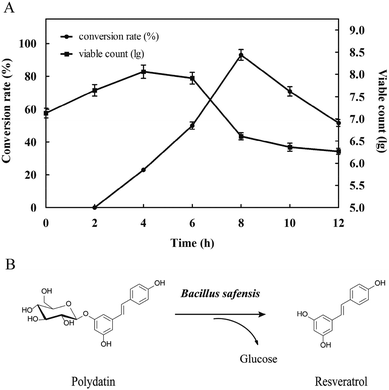 |
| Fig. 4 Analysis of resveratrol conversion. (A) The change of conversion rate and viable count with time (B) the conversion process. | |
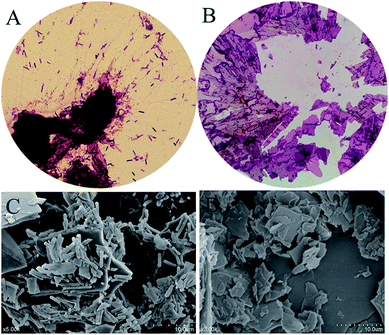 |
| Fig. 5 Observation of microscopic structure. (A) Product observed by optical microscope (B) resveratrol standard observed by optical microscope (C) product observed by SEM (D) resveratrol standard observed by SEM. | |
3.2.2 The overexpression of key enzymes. Polydatin is the glycosidic derivative of resveratrol. While enzymes such as β-glucosidase can be used to hydrolyze glucose from various aglycone to enhance the pharmacological effects of multiple chemical compounds, including resveratrol, terpenes, and volatile phenols.22,35,36 Therefore, we cloned and expressed glycosidase genes in B. safensis CGMCC 13129 to explore the key enzymes in this conversion process. The glucosidase gene was amplified from the genomic DNA of B. safensis CGMCC 13129, the gene was ligated into the expression vector pET28a, and the resulting plasmid was transformed into E. coli BL21 (DE3). The recombinant glucosidase was isolated and purified from the cultured cells. After preliminary detection, two glucosidases with higher activity were obtained. The glucosidases were analyzed by SDS-PAGE. As shown in Fig. 6, the band on the SDS-PAGE gel was observed with an apparent molecular mass of ∼62 kDa (lane 2) and ∼54 kDa (lane 3). The full-length DNA of the bgl4 gene consisted of 1608 base pairs that encoded a polypeptide of 535 amino acids. The theoretical molecular mass and isoelectric point (pI) were 61.56 kDa and 6.10, respectively. And the bgl5 gene consisted of 1407 base pairs that encoded a polypeptide of 468 amino acids, the theoretical molecular mass and pI were 54.43 kDa and 5.86, respectively. Finally, the standard assay mixture was used for the determination of β-glucosidases activity. The final specific activity of glucosidase was 8.92 U mg−1 and 6.20 U mg−1, respectively. And the conversion rate was 53% and 42%, respectively.
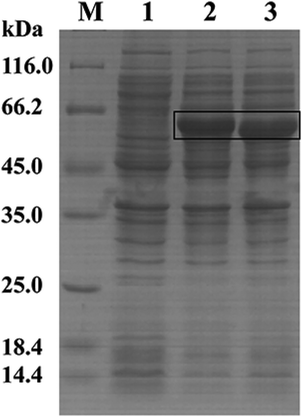 |
| Fig. 6 SDS-PAGE analysis of glucosidases lane M: protein molecular mass marker, lane 1: fragmentized liquid supernatant of E. coli BL21/pET28a, lane 2: fragmentized liquid supernatant of E. coli BL21/pET28a-bgl4, lane 3: fragmentized liquid supernatant of E. coli BL21/pET28a-bgl5. | |
3.3 Identification of products
3.3.1 HPLC, LC-MS and 1H-NMR analysis of product. Owing to high selectivity and sensitivity, HPLC and LC-MS were used for analysis of resveratrol produced by B. safensis CGMCC 13129.37 The purified samples were subjected to analysis and compared with resveratrol standard. An obvious resveratrol peak was detected at the same retention time as resveratrol standard.38 From Fig. 7(A), it was seen that the peak appeared at 5.853 min and the purity reached 99.3%. Thus it represented that resveratrol generated by bacteria transformation.
