DOI:
10.1039/C9RA00551J
(Review Article)
RSC Adv., 2019,
9, 19465-19482
Solvent-free incorporation of CO2 into 2-oxazolidinones: a review
Received
22nd January 2019
, Accepted 4th May 2019
First published on 21st June 2019
Abstract
This review is an attempt to give an overview on the recent advances and developments in the synthesis of 2-oxazolidinone frameworks through carbon dioxide (CO2) fixation reactions under solvent-free conditions. The cycloaddition of CO2 to aziridine derivatives is discussed first. This is followed by carboxylative cyclization of N-propargylamines with CO2 and three-component coupling of epoxides, amines, and CO2. Finally, cycloaddition of CO2 to propargylic alcohols and amines will be covered at the end of the review. The literature has been surveyed up until the end of 2018.
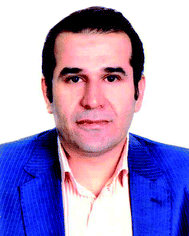 Sattar Arshadi | Sattar Arshadi was born in Miandoab, west Azarbayjan province, Iran, in 1973. He received his BSc degree in chemistry from the University of Kermanshah (1997) and his MSc (2000) under the supervision of Professor Issa Yavari and PhD (2004) in organic chemistry under the supervision of Professor M. Z. Kassaee both at Tarbiat Modares University, Tehran, Iran. Then, he went to the University of Payame Noor as an assistant professor (2005). His main research interests are computational chemistry (especially on rearrangements and interactions in nano systems), organic synthesis and spectral studies of organic compounds. |
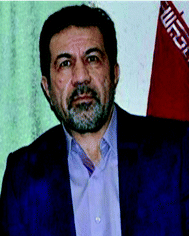 Alireza Banaei | Alireza Banaei was born in Ardabil, Iran, in 1966. He received his BS degree in Pure Chemistry from the University of Shahid Beheshti Tehran, Iran, and his MS degree in organic chemistry from Tabriz University, Tabriz, Iran, in 1994 under the supervision of Prof. A. Shahrisa. He completed his PhD degree in 2000 under the supervision of Prof. A. Shahrisa. Now he is working at the University of Payamenoor as an Associate Professor. His research interests include inorganic and organic synthesis, new methodologies in nano material synthesis, carbon dots. |
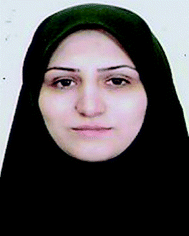 Saeideh Ebrahimiasl | Saeideh Ebrahimiasl was born in Tabriz, Iran, in 1973. She received her PhD degree in nanomaterials and nanotechnology from Institute of Advanced Materials, University Putra Malaysia in 2010. Professor Majid Monajjemi, Professor Wan Zin Wan Yunus, Professor Zulkarnain Zainal, Professor Mohd Zobir in physical chemistry, nanotechnology and electrochemistry were her most famous supervisors and examiners. Now she is head of Industrial nanotechnology Research Center in Tabriz and academic member of Islamic Azad University of Ahar. Her research interests include synthesis and application of nanomaterials in different sciences. |
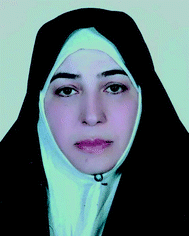 Aazam Monfared | Aazam Monfared was born in Tehran, Iran, in 1965. She received her BS degree in Pure Chemistry from University of Shahid Beheshti, Tehran, Iran, and her MS degree in Organic chemistry from Shahid Beheshti University, Tehran, Iran, in 1991 under the supervision of Prof. A. Rustaiyan. She received her PhD degree in 1999 under the supervision of Prof. A. Rustaiyan in Shahid Beheshti University, Tehran, Iran. Now, she is working at Payame Noor University of Tehran as Associate Professor. Her research interests include organic synthesis, phytochemistry, drug synthesis, nano chemistry, methodologies and theoretical chemistry. |
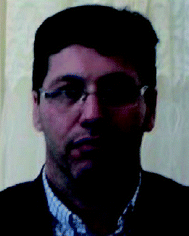 Esmail Vessally | Esmail Vessally was born in Sharabiyan, Sarab, Iran, in 1973. He received his BS degree in Pure Chemistry from University of Tabriz, Tabriz, Iran, and his MS degree in organic chemistry from Tehran University, Tehran, Iran, in 1999 under the supervision of Prof. H. Pirelahi. He completed his PhD degree in 2005 under the supervision of Prof. M. Z. Kassaee. Now he is working at Payame Noor University as full Professor of Organic Chemistry. His research interests include Theoretical Organic Chemistry, new methodologies in organic synthesis and spectral studies of organic compounds. |
1. Introduction
Needless to say that heterocycles are the most common structural classes of natural products1 and marketed drugs.2 It is estimated that over 70% of all pharmaceutical products contain at least one heterocyclic fragment in their structures.3 Interestingly, heterocyclic moieties were present in the structures of all top 10 brand name small molecule drugs (according to retail sales) in 2010.4 2-Oxazolidiones (five-membered cyclic carbamates) are one of the important five-membered heterocyclic compounds which exist in many pharmaceutically active compounds (e.g., zolmitriptan, toloxatone, cimoxatone, befloxatone),5 and agrochemicals (e.g., phosalone).6 In particular, they represent a new class of synthetic antibacterial agents (Fig. 1), active against multiply-resistant Gram-positive pathogens.7 The title compounds are also versatile intermediates in organic synthesis8 and have been widely applied as chiral auxiliaries in asymmetric syntheses.9 General synthetic methods toward the 2-oxazolidione framework involve the oxidative carbonylation of β-amino alcohols using phosgene and its derivatives,10 the oxidative carbonylation of β-amino alcohols using carbon monoxide11 and the intramolecular cyclization of N-Boc-protected propargyl amines.12 However, these methods, suffer from limited substrate scope, prolonged reaction times and require toxic reagents and harsh conditions. Therefore, the development of convenient and truly efficient protocols for the synthesis of 2-oxazolidione compounds that benefit from nontoxic, inexpensive, and easily available substrates is highly desirable.
