DOI:
10.1039/C9RA00742C
(Paper)
RSC Adv., 2019,
9, 11160-11169
Novel synthesis of a clay supported amorphous aluminum nanocomposite and its application in removal of hexavalent chromium from aqueous solutions†
Received
28th January 2019
, Accepted 1st April 2019
First published on 9th April 2019
Abstract
A bentonite supported amorphous aluminum (B–Al) nanocomposite was synthesized by the NaBH4 reduction method in an ethanol–water interfacial solution and characterized with SEM, TEM, XRD, FT-IR and XRF. Surface morphology and line scans obtained from TEM imaging suggest the successful synthesis of the nanocomposite while XRF data shows a drastic change in Al concentration in the synthesized nanocomposite with respect to raw bentonite. This synthesized nanocomposite was further utilized for the removal of hexavalent chromium (Cr(VI)) from aqueous solutions. The very high removal efficiency of the composite for Cr(VI) (i.e. 49.5 mg g−1) was revealed by the Langmuir sorption isotherm. More than 90% removal of Cr(VI) in just 5 minutes of interaction suggests very fast removal kinetics. Inner sphere complexation and coprecipitation of Cr(VI) can be concluded as major removal mechanisms. No influence of ionic strength suggests inner sphere complexation dominated in Cr(VI) uptake. pH of the solution didn't influence the sorption much but comparatively the removal was higher under alkaline conditions (99.4%) than under acidic conditions (93.7%). The presence of humic acid and bicarbonate ions reduced the sorption significantly. The final product, Cr–Al(OH)3 results in precipitation by forming alum which indicates that clay supported amorphous aluminum nanocomposites can be considered as potential sorbents for toxic metal ions in the environment.
1. Introduction
Toxicity arises in living organisms if heavy metals are present above their permissible concentrations and can damage the whole ecosystem due to their persistency and non-degradability.1–3 Increased concentrations of these metal ions in water bodies are mainly attributed to anthropogenic sources.4
Out of several toxic metal ions, chromium is one of the most extensively used heavy metals in various industrial activities like in dyes and pigments, electroplating, wood preservation, corrosion inhibition, textiles, copying machine toner, fertilizer manufacture, etc.5,6 The maximum permissible limit for chromium has been set at 0.1 mg L−1 by the United States Environmental Protection Agency (USEPA) while the World Health Organization (WHO) and the Bureau of Indian Standards (BIS) set the maximum permissible limit of chromium in drinking water at 0.05 mg L−1.7–9 Out of the environmentally relevant forms of Cr, Cr(III) is an essential micronutrient, playing a role in glucose metabolism; in contrast, Cr(VI) is considered as a carcinogen (through inhalation) and is associated with both acute and chronic health effects (by both inhalation and ingestion).10
Various removal mechanisms have been reported for chromium remediation like chemical precipitation, oxidation/reduction, membrane filtration, ion-exchange and adsorption, among all sorption is a simple, effective, and economical method for contaminant remediation.11–13 Many adsorbents have been synthesized so far and used to remove chromium.13–22 For example, recently, nano zero valent iron (nZVI) has been explored for the removal of chromium23–26 but intense oxidation, iron release and immediate aggregation are the limitations of utilizing nZVI.27 Various composites of aluminium (Al2O3, γ-Al2O3, Al(OH)3 etc.) have been used for the remediation of Cr(VI) in the environment but only physical sorption resulted in almost complete desorption in just 3 hours.28,29
Here, for the first time, we have synthesized amorphous aluminium by NaBH4 reduction method and supported them on bentonite clay to prevent further aggregation of smaller particles and hence to have very high reactivity. Novel clay supported amorphous aluminium was characterized by XRD, TEM-EDS, FT-IR, XRF etc. The synthesized novel nanocomposite was further utilized for removal of Cr(VI) from aqueous solution. Adsorption kinetics and isotherms were studied to elucidate the sorption behaviour of this novel nanocomposite. Various factors which can influence the sorption like pH, temperature, ionic strength have been studied. Effect of complexing agents (bicarbonates and humic acid) on removal of Cr(VI) have also been explored in detail. After systematic and comparative experiments, reaction precipitate was collected, characterized and analysed by FT-IR and XRD to clarify the possible Cr(VI) removal mechanisms. Finally this study proposes a new, cost effective and novel nanocomposite for the removal of Cr(VI) and other heavy metal ions in the environment.
2. Materials and methods
2.1 Materials and chemicals
Extra pure bentonite clay with the composition (in wt%) – 54.9% SiO2, 19.2% Al2O3, 8.3% Fe2O3, 1.99% MgO, 0.17% CaO, 1% K2O and 3.79% Na2O was obtained from Loba chemie Pvt. Ltd. and used for experiments without further processing. Following chemicals of analytical purity were purchased from Merck-Ethanol, NaBH4, K2Cr2O7, NaCl, KCl, CaCl2, MgCl2, AlCl3, HCl, NaOH, NaHCO3. Humic acid was obtained from Sigma-Aldrich. All solutions were prepared with 18.2 MΩ Milli-Q water.
2.2 Synthesis of clay supported amorphous aluminium
Bentonite and aluminum were chosen due to their very high abundance on Earth's crust. While NaBH4 was chosen as reducing agent and also to minimize Al oxidation during synthesis.