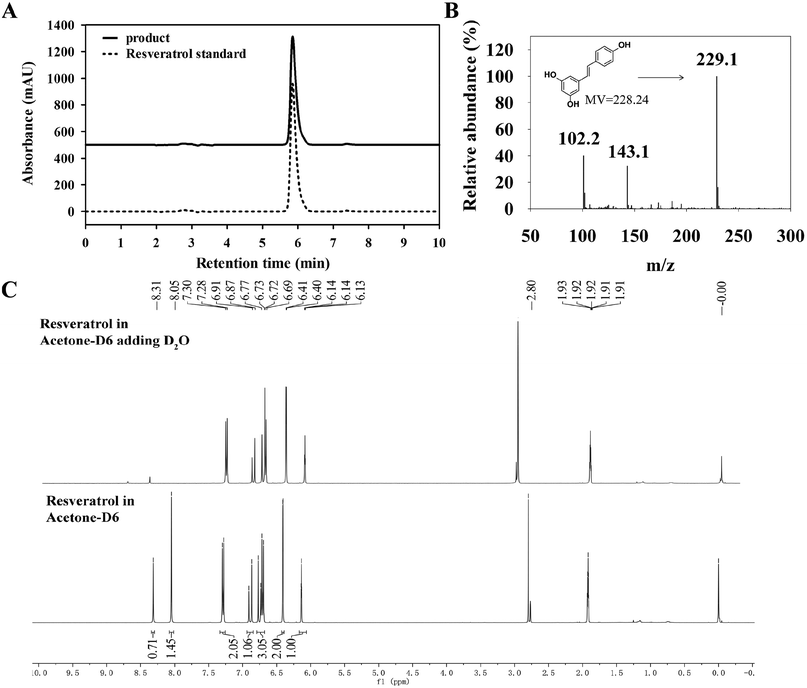 |
| Fig. 7 HPLC, LC-MS and 1H-NMR analysis of resveratrol (A) HPLC analysis of resveratrol. The elution was monitored at 306 nm at a flow rate of 1.0 mL min−1 (B) MS spectrum of resveratrol m/z 229.1 [M + H]+ (calculated for C14H13O3, 229.1) (C) 1H-NMR of resveratrol. | |
To further confirm resveratrol structure produced by B. safensis CGMCC 13129, subsequent analysis was performed with LC-MS. The compound exhibited ion peaks at m/z 102.1, 143.1 and 229.1 (Fig. 7(B)). The main peak at m/z 229.1 represented the compound was resveratrol, which was formed by acquiring a molecule from the deprotonated species [M + H]+.39 Based on these results, it indicated that resveratrol was successfully generated by bacterial biotransformation with CGMCC 13129 strain, and extraction with methanol was effective for resveratrol production.
As known, cis–trans isomers had significant differences on chemical properties, physiological activities and pharmacological effects. It was found that resveratrol derived from natural plants was described with a mixture of cis & trans structures.40 The trans-resveratrol was considered as a biologically active isomer41 and contributed to a variety of health benefits such as the antioxidant, antiaging, anti-inflammatory and anti-tumor biological properties.42 In order to investigate the structure of resveratrol produced by B. safensis CGMCC 13129, it was unambiguously confirmed by 1H-NMR analysis (Fig. 7(C)). The 1H-NMR spectra data for resveratrol was analyzed as follows: 1H-NMR (400 Hz, C3D6O): δ 6.27 (1H, d, J = 2.1 Hz), 6.54 (2H, d, J = 2.1 Hz), 6.84 (2H, d, J = 8.3 Hz), 6.89 (1H, d, J = 16.3 Hz), 7.02 (1H, d, J = 16.3 Hz) and 7.42 (2H, d, J = 8.3 Hz). Therefore, the compound was identified as trans-resveratrol and our results were similar to other studies.22,43
3.3.2 Biological activity analysis of products. Resveratrol was usually regarded as a natural antimicrobial agent.44 Therefore, the resveratrol produced by B. safensis CGMCC 13129 was used to detect its inhibitory effects on Escherichia coli, Staphylococcus aureus and Bacillus subtilis by the Oxford cup method, while the biological activity of product was evaluated. The results showed that the resveratrol had certain antibacterial activity on both Gram-positive and Gram-negative bacteria (Fig. 8). The DIZ values for all tested bacterial strains were in the range of 13.42–14.12 mm. The DIZ value of E. coli was the maximum, which represented that resveratrol produced by B. safensis CGMCC 13129 had a remarkable antibacterial effect on E. coli.