 |
| Fig. 1 Selected examples of oxazolidinone antibacterial agents. | |
The conversion of carbon dioxide into profitable chemicals such as esters, aldehydes, carboxylic acids, alcohols, and amides has received ever-increasing attention in recent years, not only because it is the chief anthropogenic greenhouse gas, but also because it has been regarded as an abundant, inexpensive, nontoxic, nonflammable, and renewable C1-building block.13,14 In this regard, the synthesis of 2-oxazolidiones employing CO2 as an environmentally friendly alternative to the phosgene is of great interest.15–17 Despite the variety of methodologies for the incorporation of CO2 into 2-oxazolidinones, such reactions usually require expensive and toxic organic solvents which are undesirable effects for health and environmental.18 With increasing awareness of the concept of green chemistry,19–21 researchers have focused their attention on the development of solvent-free processes. In this context, numerous solvent-free procedures for the CO2-based synthesis of 2-oxazolidinones are reported in the literature. Since a number of advances and developments in this interesting research arena have occurred in recent years, a comprehensive review on this hot research topic seems to be timely. As a part of our continual review articles on utilization of CO2 for the production of valuable products,14,17 herein we will highlight the most important advances and discoveries in the synthesis of 2-oxazolidinone derivatives from CO2 under solvent-free conditions (Fig. 2), by hoping that it will stimulate researchers to further thinking and research in the topic.
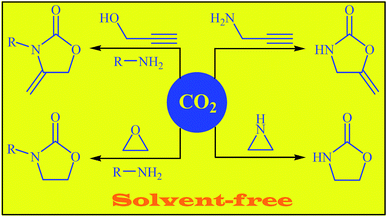 |
| Fig. 2 CO2-based synthesis of 2-oxazolidione framework under solvent-free conditions. | |
2. Cycloaddition of aziridines with CO2
Aziridine, the nitrogenous analogue of epoxide, is one of the most important simple heterocycles, which is found in a wide variety of natural products and biologically active drugs.22 This heterocycle is also one of the well-known and versatile intermediates in the synthesis of various types of nitrogen-containing compounds.23 One very attractive and promising synthetic application of aziridines involves the preparation of 2-oxazolidinone derivatives through the coupling with CO2.16 In 2013, Ghosh and co-workers published an interesting review article that covers most of the advances in this chemistry.15 However, solvent-free reactions were almost omitted. In this section, we present the current literature on cycloaddition of CO2 to aziridines under neat conditions. These lead to the formation of substituted 2-oxazolidinones in a green and ideal atom economic procedure (all the atoms present in the starting materials are present in the product). The ionic liquid-catalyzed reactions are discussed first. This is followed by organocatalyzed and metal-catalyzed reactions. Finally, metal–organic frameworks (MOFs)-catalyzed reactions will be covered at the end of the section.
2.1. Ionic liquid catalyzed reactions
Ionic liquid catalyzed synthesis of oxazolidin-2-one derivatives by cycloaddition of CO2 to aziridines under solvent-free conditions was developed for the first time by L.-N. He and co-workers in 2008.24 They showed that treatment of 1-alkyl-2-arylaziridines 1 with CO2 (8 MPa) in the presence of 0.25 mol% of PEG6000(NBu3Br)2 at 100 °C under solvent-free condition, resulted in 5-aryl-2-oxazolidinones 2 in moderate to excellent yields, along with small amounts of the 4-aryl-oxazolidinone 3 side products (Scheme 1). The results demonstrated that the regiochemical effect of the reaction was strongly dependent on the nature of the R2 group on the starting material. If R2 is an aryl group, product 2 is favored, whereas if R2 is an alkyl group, product 3 is favored. It is noted that 1,2-diphenylaziridine did not take part in this cycloaddition reaction and therefore no other N-aryl aziridines were examined in the protocol. The comparison of the catalytic activity of PEG6000(NBu3Br)2 with unsupported quaternary ammonium (Bu4NBr) and the support (PEG6000) established its superior comparability with them in terms of product yield. In addition, this catalyst displayed higher activity than the simple physical mixture of Bu4NBr and PEG6000 under the same conditions. Interestingly, the recycling test established that the catalyst could be recovered via centrifugation and reused for several times without any significant loss in the catalytic activity. Mechanistically, this PEG6000(NBu3Br)2-catalyzed reaction is believed to proceed through a coordination-ring opening-cyclization sequential process (Scheme 2). Shortly afterwards, the same research team found that 1-butyl-4-aza-1-azoniabicyclo[2.2.2]octane bromide ([C4DABCO]Br) could also effectively catalyze the cycloaddition of CO2 to aziridines under solvent-free conditions.25 Thus, in the presence of 1 mol% of [C4DABCO]Br at 90 °C, the reaction of the same set of 1-alkyl-2-arylaziridines 1 with CO2 (6 MPa) furnished the expected 5-aryl-2-oxazolidinones 2 with yield range from 6% to 92%. The results demonstrated that the efficiency of this reaction was dependent on the steric effects of the alkyl substituent at the nitrogen atom. While substrates bearing a less sterically hindered R1 (e.g., Me, Et, nPr) gave the desired products in good yields, the bulky alkyl group (e.g., iPr, tBu) substituted aziridines afforded unsatisfactory yields. In another study, the same authors reported the synthesis of a library of 5-aryl-2-oxazolidinones in high yields (up to 93%) via fixation of CO2 (3 MPa) onto the corresponding aziridines, catalyzed by BrDBNPEG150DBNBr (DBN: 1,5-diazabicyclo[4.3.0]non-5-ene) and in the absence of solvent or additive.26 The catalyst showed excellent reusability in this system without appreciable decrease in performance after four consecutive runs.