Briefly bentonite clay and aluminum salt (AlCl3) were taken with a clay/Al mass ratio of 1
:
1 in 50 ml interfacial solution (ethanol + water = 4
:
1) and sonicated for 30 minutes then under continuous magnetic stirring, 100 ml of 0.93 M NaBH4 was then added dropwise with a constant flow rate of 4.5 ml min−1 using peristaltic pump (Masterflex). After complete addition of NaBH4, mixtures were vigorously stirred for another 20 minutes and cream coloured product was filtered and washed thoroughly 5 times with absolute ethanol. Obtained material was vacuum dried in an oven, powdered and stored for further characterization and sorption experiments. Al3+ has very high ionic potential and hence can easily be exchanged with other ions present in interlayer spaces of clay, during initial sonication and magnetic stirring. Oxidation will be minimum in these spaces due to hindrance.30,31
2.3 Characterizations and measurements
Field emission scanning electron microscope (FESEM) images were obtained using Carl Zeiss SUPRA 55VP FESEM. Transmission electron microscopy (TEM) images were obtained by JEOL, JEM 2100 HR model. Energy dispersive analysis of X-ray (EDAX) studies were performed with the Oxford Instruments X-Max with INCA software coupled to both FESEM and TEM. Zeta potentials and pHPZC in aqueous suspension solution were measured with a Malvern Zetasizer ZS-90 dynamic light scattering (DLS) instrument. X-ray diffraction (XRD) measurements were performed with a Rigaku (mini flex II, Japan) powder X-ray diffractometer having a high-power Cu-Kα radioactive source (wavelength = 0.154 nm) at 40 kV/70 mA. All samples were scanned from 5° to 90° 2θ range at a scanning rate of 3° 2θ per minute. The Fourier transform infrared (FTIR) spectroscopy studies were carried out with a PerkinElmer spectrum RX1 with KBr pellets. The residual Cr(VI) in solution was analyzed using the 1,5-diphenylcarbazide method with a UV-Visible spectrophotometer (Evolution 201, Thermo-Fisher Scientific) at wavelength of 540 nm. Aluminum concentration in raw bentonite and synthesized nanocomposite was determined by fusion beads X-ray fluorescence spectroscopy (S8 TIGER 4 kW, Bruker).
2.4 Batch sorption experiments and control
Batch experiments were carried out under room temperature. For chromium removal, based on the solid to solution ratio sorption experiment (Fig. S1†), 15 mg of nanocomposite (m) was added in 25 ml (v) of 10 ppm chromium aqueous solution (C0) in 50 ml centrifuge tubes at circumneutral pH condition. Reaction mixtures were placed on a shaker at 200 rpm for 24 h at 25 °C, then the mixtures were filtered using 0.45 μm filter paper (Merck Millipore) and elute were analysed for residual chromium concentrations (Ce) using UV-Vis spectrophotometer (Calibration curve attached Fig. S2†). To assure QA/QC of obtained data, controlled experiments were carried out in parallel by providing similar conditions but without adding nanocomposite and blanks were run without adding chromium in the aqueous solutions. All experiments were carried out in duplicates and relative error was less than 5%, hence error bars are eliminated for better visualization of the data. Removal (%) and sorption capacity (q) was calculated as
3. Results and discussions
3.1 Nanocomposite characterization
The surface morphology of bentonite clay was characterized by both scanning electron microscopy (SEM) and transmission electron microscopy (TEM). TEM imaging, HRTEM and line scanning was also performed for the characterization of synthesized nanocomposite. Clay flakes of less than 2 μm are clearly visible in both SEM and TEM images in Fig. 1a and b respectively, while spherical shaped or amorphous Al nanoparticles on the surface of bentonite can be seen in TEM images of nanocomposite (Fig. 1c and d). Diameter of these spherical shaped, non-crystalline or amorphous Al nanoparticles on clay surface is roughly around 12–18 nm. Particles are homogeneously dispersed on the surface of clay which also helps in preventing the aggregation of these particles.
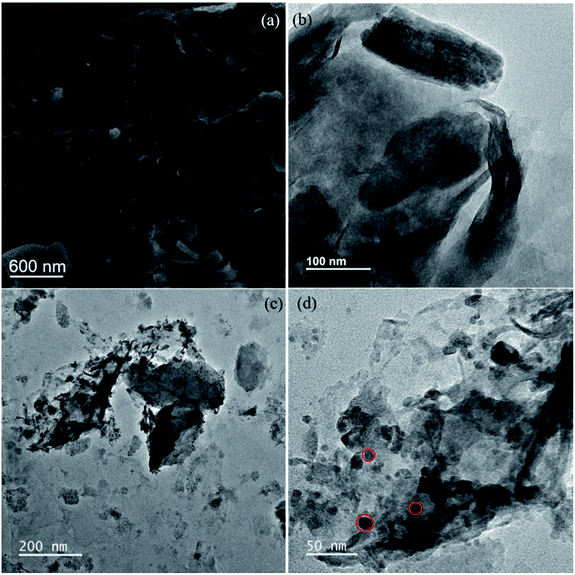 |
| Fig. 1 (a) SEM image of bentonite clay showing <2 μm clay flakes, TEM images of (b) bentonite, (c and d) synthesized B–Al composite (red circles highlighting amorphous Al on clay surface). | |
XRF data (Table 1) shows a drastic increase in Al content and TEM line scan shows increase in Al intensity along these spheres (Fig. S3†). Results suggests a successful synthesis and supporting of amorphous aluminum.