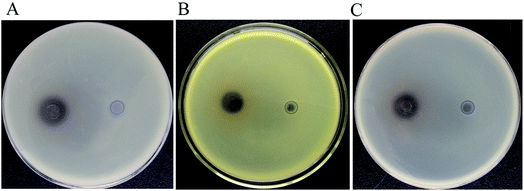 |
| Fig. 8 The inhibition zone analysis samples with resveratrol were on the left, and the controls with methanol were on the right. (A) Escherichia coli, the DIZ values was 14.12 ± 0.61 nm (B) Staphylococcus aureus, the DIZ values was 13.82 ± 0.65 nm (C) Bacillus subtilis, the DIZ values was 13.42 ± 0.59 nm. | |
3.4 Optimization of conversion conditions
A successful conversion process depended on suitable conditions.45 In order to optimize conversion process, factors such as temperature, pH, substrate concentration and reaction time were determined. The results showed that the highest conversion rate appeared at 37 °C, while lower temperature resulted in the decreasing of conversion rate due to slower rate of cell growth (Fig. 8(A)). It was obvious that pH 7 was the optimum pH and the conversion rate was 89.7%. Both alkaline and acidic conditions resulted in a lower conversion rate of resveratrol (Fig. 8(B)). It was explained that enzyme from B. safensis CGMCC 13129, which hydrolyzed polydatin to form resveratrol, mainly catalyzed by neutral enzyme and the optimum temperature of this enzyme should be 37 °C. Meantime, the highest conversion rate of 93.1% was obtained with 0.15% substrate concentration (Fig. 8(C)). The conversion obviously decreased with increasing substrate concentration after the substrate concentration was over 0.15%. It was explained that excessive substrate was negative on the growth of this bacteria. From Fig. 8(D), the conversion rate reached the highest level at 92.5% through the first 8 hours, and then declined over time. With the transformation time increasing after 8 h, the resveratrol was accumulated and adsorption between bacteria and resveratrol occurred. It was not conducive for resveratrol producing. Besides, resveratrol slightly degraded over time, which also resulted in the decrease of conversion rate. This phenomenon was also reported in Trela and Liazid's presentations.46,47 Based on these results, the resveratrol was extracted and the conversion rate reached more than 90%. While in Chen's report, the polydatin was transformed to resveratrol by piceid-β-D-glucosidase from Aspergillus oryzae sp. 100 with the conversion rate of 2 g h−1 U−1 under 60 °C, pH 5.0, 4 h.48 The biotransformation method of producing resveratrol with co-immobilized Aspergillus niger and yeast was investigated in Jin's study and the conversion rate reached about 96.7% under 30 °C, pH 6.5, 2 days.49 The conversion rate reached 85.21% under 31 °C pH 6.0 and 60 h with the method of Aspergillus oryzae negative pressure cavitation bioreactor.50 In this study, the time of conversion to resveratrol was shortened to 8 h successfully and it provided a more convenient and simple procedure, so our research provided a promising and competitive biological material for the production of resveratrol (Fig. 9).