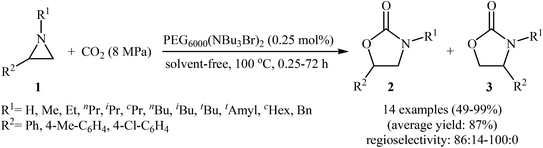 |
| Scheme 1 PEG6000(NBu3Br)2-catalyzed fixation of CO2 with 2-arylaziridines 1 developed by He. | |
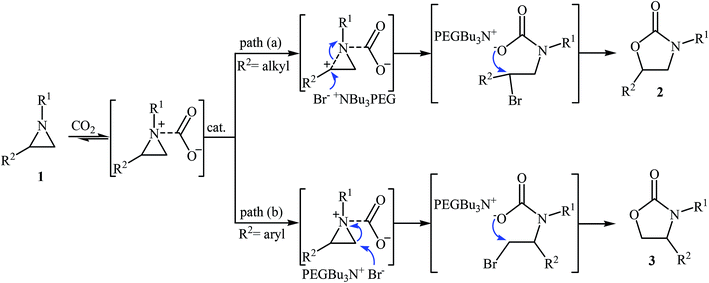 |
| Scheme 2 Mechanistic proposal for the reaction in Scheme 1. | |
In 2011, Bhanage and colleagues introduced a novel polymer supported 1-(2,3-dihydroxylpropyl)-imidazolium bromide (PS-DHPIMBr) catalyst for 2-oxazolidinones synthesis from CO2 and aziridines under solvent-free condition (Scheme 3).27 The hybrid system exhibited a high catalytic activity and reusability for cycloaddition of 1-alkyl-2-arylaziridines 4 with CO2 (5 MPa) under solvent-free conditions (Scheme 4). The cycloaddition was carried out at room temperature for 3–12 h and afforded the desired 5-aryl-2-oxazolidinones 5 in moderate to almost quantitative yields.
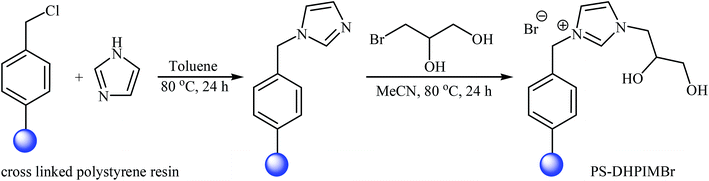 |
| Scheme 3 Synthetic route to PS-DHPIMBr. | |
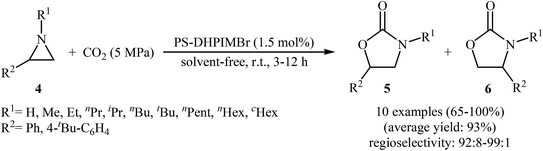 |
| Scheme 4 PS-DHPIMBr-catalyzed fixation of CO2 with 1-alkyl-2-arylaziridines 4 under neat conditions. | |
Very recently, Luo and Ji along with their co-workers designed and synthesized a novel imidazolium-based ionic liquid functionalized zinc porphyrin (IL-ZnTPP) as one of the most versatile catalysts known for this reaction (Fig. 3).28 The prepared IL-functionalized metalloporphyrin was employed as an efficient catalyst for cycloaddition of CO2 to N-substituted-2-arylaziridines under solvent-free conditions. Several 5-aryl and 4-aryl substituted 2-oxazolidinones with a regioisomeric ratio up to 98
:
2 were synthesized at 90 °C and 2 MPa of CO2 by using very low catalytic loading of 0.1 mol%. The IL-ZnTPP was also successfully utilized as a catalyst for the cycloaddition reaction of various epoxides with CO2. Moreover, this catalyst could be reused up to ten times without any change in its catalytic activity and selectivity.