Table 1 XRF data for major elements concentrations in bentonite and B–Al nanocomposite
Oxides (%) |
SiO2 |
TiO2 |
Al2O3 |
Fe2O3 |
MnO |
MgO |
CaO |
Na2O |
K2O |
P2O5 |
Bentonite |
54.91 |
1.828 |
19.24 |
8.3 |
0.06 |
1.99 |
0.17 |
3.79 |
1.01 |
0.089 |
B–Al |
10.09 |
0.3082 |
39.2 |
1.39 |
0 |
0.36 |
0.01 |
2.78 |
0.18 |
0.0182 |
Fig. 2 shows the FTIR patterns of the prepared nanocomposite. Raw bentonite, B–Al, B–Al–Cr, all composites have broad peaks around 3465 cm−1 which can be attributed to the OH group stretching while broad bands around 558 cm−1 were related to Al–O stretching vibrations. Si–O bending vibration peak was around 1044 cm−1 as shown in Fig. 2.32,33 Antisymmetric stretching vibration of Si–O–Si in the bentonite caused absorption peak near 797 cm−1. The absorption peak near 3626 cm−1 is the vibratory absorption peak of the hydroxyl group. A vibrational absorption peak of O–H was at 1632 cm−1, which reflected crystal water in the bentonite lattice.34–38 FT-IR data for B–Al–Cr precipitate gives a new absorption peak in 515–550 cm−1 region (525 cm−1) which corresponds to Cr2O42− suggests Cr(OH)3 formation which suggests the reduction of Cr(VI) to Cr(III) as a probable removal mechanism.39,40
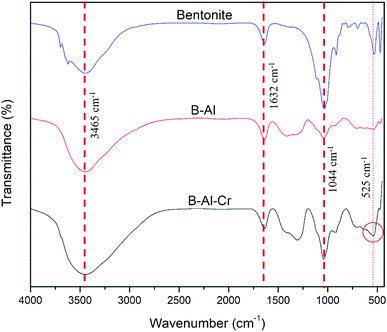 |
| Fig. 2 FT-IR spectra of bentonite, synthesized B–Al nanocomposite and B–Al–Cr reaction precipitate. | |
X-ray diffraction patterns of bentonite, B–Al composite and B–AL–Cr precipitate are shown in Fig. 3. The data obtained for all materials shows the peak at 19.7° which is of sodium bentonite, and the bentonite is 2
:
1 of the mineral structure. The peaks at 21.9° and 26.6° represent quartz. The peak at 27.8° stands for the feldspar.41,42 All these peaks are present in B–Al and B–Al–Cr, which reveals no change in clay composition after the interaction. As amorphous nature of aluminum can be revealed by TEM images (Fig. 1c and d), obtained XRD peaks in case of B–Al composite can be correlated with cationically exchanged metal ions with Al3+ and reduced by NaBH4 which can be supported with XRD data of B–Al–Cr where the surface has Al(OH)3–Cr coprecipitate and hence shows no peaks other than of bentonite.
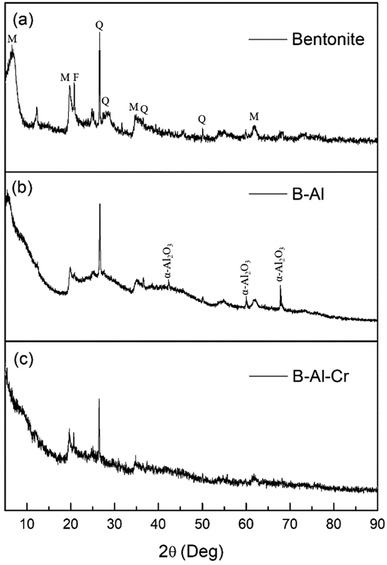 |
| Fig. 3 X-ray diffractogram for (a) bentonite, (b) B–Al nanocomposite and (c) B–Al–Cr precipitate (M = montmorillonite, Q = quartz, F= feldspar). | |
Presence of new peaks in XRD of B–Al composite corresponds to Al2O3, suggests presence of an oxide layer on aluminum supported on bentonite clay. Crystallite size obtained from XRD data at 60° 2-theta peak was almost equal to 27 nm while TEM image shows multiple 12–18 nm sized particles.
3.2 Cr(VI) sorption on nanocomposite
3.2.1 Sorption kinetics. Experiment was performed to determine the removal kinetics and the data in Fig. 4 shows very fast removal of Cr(VI) in the solution as more than 90% of chromium was removed within 5 minutes of reaction. Equilibrium was achieved in 20 minutes of reaction.
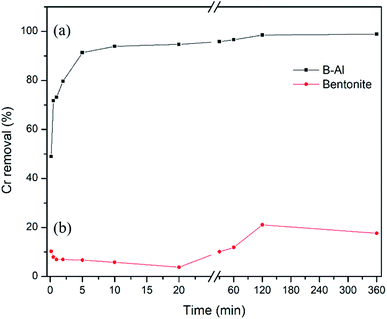 |
| Fig. 4 Effect of time on sorption of Cr(VI) on (a) raw bentonite and (b) B–Al nanocomposite. | |
To get insights in removal mechanism, pseudo first order, pseudo second order and intraparticle diffusion modelling was performed (Fig. 5a, b and c respectively). Linear form of pseudo first-order reaction kinetics equation, proposed by Lagergren was used to model the Cr(VI) removal kinetics. Used equation was as follows
ln(qe − qt) = ln qe − k1t |
where
qe and
qt (mg g
−1) are the sorption capacities at equilibrium and at time
t (min), respectively.
k1 is the pseudo-first-order reaction rate constant (min
−1) which can be obtained from the slope of the graph between ln(
qe −
qt) and
t. Intercept provide the calculated sorption capacity. Data fit was poor as
R2 = 0.90 (
Fig. 5a) and calculated sorption capacity (
Table 2) was very different than experimentally obtained sorption capacity which suggests sorption doesn't follow the first order reaction kinetics.