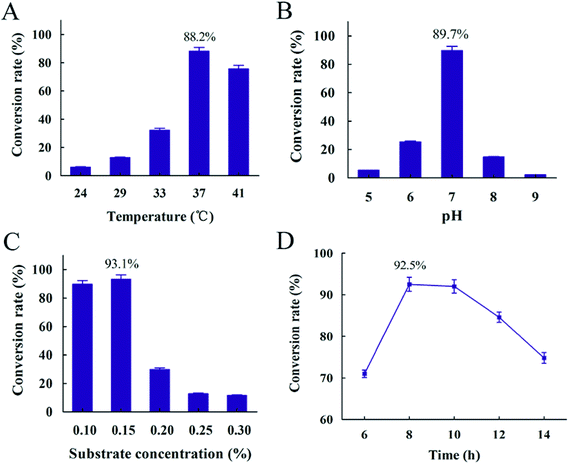 |
| Fig. 9 Conversion rate influenced by different conversion conditions (A) temperature (B) pH (C) substrate concentration (D) time. | |
4. Conclusions
This research presented that bacteria could be applied to produce resveratrol by biotransformation. In this paper, a strain which produced β-glucosidase was screened from the soil planted polygonum cuspidatum yearly. It was identified as Bacillus safensis and named as B. safensis CGMCC 13129. Based on its enzyme-specific activities and biotransformation, the resveratrol was produced efficiently. The biotransformation conditions, including temperature, pH, substrate concentration and time, were optimized. The results showed that the optimal conversion time was 8 h (37 °C, pH = 7), and the conversation rate reached 93.1% with methanol extraction. By 1H-NMR analysis, it demonstrated that B. safensis CGMCC 13129 had the ability to produce trans-resveratrol efficiently and rapidly. Our study provided innovative biological producing process for potential resveratrol application.
Conflicts of interest
There are no conflicts to declare.
Acknowledgements
This work was financially supported by The National Key Research and Development Program of China (2017YFB0308401), National Natural Science Foundation of China (Grant No. 31871741) and Public Service Platform for Selection and Fermentation Technology of Industrial Microorganisms (17PTGCCX00190).
References
- Y. Z. Mei, R. X. Liu, D. P. Wang, X. Wang and C. C. Dai, Biotechnol. Lett., 2015, 37, 9–18 CrossRef CAS PubMed.
- A. Y. Berman, R. A. Motechin, M. Y. Wiesenfeld and M. K. Holz, NPJ Precis. Oncol., 2017, 1, 35 CrossRef PubMed.
- I. Ahuja, R. Kissen and A. M. Bones, Trends Plant Sci., 2012, 17, 73–90 CrossRef CAS PubMed.
- M. Li, K. R. Kildegaard, Y. Chen, A. Rodriguez, I. Borodina and J. Nielsen, Metab. Eng., 2015, 32, 1 CrossRef PubMed.
- L. Huang, S. Zhang, J. Zhou and X. Li, RSC Adv., 2019, 9, 2572–2580 RSC.
- J. Christenson, S. J. Whitby, D. Mellor, J. Thomas, A. McKune, P. D. Roach and N. Naumovski, Metab. Syndr. Relat. Disord., 2016, 14, 323–333 CrossRef CAS PubMed.
- S. Jin, M. Luo, W. Wang, C. J. Zhao, C. B. Gu, C. Y. Li, Y. G. Zu, Y. J. Fu and Y. Guan, Bioresour. Technol., 2013, 136, 766–770 CrossRef CAS PubMed.
- A. Martínez-Márquez, J. A. Morante-Carriel, K. Ramírez-Estrada, R. M. Cusidó, J. Palazon and R. Bru-Martínez, J. Plant Biotechnol., 2016, 14, 1813–1825 CrossRef PubMed.
- M. T. Barcia, P. B. Pertuzatti, D. Rodrigues, S. Gómez-Alonso, I. Hermosín-Gutiérrez and H. T. Godoy, Food Res. Int., 2014, 62, 500–513 CrossRef CAS.
- L. Z. Kang, X. L. Zeng, Z. W. Ye, J. F. Lin and L. Q. Guo, Food Chem., 2014, 164, 211–218 CrossRef CAS PubMed.