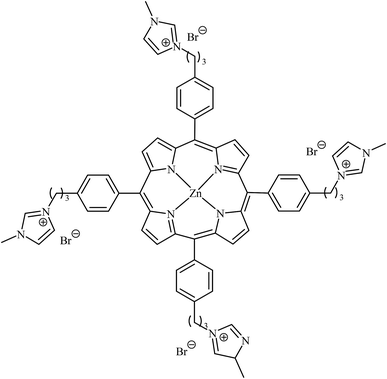 |
| Fig. 3 Chemical structure of IL-ZnTPP. | |
2.2. Organocatalyzed reactions
In 2010, Jiang and co-workers investigated the application of α-amino acids as hydrogen bond donor catalysts for the fixation of CO2 onto aziridines.29 They tested several naturally occurring α-amino acids for the benchmark cycloaddition of CO2 (8 MPa) to N-propyl-2-phenylaziridine under solvent-free conditions. After optimization, it was found that the use of 0.6 mol% of L-histidine as the catalyst gave the best results. Examination of the scope of the reaction revealed that a variety of 1-alkyl-2-arylaziridines 7 bearing both electron-donating and -withdrawing groups afforded the 5-aryl-2-oxazolidinone derivatives 8 in fair to excellent yields along with trace amounts of 4-aryl-2-oxazolidinone 9 side products (Scheme 5). According to the author proposed mechanism (Scheme 6, path a), this cycloaddition reaction proceeds through the formation of intermediate A via the activation of the aziridine ring by hydrogen bonding of primary amine group of amino acid with nitrogen atom of aziridine 7. Next, a nucleophilic attack of the carboxylate ion of another amino acid on the more sterically hindered side of the activated aziridine ring affords the zwitterion B, which after interaction with CO2 affords intermediate C. Finally, the intramolecular cyclization of intermediate C leads to the expected 5-aryl-2-oxazolidinone 8. In another possibility (Scheme 6, path b), ring-opening reaction of intermediate A occurs at the less sterically hindered side of the aziridine ring to form intermediate D, which undergoes interaction with CO2 to afford the intermediate E. The last step of the transformation involves the intramolecular cyclization via nucleophilic attack furnishing the 4-aryl-2-oxazolidinone 9. In a closely related investigation, Dou, He, and Yang also described that the reaction of CO2 (6 MPa) with aziridines in the presence of a catalytic amount of proline under neat conditions produced corresponding 2-oxazolidinones good yields.30
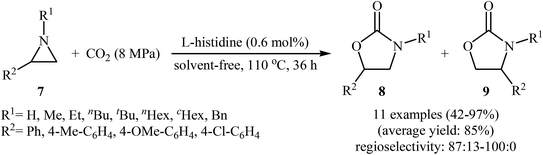 |
| Scheme 5 L-Histidine-catalyzed cycloaddition of CO2 with 1-alkyl-2-arylaziridines 7. | |
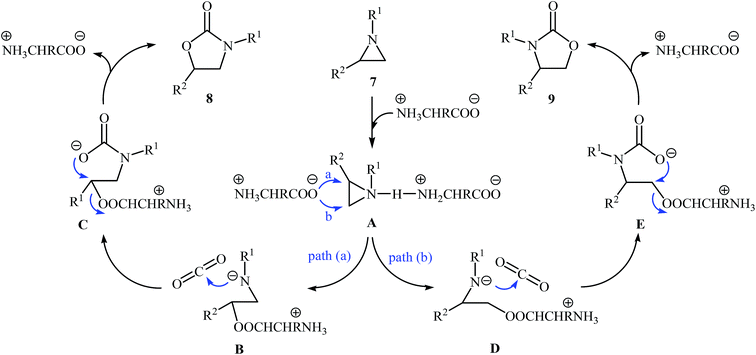 |
| Scheme 6 Mechanism that accounts for the formation of 2-oxazolidinones 8 and 9. | |
In 2014, Bhanage's research team developed a novel amine functionalized MCM-41, through one-pot reaction of cetyltrimethyl ammonium bromide (CTAB), tetraethyl ortho-silicate (TEOS), and 3-[2-(2-aminoethylamino)ethylamino]propyltrimethoxysilane (AEPTMS) in an aqueous solution of NaOH followed by treatment of the obtained solid product with a mixture of EtOH/HCl with ratio 100
:
1 at 80 °C (Fig. 4).31 The amine functionalized MCM-41 was found to be an efficient catalyst in the synthesis of 2-oxazolidinones through the coupling CO2 (5 MPa) with corresponding aziridines under mild, metal-, additive-, and solvent-free conditions. Interestingly, this heterogeneous catalyst could be successfully recovered from the reaction mixture by a simple filtration, followed by washing with acetone and distilled water and drying. It could be reused for five consecutive runs without any remarkable loss in its catalytic activity. The authors suggested that the amino groups could be the active sites of this catalyst and proposed a mechanistic pathway similar to the one described in Scheme 6.
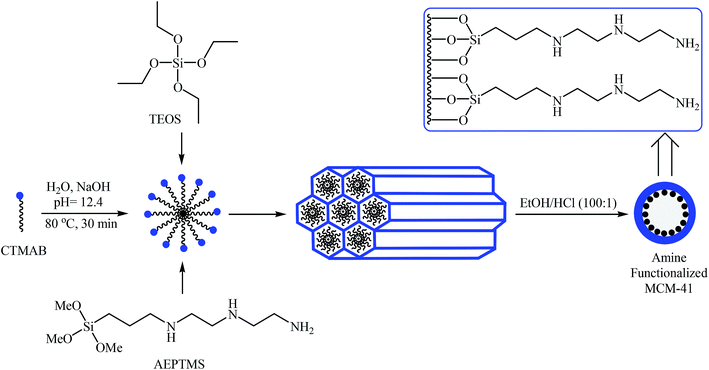 |
| Fig. 4 Synthesis route for the preparation of amine functionalized MCM-41. | |
The same group also reported the use of N-heterocyclic olefins 10 as robust organocatalysts for the cycloaddition of CO2 to aziridines under solvent-free conditions (Scheme 7a).32 The catalytic activity of these various N-heterocyclic olefins was found to be of the order 10d > 10c > 10b > 10a. Therefore, 2,3-dihydro-1,3-diisopropyl-2-methylene-1H-imidazole 10d was chosen for the synthesis of 2-oxazolidinones. Under optimized conditions [10d (0.4 mol%), CO2 (2 MPa), neat, r.t.] a series of 1-alkyl-2-arylaziridines 12 react to give corresponding 5-aryl-2-oxazolidinones 13 in moderate to excellent yields with excellent turnover numbers (TONs) and turnover frequencies (TOFs) (Scheme 7b). Notably, this catalyst has also been successfully utilized for N-formylation of amines with CO2 in the presence of polymethylhydrosiloxane (PMHS) and 9-borabicyclo[3.3.1]nonane (9-BBN) as the reducing agent.