43
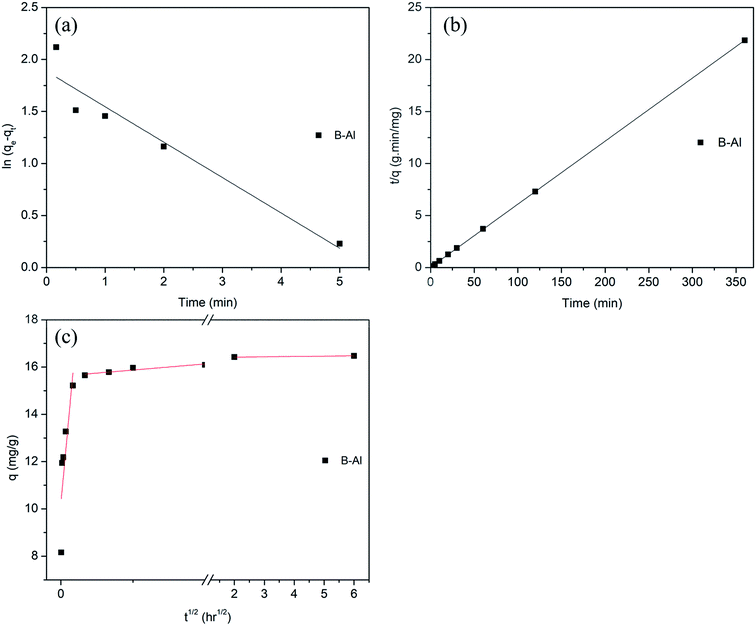 |
| Fig. 5 Various reaction kinetics models (a) pseudo first order, (b) pseudo second order and (c) intra particle diffusion model. | |
Table 2 Obtained parameters for various reaction kinetics models
Model |
K |
Q (mg g−1) |
R2 |
Intercept |
Pseudo first order |
0.35 min−1 |
6.55 |
0.90 |
|
Pseudo second order |
0.102 g mg−1 min−1 |
16.49 |
0.99 |
|
Intraparticle diffusion |
0.52 mg g−1 h0.5 |
|
0.84 |
15.61 |
To analyze the chemisorption kinetics, pseudo second order kinetic equation44 was applied
where
k2 is the second order reaction rate constant for pseudo second-order sorption (g mg
−1 min
−1). The plot
t/
qt vs. t is linear and can be used to obtained calculated sorption capacity and
k2. As seen in plot, data show excellent linear fit (
R2 = 0.99) and calculated
q matches with experimental
q (
Table 2). It shows that sorption follows second order kinetics which suggests chemisorption as a probable removal mechanism.
45 Data for both kinetic the models are shown in
Table 2.
Intra particle diffusion modelling. Webber's pore-diffusion model (intra particle diffusion) is based on Fick's second law of diffusion and linear form46 is as follows
Where ki (mg g−1 h0.5) is intra particle diffusion rate constant and c signify resistance in mass transfer due to boundary layer. As shown in Fig. 5c, the plot shows three linear portions which confirm that the external (bulk) mass transfer at the initial stage which is followed by intra particle pore diffusion into macro, meso and micro pores. These three plots can be related to sorption process as follows(1) film or surface diffusion where the sorbate is transported from the bulk solution to the external surface of sorbent, (2) intraparticle or pore diffusion, where sorbate molecules move into the interior of sorbent particles, and (3) adsorption on the interior sites of the sorbent. It can be seen that adsorption is very rapid and hence it can be assumed that it does not influence the overall removal kinetics. Therefore, surface diffusion or intraparticle diffusion will be the dominant sorption process.47
As shown in Fig. 5c, first linear segment which is very steep shows the surface or film diffusion while second gentle slope represents gradual adsorption stage where intraparticle diffusion was rate limiting step and third horizontal line represent final equilibrium. As the curve is not starting from origin, it can be inferred that intraparticle diffusion was not the only rate limiting step but out of these three steps controlling adsorption, intraparticle diffusion was rate limiting. Data for intercept value and intraparticle diffusion rate constant was also given in Table 2.
3.2.2 Adsorption isotherms. Data obtained for both Langmuir and Freundlich isotherms (detailed equations in ESI†) Fig. 6 a and b shows good fit (R2 = 0.99 and 0.98 respectively).
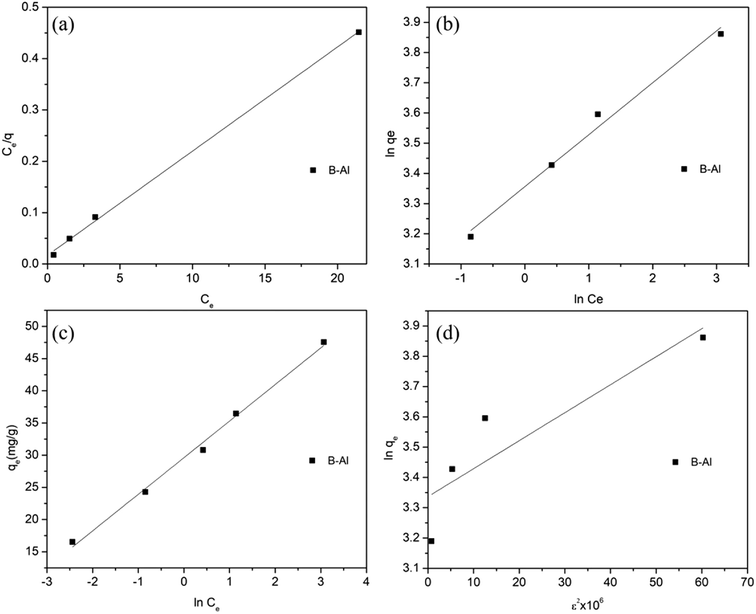 |
| Fig. 6 Various sorption isotherms, (a) Langmuir, (b) Freundlich, (c) Temkin and (d) DKR. | |
Maximum sorption capacity obtained from Langmuir isotherm was 49.5 mg g−1 while the value of ‘n’ obtained by Freundlich isotherm (Table 3) was 5.81. n > 1 suggests strong sorbate–sorbent interaction and chemisorption as removal mechanism. More perfectly following Langmuir isotherm suggests monolayer sorption with uniform sorption affinity of surface.