- M. H. Keylor, B. S. Matsuura, M. Griesser, J. R. Chauvin, R. A. Harding, M. S. Kirillova, X. Zhu, O. J. Fischer, D. A. Pratt and C. R. Stephenson, Science, 2016, 354, 1260 CrossRef CAS PubMed.
- L. Hai, D. He, X. He, K. Wang, X. Yang, J. Liu, H. Cheng, X. Huang and J. Shangguan, J. Mater. Chem. B, 2017, 5, 29 Search PubMed.
- S. Jin, B. Yang, Y. Cheng, J. Tan, H. Kuang, Y. Fu, X. Bai, H. Xie, Y. Gao and C. Lv, Food Bioprod. Process., 2017, 102, 177–185 CrossRef CAS.
- J. Che, J. Shi, Z. Gao and Y. Zhang, J. Microbiol. Biotechnol., 2016, 26, 1348 CrossRef CAS PubMed.
- L. Zhou, S. Li, T. Zhang, W. Mu and B. Jiang, J. Sci. Food Agric., 2016, 96, 2588–2595 CrossRef CAS PubMed.
- J. R. K. Cairns and A. Esen, Cell. Mol. Life Sci., 2010, 67, 3389–3405 CrossRef PubMed.
- J. Wu, P. Liu, Y. Fan, H. Bao, G. Du, J. Zhou and J. Chen, J. Biotechnol., 2013, 167, 404–411 CrossRef CAS PubMed.
- B. Houillé, N. Papon, L. Boudesocque, E. Bourdeaud, S. Besseau, V. Courdavault, C. Enguehardgueiffier, G. Delanoue, L. Guérin and J. P. Bouchara, J. Nat.
Prod., 2014, 77, 1658–1662 CrossRef PubMed.
- M. Salgado, S. Rodríguez-Rojo, F. M. Alves-Santos and M. J. Cocero, J. Food Eng., 2015, 165, 13–21 CrossRef CAS.
- F. Lai, Y. E. Miao, Y. Huang, Y. Zhang and T. Liu, ACS Appl. Mater. Interfaces, 2015, 8, 3558–3566 CrossRef PubMed.
- S. Fujikawa, Y. Fukui, K. Koga and J. i. Kumada, J. Ferment. Technol., 1987, 65, 419–424 CrossRef CAS.
- A. Todaro, R. Palmeri, R. N. Barbagallo, P. G. Pifferi and G. Spagna, Food Chem., 2008, 107, 1570–1575 CrossRef CAS.
- K. Minamida, M. Tanaka, A. Abe, T. Sone, F. Tomita, H. Hara and K. Asano, J. Biosci. Bioeng., 2006, 102, 247–250 CrossRef CAS PubMed.
- J. Hong, W. Guan, G. Jin, H. Zhao, X. Jiang and J. Dai, Microbiol. Res., 2015, 170, 69–77 CrossRef CAS PubMed.
- J. Sambrook, E. F. Fritsch and T. Maniatis, Molecular Cloning: A Laboratory Manual, Cold Spring Harbor Laboratory Press, 1989 Search PubMed.
- E. W. Sayers, T. Barrett, D. A. Benson, E. Bolton, S. H. Bryant, K. Canese, V. Chetvernin, D. M. Church, M. DiCuccio and S. Federhen, Nucleic Acids Res., 2012, 40, D13–D25 CrossRef CAS PubMed.
- S. F. Altschul, T. L. Madden, A. A. Schäffer, J. Zhang, Z. Zhang, W. Miller and D. J. Lipman, Nucleic Acids Res., 1997, 25, 3389–3402 CrossRef CAS PubMed.
- N. Saitou and M. Nei, Mol. Biol. Evol., 1987, 4, 406 CAS.
- S. Kumar, G. Stecher and K. Tamura, Mol. Biol. Evol., 2016, 33, 1870–1874 CrossRef CAS PubMed.
- D. G. Wang, W. Y. Liu and G. T. Chen, J. Pharm. Anal., 2013, 3, 241–247 CrossRef CAS PubMed.