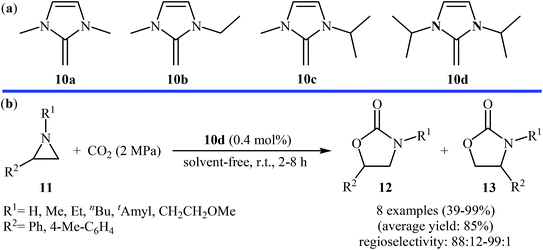 |
| Scheme 7 (a) Chemical structure of N-heterocyclic olefins 10a–d; (b) fixation of CO2 with 1-alkyl-2-arylaziridines 11 by using 2,3-dihydro-1,3-diisopropyl-2-methylene-1H-imidazole as an organocatalyst. | |
The synthesis of a library of 5-aryl-2-oxazolidinones in high yields (up to 98%) and excellent regioselectivity (100%) was also reported by Peng-Sun and co-workers through the coupling of CO2 (2 MPa) with corresponding aziridines using an agricultural and sugar mill waste material, sugarcane bagasse (SCB), as an efficient, reusable, and environmental catalyst and KI as an additive under solvent-free conditions at 120 °C. It should be mentioned that SCB is a complex biopolymer, mainly consisting of cellulose (40–50%), hemicelluloses (25–30%), and lignin (20–25%).33
2.3. Metal-catalyzed reactions
In 2009, one of the earliest metal-catalyzed coupling of aziridines with CO2 under solvent-free conditions was published by L. N. He and co-workers, who showed that the reaction of 1-alkyl-2-arylaziridines 14 with CO2 (6 MPa) in the presence of 5 mol% of ZrOCl2·8H2O as an inexpensive and moisture stable catalyst at 100 °C afforded 5-aryl-2-oxazolidinones 15 in moderate to excellent yields and outstanding regioselectivity (Scheme 8).34 Moreover, the reaction of a chiral aziridine with CO2 under the standard condition gave the desired 2-oxazolidinone with retention of stereochemistry. The authors found that other zirconium catalysts also promoted the reaction (e.g., Zr(SO4)2·4H2O, ZrOSO4·4H2O, ZrO(NO3)2·2H2O); albeit in lower yields. The recycling test established that the catalyst could be freely recycled and reused over five times for the same reaction without significant loss in the catalytic activity. Interestingly, the recovered catalyst showed higher activity in comparison with the fresh catalyst (yield was increased from 80% in the first run to 90% in the fifth run), presumably due to its morphological variation. Five years later, the same authors slightly improved the efficiency of this cycloaddition reaction in the terms of yield and CO2 pressure by performing the process in the presence of 5 mol% of mesoporous zirconium organophosphonate (ZrHEDP, HEDP = 1-hydroxyethylidene-1,1′-diphosphonic acid) as a heterogeneous reusable catalyst at 100 °C.35
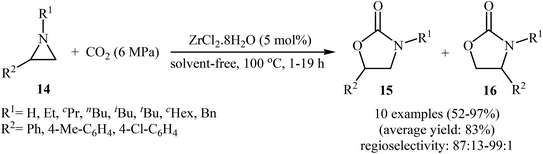 |
| Scheme 8 ZrCl2-catalyzed coupling of aziridines 14 with CO2 under solvent-free conditions. | |
With the aim of designing a milder procedure to 2-oxazolidinone derivatives via metal-catalyzed cycloaddition of CO2 to aziridines under neat conditions, recently, Ji and co-workers were able to demonstrate that a series of 5-aryl-2-oxazolidinones 19 could be successfully obtained via the incorporation of CO2 at 1.0 MPa pressure into corresponding 1-alkyl-2-arylaziridines 18 employing only 1 mol% of bifunctional aluminum salen complex 17 as a novel and recyclable catalyst at 50 °C (Scheme 9).36
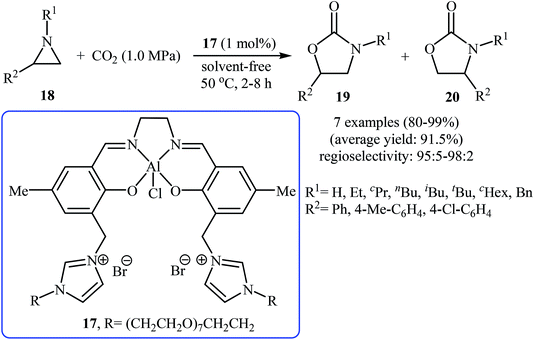 |
| Scheme 9 Cycloaddition of aziridines 18 with CO2 under solvent-free conditions catalyzed by bifunctional aluminum salen complex 17. | |
2.4. Metal–organic framework-catalyzed reactions
In 2014, Ma and Qiao designed a novel titanium–phosphonate hybrid material with mesoscale periodicity by using amino-containing alendronate sodium trihydrate (AST) as a coupling molecule in a facile one-pot hydrothermal method.37 The hybrid material possesses highly periodic mesopores with a large Brunauer–Emmett–Teller (BET) surface area of 540 m2 g−1 and pore volume of 0.43 cm2 g−1. The obtained material was successfully used as a reusable catalyst for the cycloaddition reaction between 1-alkyl-2-arylaziridines 21 and CO2. In their optimization study, the authors found that the use of only 1 mol% of catalyst under solvent-free conditions and 3 MPa pressure of CO2 gave the best results (Scheme 10). This hybrid system (Ti-AST) with homogeneously incorporated phosphonate moieties has bifunctional acidic and basic sites owing to abundant P–OH and –NH2 groups, respectively. The results showed that phosphate and amino groups can respectively activate aziridine and CO2 (Scheme 11). For Ti-MDA (MDA: methylene diphosphonic acid) without –NH2, CO2 molecules physically adsorbed on the pore surface and fail to undergo nucleophilically attack by aziridine; while for Ti-AST′ with insufficient P–OH the majority of aziridine molecules remain inert without fully activation by P–OH, resulting in the low catalytic activity.