Table 3 Obtained parameters for various sorption isotherms
Sorption isotherm model |
Parameters |
Langmuir |
KL = 1.19 L mg−1 |
qmax = 49.5 mg g−1 |
R2 = 0.99 |
Freundlich |
KF = 28.68 |
n = 5.81 |
R2 = 0.98 |
Temkin |
b = 0.44 kJ mol−1 |
KT = 0.18 L g−1 |
R2 = 0.99 |
DKR |
E = 134.16 kJ mol−1 |
Xm = 28.1 mg g−1 |
R2 = 0.70 |
Temkin isotherm (Fig. 6c, Table 3) assumes chemisorption as the removal process and quite well fit (R2 = 0.99) again proves the chemisorption as Cr(VI) removal mechanism.48
Data (Fig. 6d, Table 3) reveals that maximum sorption capacity obtained through DKR isotherm is not matching with qmax obtained through Langmuir isotherm and data fit was also poor (R2 = 0.70) which suggests that sorption is not following DKR isotherm. It reveals that this sorption is not a physical process.
3.2.3 Effect of ionic strength. Ionic strength widely used as a parameter to distinguish metal-adsorbent binding i.e. outer vs. inner sphere complexation. Other ions in the solution generally induce site competition. Outer sphere complexes are chiefly electrostatic in nature while inner sphere complexes have covalent characteristics.49,50 Compared to inner sphere complexes, outer sphere complexes are more vulnerable site competition. This is because in outer sphere complex, during complex formation, there is retainment of coordinated water by adsorbed ions, surface functional groups or by both. On the other hand, in an inner sphere surface complex, water is replaced by the surface functional groups with higher coordination affinity, so that immediately adjacent interacting sites can result in formation of electron cloud overlapping to build a strong covalent bond.51,52 In this presented study, effect of mono, di and trivalent cation induced ionic strength was evaluated and data shown in Fig. 7 suggests that there is almost no effect of ionic strength on Cr(VI) removal which strongly suggests inner sphere complexation as a probable removal mechanism.
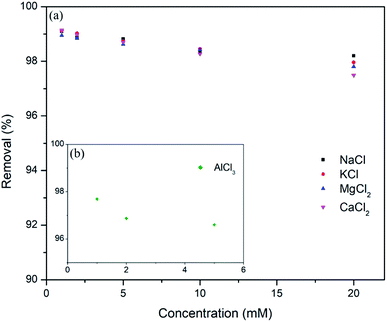 |
| Fig. 7 Effect of ionic strength induced by (a) mono and divalent cations and (b) trivalent cation on removal of Cr(VI). | |
3.2.4 Effect of pH. Solution pH is the critical factor which not only influences the form of functional groups on the surface of adsorbents but also dramatically changes the speciation of the metal ions (Fig. S4†).53 Both the factors can change the adsorption performance. Effect of pH was studied over a wide range of pH (3–10) but no such drastic change in sorption capacity was observed (Fig. 8). A slow decrease in acidic environment and increase in alkaline environment in sorption capacity was there which can be related to the metal ion leaching in acidic environment and dominating Cr(OH)3 amorphous chromium specie in alkaline environment.
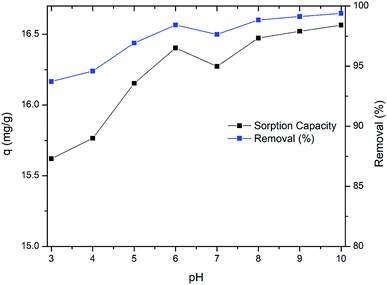 |
| Fig. 8 Effect of initial solution pH on removal of Cr(VI) by B–Al nanocomposite. | |
Generally, clay particles have negative surface over a wide range of pH54 as shown for used bentonite clay in Fig. 9b, a drastic shift was observed in zeta potential (from highly negative to highly positive) after supporting amorphous aluminum on clay surface (Fig. 9a). Point of zero charge (pHPZC) was nearly at pH 8.68. Which shows that, in environmental conditions (pH 4 to 8) the composite will have +ve charge which will strongly attract the dominant anionic Cr(VI) species like HCrO4−. Slightly higher sorption capacity at pH = 6 can be related to the highest positive zeta potential at this point.55
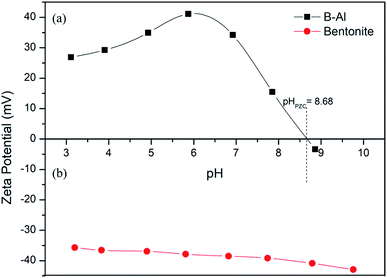 |
| Fig. 9 Zeta potential as a function of pH and point of zero charge (pHPZC) for (a) B–Al nanocomposite and (b) bentonite. | |
3.2.5 Effect of humic acid. Humic substances are complex organic compounds that are omnipresent in water, soils and sediments as well.56 In groundwater and surface water bodies their concentration can range up to 100 mg L−1 with an average of 5 mg L−1.57 To see the effect of humic substances, different concentrations of humic acid (HA) were spiked in the Cr(VI) solution and it was noted (Fig. 10) that increased concentration of HA resulted in drastic decrease in the Cr(VI) removal which is due to rapid oxidation of amorphous aluminum by HA and coprecipitation of organic matter with aluminum hydroxide.58,59 The removal was about 46% in presence of 5 ppm HA which was decreased to 34% when HA concentration was 20 ppm.