- H. E. Gottlieb, V. Kotlyar and A. Nudelman, J. Org. Chem., 1997, 62, 7512–7515 CrossRef CAS PubMed.
- G. Zhang, M. Hu, L. He, P. Fu, L. Wang and J. Zhou, Food Bioprod. Process., 2013, 91, 158–168 CrossRef CAS.
- A. S. Bellé, C. R. Hackenhaar, L. S. Spolidoro, E. Rodrigues, M. P. Klein and P. F. Hertz, Food Chem., 2018, 246, 266–274 CrossRef PubMed.
- A. Levican, S. Rubio-Arcos, A. Martinez-Murcia, L. Collado and M. J. Figueras, Syst. Appl. Microbiol., 2015, 38, 30–35 CrossRef CAS PubMed.
- B. Y. M. S and B. VS, Crit. Rev. Biotechnol., 2002, 22, 375–407 CrossRef PubMed.
- P. González-Pombo, L. Fariña, F. Carrau, F. Batista-Viera and B. M. Brena, Process Biochem., 2011, 46, 385–389 CrossRef.
- L. Jaitz, K. Siegl, R. Eder, G. Rak, L. Abranko, G. Koellensperger and S. Hann, Food Chem., 2010, 122, 366–372 CrossRef CAS.
- S. Y. Shin, N. S. Han, Y. C. Park, M. D. Kim and J. H. Seo, Enzyme Microb. Technol., 2011, 48, 48–53 CrossRef CAS PubMed.
- Y. J. Jeong, S. G. Woo, C. H. An, H. J. Jeong, Y. S. Hong, Y. M. Kim, Y. B. Ryu, M. C. Rho, W. S. Lee and C. Y. Kim, Mol. Cells, 2015, 38, 318 CrossRef CAS PubMed.
- M. H. Keylor, B. S. Matsuura and C. R. Stephenson, Chem. Rev., 2015, 115, 8976–9027 CrossRef CAS PubMed.
- S. W. Tsang, H. Zhang, Z. Lin, H. Mu and Z. X. Bian, Biomed. Pharmacother., 2015, 71, 91–97 CrossRef CAS PubMed.
- A. Ahmad and R. Ahmad, Chem. Biol. Interact., 2014, 221, 1 CrossRef CAS PubMed.
- C. Caddeo, M. Manconi, M. C. Cardia, O. Diez-Sales, A. M. Fadda and C. Sinico, Int. J. Pharm., 2015, 484, 138–145 CrossRef CAS PubMed.
- L. Paulo, S. Ferreira, E. Gallardo, J. A. Queiroz and F. Domingues, World J. Microbiol. Biotechnol., 2010, 26, 1533–1538 CrossRef CAS.
- H. P. Kuo, R. Wang, Y. S. Lin, J. T. Lai, Y. C. Lo and S. T. Huang, Bioresour. Technol., 2017, 243, 986–993 CrossRef CAS PubMed.
- A. Liazid, M. Palma, J. Brigui and C. G. Barroso, J. Chromatogr. A, 2007, 1140, 29–34 CrossRef CAS PubMed.
- B. C. Trela and A. L. Waterhouse, J. Agric. Food Chem., 1996, 44, 1253–1257 CrossRef CAS.
- M. Chen, D. Li, Z. Gao and C. Zhang, Bioproc. Biosyst. Eng., 2014, 37, 1–6 CrossRef.
- S. Jin, M. Luo, W. Wang, C. J. Zhao, C. B. Gu, C. Y. Li, Y. G. Zu, Y. J. Fu and Y. Guan, Bioresour. Technol., 2013, 136, 766–770 CrossRef CAS PubMed.
- J. Shuang, B. Yang, Y. Cheng, J. Tan, H. Kuang, Y. Fu, X. Bai, H. Xie, G. Yuan and L. Chen, Food Bioprod. Process., 2016, 102, 177–185 Search PubMed.
Footnote |
† Electronic supplementary information (ESI) available. See DOI: 10.1039/c9ra01338e |
|
This journal is © The Royal Society of Chemistry 2019 |