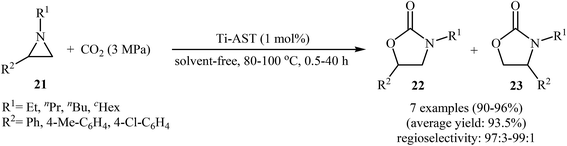 |
| Scheme 10 Cycloaddition reaction between 1-alkyl-2-arylaziridines 21 and CO2 catalyzed by Ti-MDA under solvent-free conditions. | |
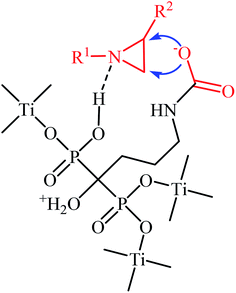 |
| Scheme 11 Activation of aziridines and CO2 with dual active sites of Ti-MDA. | |
In 2016, Zhao and He along with their co-workers prepared a unique porous framework {[Cu2(BCP)(H2O)2]·3DMF}n(H4BCP = 5-(2,6-bis(4-carboxyphenyl)pyridin-4-yl)isophthalic acid) with 30-nuclear copper nanocages by solvothermal methods.38 The metal–organic framework was reported to be an efficient and environmentally friendly catalyst for the coupling of 1-alkyl-2-arylaziridines 24 with CO2 (2 MPa) in the presence of tetrabutylammonium bromide (TBAB) as a co-catalyst under solvent-free conditions (Scheme 12). The MOF could be recovered by centrifugation and filtration, and efficiently reused for 10 catalytic cycles without any obvious loss in catalytic activity. Notably, the ICP analysis of reaction filtrate indicated the leaching of the active catalytic species was negligible.
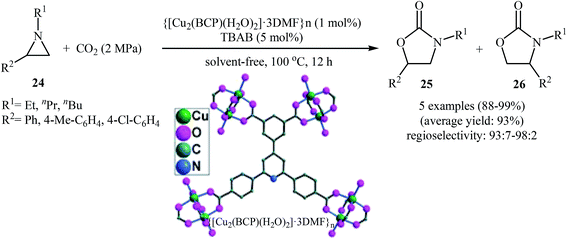 |
| Scheme 12 {[Cu2(BCP)(H2O)2]·3DMF}n-catalyzed coupling of aziridines 24 with CO2. | |
3. Carboxylative cyclization of N-propargylamines with CO2
N-Propargylamines are one of the most versatile and specific class of heteroatom-containing alkynes having diverse reaction patterns.39 They have been widely used as building blocks in the synthesis of various N-heterocycles, including pyrroles, pyridines, pyrazines, quinolines, imidazoles, lactames, etc40. In recent years tremendous effort has been made to develop the carboxylative cyclization of titled compounds with carbon dioxide to provide the 2-oxazolidinone derivatives.17a In this section, we will look at the available literature on the synthesis of 2-oxazolidinones via fixation of CO2 with N-propargylamines under solvent-free conditions. The section is divided into two major subsections. The first focuses exclusively on metal-catalyzed reactions while the second will discuss metal-free procedures.
3.1. Metal-catalyzed reactions
In 2016, the group of L.-N. He developed a novel copper(II) substituted polyoxometalate-based ionic liquid [(nC7H15)4N]6[SiW11O39Cu] as a halogen-free single-component bifunctional catalyst for the carboxylative cyclization of N-propargylamines 27 with CO2 under solvent-free conditions.41 The reaction proceeded smoothly under mild conditions (atmospheric pressure of CO2 at 60 °C) and afforded the expected 5-alkylideneoxazolidin-2-ones 28 in excellent yields (Scheme 13). The protocol is noteworthy in that both internal and external N-propargylamines were well tolerated. However, α,α-disubstituted N-propargylamines failed to participate in this reaction. It was suggested that the catalyst simultaneously activate both CO2 and N-propargylamines. According to the authors proposed mechanism, both the CO2 molecule and N–H bond of propargylamine could be activated by the polyoxometalate anion and the Cu(II) species is able to active the triple bond of propargylamine, thus promoting CO2 conversion under mild conditions (Scheme 14).