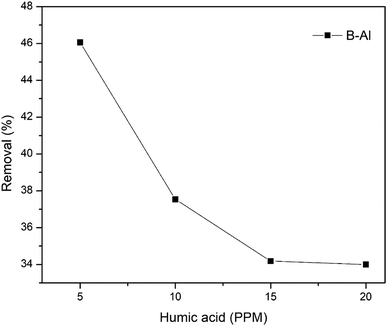 |
| Fig. 10 Effect of humic acid on sorption of Cr(VI) on B–Al nanocomposite. | |
3.2.6 Effect of bicarbonate ions. In the environmentally relevant conditions, carbonate speciation suggests bicarbonate ions as the dominant specie and these ions are very commonly present in natural environment with the concentrations ranging from 0.5 to 8 mM in aqueous environment. Hence, it is essential to study the change in removal efficiency of adsorbent in the presence of varying bicarbonate concentrations. These bicarbonate ions can be adsorbed on mineral oxide surfaces strongly over a wide pH range. HCO3− can reduce the sorption of anionic species due to direct competition for binding sites on sorbent.60,61 Little complex mechanism can occur in case of positively charged species (i.e. heavy metal ions) which involves soluble carbonate complexes and formation of precipitates as well. Varying concentrations of HCO3− (0.5 mM to 5 mM) were spiked and change in removal% was observed (Fig. 11). Data shows a drastic decrease in Cr(VI) sorption capacity of adsorbent. This may be due to rapid adsorption of HCO3− ions on surface of bentonite–amorphous aluminum nanocomposite which inhibits the sorption of present anionic Cr(VI) species.62
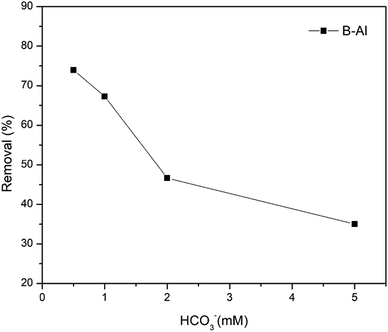 |
| Fig. 11 Effect of bicarbonate ion (HCO3−) concentration on removal of Cr(VI). | |
4. Conclusions
A novel nanocomposite i.e. amorphous aluminum modified bentonite (B–Al nanocomposite) was successfully synthesized using NaBH4 reduction method and was utilized for the removal of Cr(VI) from aqueous solutions. Synthesized B–Al nanocomposite shows excellent sorption capacity for Cr(VI) with a maximum of 49.5 mg g−1. Simultaneous adsorption, rapid reduction, alum formation and precipitation resulted in very rapid removal of Cr(VI) as >90% of chromium was removed from the solution within 5 minutes of interaction. Chemisorption is the major removal mechanism as revealed by well fit of pseudo 2nd order kinetic model and Langmuir, Freundlich, Temkin isotherm models. No effect of increased ionic strength was due to formation of strong inner sphere complexes. Presence of higher concentrations of humic acid and bicarbonate ions reduced the sorption capacity significantly. There was no such effect of solution pH and temperature on sorption which suggests its suitability in various environmental conditions. Alum precipitation and sorption of secondary contaminants (if any) by bentonite clay make it an ecofriendly nanosorbent for toxic metal ions.
Conflicts of interest
There are no conflicts to declare.
Acknowledgements
We gratefully acknowledge the financial support from Ramanujan Fellowship grant from (SB/S2/RJN-006/2016) and ECR project (ECR/2017/000707) from DST, India. We are thankful to Indian Institute of Science Education and Research Kolkata's central instrumentation facility for sample analysis (SEM, TEM, XRD, FTIR and UV-Vis).
References
- X. Y. Wu, et al., A review of toxicity and mechanisms of individual and mixtures of heavy metals in the environment, Environ. Sci. Pollut. Res., 2016, 23(9), 8244–8259 CrossRef CAS PubMed.
- L. Jarup, Hazards of heavy metal contamination, Br. Med. Bull., 2003, 68, 167–182 CrossRef PubMed.
- G. K. Darbha, A. Ray and P. C. Ray, Gold nanoparticle-based miniaturized nanomaterial surface energy transfer probe for rapid and ultrasensitive detection of mercury in soil, water, and fish, ACS Nano, 2007, 1(3), 208–214 CrossRef CAS PubMed.
- G. K. Darbha, et al., Selective detection of mercury (II) ion using nonlinear optical properties of gold nanoparticles, J. Am. Chem. Soc., 2008, 130(25), 8038–8043 CrossRef CAS PubMed.
- R. Saha, R. Nandi and B. Saha, Sources and toxicity of hexavalent chromium, J. Coord. Chem., 2011, 64(10), 1782–1806 CrossRef CAS.
- J. Guertin, et al., Chromium (VI) handbook, Boca Raton, Fla, CRC Press, 2005, p. 784 Search PubMed.
- World Health Organization, Guidelines for drinking-water quality, World Health Organization, Geneva, 4th edn, 2011, xxiii, p. 541 Search PubMed.
- Agency, U.S.E.P., National Primary Drinking Water Regulations, 2012 Search PubMed.
- Standards, B.o.I., Indian Standard drinking water specification, 2012, New Delhi, India Search PubMed.
- R. J. Reeder, M. A. A. Schoonen and A. Lanzirotti, Metal Speciation and Its Role in Bioaccessibility and Bioavailability, Rev. Mineral. Geochem., 2006, 64(1), 59–113 CrossRef CAS.
- A. S. Dharnaik and P. K. Ghosh, Hexavalent chromium [Cr(VI)] removal by the electrochemical ion-exchange process, Environ. Technol., 2014, 35(17–20), 2272–2279 CrossRef CAS PubMed.
- J. A. Korak, R. Huggins and M. Arias-Paic, Regeneration of pilot-scale ion exchange columns for hexavalent chromium removal, Water Res., 2017, 118, 141–151 CrossRef CAS PubMed.
- S. K. Sharma, B. Petrusevski and G. Amy, Chromium removal from water: a review, J. Water Supply: Res. Technol.–AQUA, 2008, 57(8), 541–553 CrossRef CAS.
- K. Bhowmik, et al., Stable Ni Nanoparticle-Reduced Graphene Oxide Composites for the Reduction of Highly Toxic Aqueous Cr(VI) at Room Temperature, Langmuir, 2014, 30(11), 3209–3216 CrossRef CAS PubMed.
- H. Jabeen, et al., Enhanced Cr(VI) removal using iron nanoparticle decorated graphene, Nanoscale, 2011, 3(9), 3583–3585 RSC.
- T. Wang, et al., Synthesis of Core-Shell Magnetic Fe3O4@poly(m-Phenylenediamine) Particles for Chromium Reduction and Adsorption, Environ. Sci. Technol., 2015, 49(9), 5654–5662 CrossRef CAS PubMed.
- R. M. Schneider, et al., Adsorption of chromium ions in activated carbon, Chem. Eng. J., 2007, 132(1–3), 355–362 CrossRef CAS.
- N. S. Rajurkar, A. N. Gokarn and K. Dimya, Adsorption of Chromium(III), Nickel(II), and Copper(II) from Aqueous Solution by Activated Alumina, Clean: Soil, Air, Water, 2011, 39(8), 767–773 CAS.
- S. Mortazavian, et al., Activated carbon impregnated by zero-valent iron nanoparticles (AC/nZVI) optimized for simultaneous adsorption and reduction of aqueous hexavalent chromium: material characterizations and kinetic studies, Chem. Eng. J., 2018, 353, 781–795 CrossRef CAS.
- N. N. Fathima, et al., Solid waste removes toxic liquid waste: adsorption of chromium(VI) by iron complexed protein waste, Environ. Sci. Technol., 2005, 39(8), 2804–2810 CrossRef CAS PubMed.
- L. N. Shi, X. Zhang and Z. L. Chen, Removal of chromium (VI) from wastewater using bentonite-supported nanoscale zero-valent iron, Water Res., 2011, 45(2), 886–892 CrossRef CAS PubMed.
- C.-Y. Hu, et al., Hexavalent chromium removal from near natural water by copper–iron bimetallic particles, Water Res., 2010, 44(10), 3101–3108 CrossRef CAS PubMed.
- M. Rivero-Huguet and W. D. Marshall, Influence of various organic molecules on the reduction of hexavalent chromium mediated by zero-valent iron, Chemosphere, 2009, 76(9), 1240–1248 CrossRef CAS PubMed.
- H. Zhou, et al., Influence of complex reagents on removal of chromium(VI) by zero-valent iron, Chemosphere, 2008, 72(6), 870–874 CrossRef CAS PubMed.
- R. Singh, V. Misra and R. P. Singh, Removal of hexavalent chromium
from contaminated ground water using zero-valent iron nanoparticles, Environ. Monit. Assess., 2012, 184(6), 3643–3651 CrossRef CAS PubMed.
- T. Liu, D. C. Tsang and I. M. Lo, Chromium(VI) reduction kinetics by zero-valent iron in moderately hard water with humic acid: iron dissolution and humic acid adsorption, Environ. Sci. Technol., 2008, 42(6), 2092–2098 CrossRef CAS PubMed.
- X. Guan, et al., The limitations of applying zero-valent iron technology in contaminants sequestration and the corresponding countermeasures: the development in zero-valent iron technology in the last two decades (1994-2014), Water Res., 2015, 75, 224–248 CrossRef CAS PubMed.
- Y. Li, et al., Hexavalent chromium removal from aqueous solution by adsorption on aluminum magnesium mixed hydroxide, Water Res., 2009, 43(12), 3067–3075 CrossRef CAS PubMed.
- S. Li, et al., In situ synthesis of layered double hydroxides on gamma-Al2O3 and its application in chromium(VI) removal, Water Sci. Technol., 2017, 75(5–6), 1466–1473 CrossRef CAS PubMed.
- J. Adusei-Gyamfi and V. Acha, Carriers for nano zerovalent iron (nZVI): synthesis, application and efficiency, RSC Adv., 2016, 6(93), 91025–91044 RSC.
- L. N. Shi, et al., Synthesis, characterization and kinetics of bentonite supported nZVI for the removal of Cr(VI) from aqueous solution, Chem. Eng. J., 2011, 171(2), 612–617 CrossRef CAS.
- C. C. Ding, et al., Plasma synthesis of beta-cyclodextrin/Al(OH)3 composites as adsorbents for removal of UO22+ from aqueous solutions, J. Mol. Liq., 2015, 207, 224–230 CrossRef CAS.
- Z. Zhang, et al., Effect of Staphylococcus epidermidis on U(VI) sequestration by Al-goethite, J. Hazard. Mater., 2019, 368, 52–62 CrossRef CAS PubMed.
- J. Shao, et al., Nanoscale Zero-Valent Iron Decorated on Bentonite/Graphene Oxide for Removal of Copper Ions from Aqueous Solution, Materials, 2018, 11(6), 945 CrossRef PubMed.
- Y. B. Sun, et al., Sequestration of uranium on fabricated aluminum co-precipitated with goethite (Al-FeOOH), Radiochim. Acta, 2014, 102(9), 797–804 CAS.
- B. W. Hu, et al., New insights into Th(IV) speciation on sepiolite: Evidence for EXAFS and modeling investigation, Chem. Eng. J., 2017, 322, 66–72 CrossRef CAS.
- W. C. Cheng, et al., Fabrication of fungus/attapulgite composites and their removal of U(VI) from aqueous solution, Chem. Eng. J., 2015, 269, 1–8 CrossRef CAS.