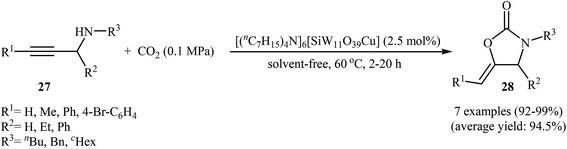 |
| Scheme 13 Carboxylative cyclization of aziridines 27 with atmospheric CO2 developed by He and co-workers. | |
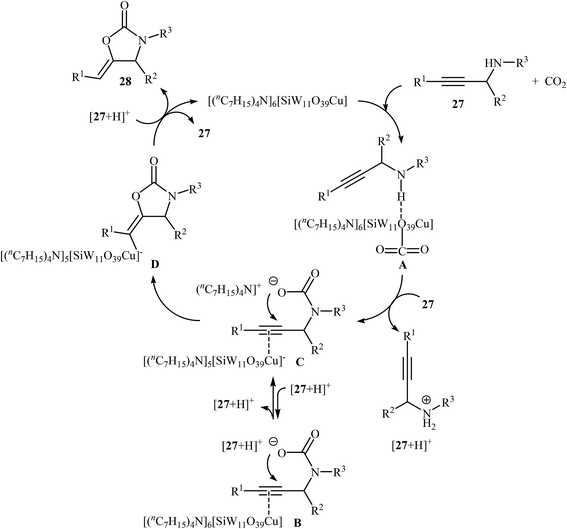 |
| Scheme 14 Proposed mechanism for the formation of 5-methylene-2-oxazolidinone 28. | |
Shortly afterwards, Sadeghzadeh, Zhiani, and Emrani introduced a novel nanosilica-supported nano-Ni@Pd-based ionic liquid (KCC-1/IL/Ni@Pd) catalyst for fixation of CO2 into N-propargylamines.42 The prepared metal–organic framework was employed as an efficient catalyst for carboxylative cyclization of substituted N-propargylamines 29 with CO2 (1 MPa) at room temperature under neat conditions. Various terminal, internal, primary, and secondary N-propargylamines were effectively used to synthesize functionalized 2-oxazolidinones 30 in high to excellent yields (Scheme 15). The attractive features of this protocol are the short reaction time, mild reaction condition, solvent- and additive-free procedure, and very high reusability of the catalyst (up to 10 ten consecutive cycles). These features constitute an economic advantage for organic transformation.
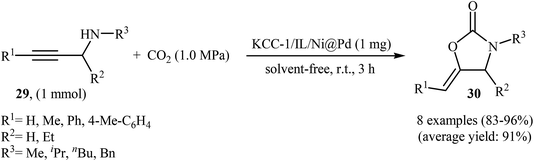 |
| Scheme 15 KCC-1/IL/Ni@Pd-catalyzed carboxylative cyclization of N-propargylamines 29 with CO2 under solvent-free conditions. | |
In a related investigation, the same research team applied KCC-1 nanoparticle-supported Salen/Ru catalyst (KCC-1/Salen/Ru(II) NPs) for the cycloaddition of the same set of N-propargylamines 29 with CO2 (1 MPa) under solvent-free conditions at 100 °C.43 The reaction was completed within 1 h and the target 2-oxazolidinones 30 were obtained with yield range from 92% to 98%.
3.2. Metal-free reactions
The first example of the synthesis of 2-oxazolidinones through carboxylative cyclization of N-propargylamines with CO2 under metal- and solvent-free conditions appeared in 2010, when N-benzylprop-2-yn-1-amine 31 underwent cyclization with CO2 (2 MPa) in the presence of commercially available D301 resin, one kind of polystyryl-supported tertiary amine, as the catalyst at 100 °C. The corresponding 5-methylene-2-oxazolidinone 32 was obtained in approximately 90% yield (Scheme 16).44 D301R was also demonstrated to be highly efficient catalyst for the cycloaddition of CO2/CS2 to aziridines.
 |
| Scheme 16 Synthesis of 5-methylene-2-oxazolidinone 32 via D301R-catalyzed fixation of CO2 with N,N-benzylprop-2-yn-1-amine 31. | |
Very recently, Takata and co-workers presented an elegant solvent-free chemical fixation of atmospheric CO2 into polymers 33 having propargylamine moieties in the main and side chains employing 1,8-diazabicyclo [5.4.0]undec-7-ene (DBU) as a base catalyst.45 The reactions were carried out in the absence of any solvent at 60 °C and provided the corresponding polyoxazolidinones 34 at excellent conversion rates and high (Z)-selectivities (Scheme 17). Interestingly, the cyclization reaction clearly enhanced the thermal stability of the polymers.
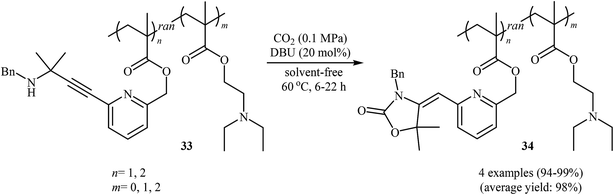 |
| Scheme 17 DBU-catalyzed CO2 fixation in polypropargylamines 33. | |
4. Three-component coupling of CO2, epoxides, and amines
Three-component reactions of CO2, epoxides, and amines are one of the most promising and novel methodologies for the synthesis of 3-substituted-2-oxazolidinones that have been the subject of number of papers in recent years. The present section will concentrate on carboxylative coupling of epoxides, amines and CO2 under solvent-free conditions.
4.1. Metal-free reactions
The first mention of the synthesis of 2-oxazolidinones through the three-component reaction between amines, epoxides and CO2 can be found in a 2014 paper by Gao and co-workers.46 They showed that treatment of ethylene oxide with aromatic amines 35 under the CO2 atmosphere (2.5 MPa) and solvent-free conditions employing 10 + 10 mol% of binary ionic liquids of BmimBr + BmimOAc as a catalytic system furnished the corresponding 3-aryl-2-oxazolidinones 36 in good to quantitative yields (Scheme 18). The results demonstrated that electron-poor anilines afforded better yields compared to the electron-rich ones. Unfortunately, in the cases of substituted epoxides and aliphatic amines the reaction did not give good yields of the desired products.