- Y. B. Sun, J. X. Li and X. K. Wang, The retention of uranium and europium onto sepiolite investigated by macroscopic, spectroscopic and modeling techniques, Geochim. Cosmochim. Acta, 2014, 140, 621–643 CrossRef CAS.
- R. A. Nyquist, et al., The handbook of infrared and Raman spectra of inorganic compounds and organic salts, Academic Press, San Diego, 1997 Search PubMed.
- S. Music, et al., Formation of chromia from amorphous chromium hydroxide, Croat. Chem. Acta, 1999, 72(4), 789–802 CAS.
- G. A. Ikhtiyarova, et al., Characterization of natural- and organo-bentonite by XRD, SEM, FT-IR and thermal analysis techniques and its adsorption behaviour in aqueous solutions, Clay Miner., 2012, 47(1), 31–44 CrossRef CAS.
- Z. X. Chi, et al., Bentonite-supported nanoscale zero-valent iron granulated electrodes for industrial wastewater remediation, RSC Adv., 2017, 7(70), 44605–44613 RSC.
- H. H. Teng, et al., Removal of Hexavalent Chromium from Aqueous Solutions by Sodium Dodecyl Sulfate Stabilized Nano Zero-Valent Iron: A Kinetics, Equilibrium, Thermodynamics Study, Sep. Sci. Technol., 2013, 48(11), 1729–1737 CrossRef CAS.
- Y. S. Ho and G. McKay, Pseudo-second order model for sorption processes, Process Biochem., 1999, 34(5), 451–465 CrossRef CAS.
- Y. S. Ho, Review of second-order models for adsorption systems, J. Hazard. Mater., 2006, 136(3), 681–689 CrossRef CAS PubMed.
- J.-P. Simonin and J. Bouté, Intraparticle diffusion-adsorption model to describe liquid/solid adsorption kinetics, Rev. Mex. Ing. Quim., 2016, 15(1), 161–173 CAS.
- H. K. Boparai, M. Joseph and D. M. O'Carroll, Kinetics and thermodynamics of cadmium ion removal by adsorption onto nano zerovalent iron particles, J. Hazard. Mater., 2011, 186(1), 458–465 CrossRef CAS PubMed.
- B. Choudhary and D. Paul, Isotherms, kinetics and thermodynamics of hexavalent chromium removal using biochar, J. Environ. Chem. Eng., 2018, 6(2), 2335–2343 CrossRef CAS.
- S. Goldberg, Inconsistency in the triple
layer model description of ionic strength dependent boron adsorption, J. Colloid Interface Sci., 2005, 285(2), 509–517 CrossRef CAS PubMed.
- Y. Zhang, et al., Effects of ionic strength on removal of toxic pollutants from aqueous media with multifarious adsorbents: A review, Sci. Total Environ., 2019, 646, 265–279 CrossRef CAS PubMed.
- M. C. S. Faria, et al., Arsenic removal from contaminated water by ultrafine delta-FeOOH adsorbents, Chem. Eng. J., 2014, 237, 47–54 CrossRef CAS.
- W. Stumm, The Inner-Sphere Surface Complex, in Aquatic Chemistry, American Chemical Society, 1995. pp. 1–32 Search PubMed.
- H. T. Ren, et al., Effects of pH and Anion Species on Cr(VI) Removal by Magnetite, Fundamental of Chemical Engineering, 2011, 233–235, 1055–1058 CAS.
- P.-I. Au and Y.-K. Leong, Rheological and zeta potential behaviour of kaolin and bentonite composite slurries, Colloids Surf., A, 2013, 436, 530–541 CrossRef CAS.
- P. Wang, et al., Halloysite nanotube@carbon with rich carboxyl groups as a multifunctional adsorbent for the efficient removal of cationic Pb(ii), anionic Cr(vi) and methylene blue (MB), Environ. Sci.: Nano, 2018, 5(10), 2257–2268 RSC.
- G. R. Aiken, Humic substances in soil, sediment, and water: geochemistry, isolation, and characterization, Wiley, New York, 1985, xiii, p.692 Search PubMed.
- S. J. Boggs, D. Livermore, and M. G. Seitz, Humic substances in natural waters and their complexation with trace metals and radionuclides: a review, [129 references], 1985, Argonne National Lab, IL (USA), p. medium: ED; size: p. 118 Search PubMed.
- M. R. Jekel, Interactions of humic acids and aluminum salts in the flocculation process, Water Res., 1986, 20(12), 1535–1542 CrossRef CAS.
- E. Tipping, et al., Al(III) and Fe(III) binding by humic substances in freshwaters, and implications for trace metal speciation, Geochim. Cosmochim. Acta, 2002, 66(18), 3211–3224 CrossRef CAS.
- I. M. C. Lo, C. S. C. Lam and K. C. K. Lai, Hardness and carbonate effects on the reactivity of zero-valent iron for Cr(VI) removal, Water Res., 2006, 40(3), 595–605 CrossRef CAS PubMed.
- J. M. Zachara, et al., Chromate Adsorption on Amorphous Iron Oxyhydroxide in the Presence of Major Groundwater Ions, Environ. Sci. Technol., 1987, 21(6), 589–594 CrossRef CAS PubMed.
- M. Villalobos, M. A. Trotz and J. O. Leckie, Surface complexation modeling of carbonate effects on the adsorption of Cr(VI), Pb(II), and U(VI) on goethite, Environ. Sci. Technol., 2001, 35(19), 3849–3856 CrossRef CAS PubMed.
Footnote |
† Electronic supplementary information (ESI) available. See DOI: 10.1039/c9ra00742c |
|
This journal is © The Royal Society of Chemistry 2019 |