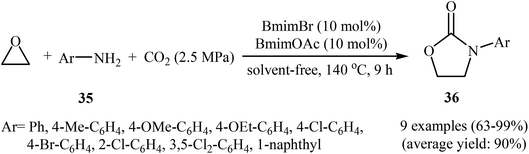 |
| Scheme 18 Gao's synthesis of 3-aryl-2-oxazolidinones 36. | |
Two year later, in a beautiful approach, the same group disclosed a metal- and solvent-free three-component reaction between epoxides 37, aromatic amines 38, and CO2 for the synthesis of 3-substituted-2-oxazolidinones 39 using the combination of 1,8-diazabicyclo[5.4.0]undec-7-ene (DBU) and a DBU-derived bromide ionic liquid (HDBUBr) as a novel synergistic catalytic system.47 The reactions were carried out under a 2.5 MPs pressure of CO2 at 130–160 °C, tolerated a series of important functional groups such as chloro, bromo, and methoxy, and provided the expected 3-aryl-2-oxazolidinones 39 in moderate to excellent yields (Scheme 19). The results showed that the presence of both organic base (DBU) and ionic liquid (HDBUBr) were critical for the success of this cyclization reaction. Unsatisfactory results were obtained in the absence of any of them. The mechanism of this carboxylative coupling was proposed based on nuclear magnetic resonance (NMR) spectroscopy investigations and density functional theory (DFT) calculations determining that the reaction proceeds by activation of epoxide via a hydrogen bond interaction with the proton of HDBUBr and then nucleophilic attack of the bromide anion of this ionic liquid to the activated epoxide to form intermediate A. Next nucleophilic attack of this intermediate to the carbon atom in CO2 gives alkyl carbonate salt intermediate B, which cyclize to the corresponding cyclic carbonate C. In parallel, nucleophile attack of the activated amine (through a hydrogen bond interaction with DBU) to the carbon atom of 2-bromoethanol in intermediate A leads to the 2-(phenylamino)ethanol intermediate D that after activation by DBU attacks to the carbonyl group of cyclic carbonate C to produce a salt intermediate E. Subsequently, an intramolecular proton transfer reaction takes place to give a carbonate intermediate F. Finally, intramolecular nucleophilic attack of a nitrogen atom to a carbonyl group in this intermediate affords the desired 3-substituted-2-oxazolidinone 39 (Scheme 20).
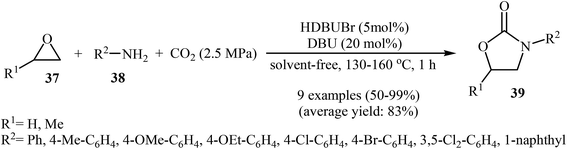 |
| Scheme 19 DBU/HDBUBr-catalyzed three-component synthesis of 3-substituted-2-oxazolidinone 39. | |
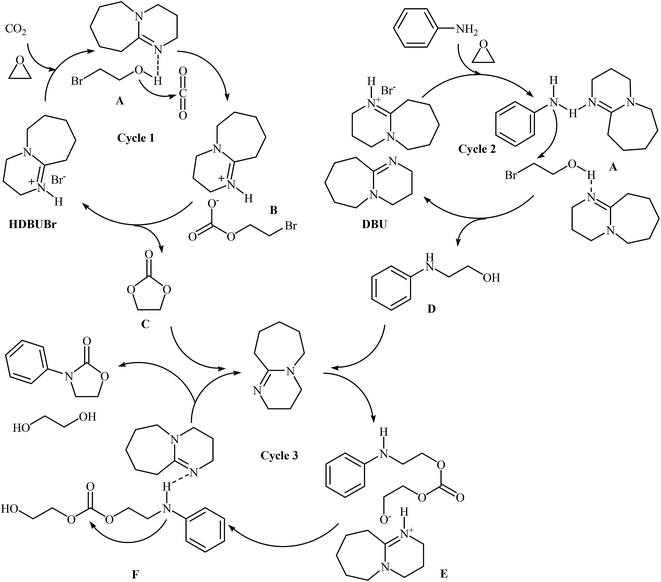 |
| Scheme 20 Mechanism proposed to explain the formation of 2-oxazolidinone 39. | |
Shortly afterwards, in a related investigation, the group of Yuan-Yao described that three component reactions of CO2, terminal epoxides, and primary aromatic amines in the presence of NBu4I/DBU combination as a catalytic system under solvent-free conditions at 115 °C produced the corresponding 2-oxazolidinones in moderate to excellent yields (51–95% for 16 examples).48
Very recently, Sadeghzadeh, Zhiani, and Emrani described the synthesis of Spirulina (Arthrospira platensis) supported ionic liquid by functionalization of S. platensis biomass surface with 1,4-butanesultone.49 The catalytic utility of the ionic liquid was investigated for the carboxylative coupling of epoxides, amines and CO2. Thus, a variety of 3-aryl-2-oxazolidinones 41 were synthesized via the S. platensis/IL catalyzed reaction of ethylene oxide with anilines 40 under the CO2 atmosphere (1 MPa) and solvent-free conditions (Scheme 21). The catalyst was found to be very active, and could be recovered and reused five reaction times without significant loss of activity.
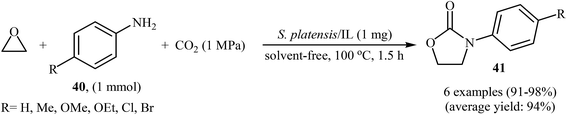 |
| Scheme 21 S. platensis/IL catalyzed synthesis of 3-aryl-2-oxazolidinones 41 from ethylene oxide, anilines 40, and CO2. | |
4.2. Metal-catalyzed reactions
In 2016, the group of Yuan-Yao reported the first protocol for metal-catalyzed three-component cycloaddition of epoxides, amines, and CO2 under solvent-free condition.50 In this investigation, various rare-Earth-metal complexes stabilized by amine-bridged tri-(phenolato) ligands (42–47), co-catalysts (e.g., NBu4I, NBu4Br, NOCt4Br, PPNCl), and additives (e.g., DBU, Et3N, TMEDA, DABCO) were examined and the combination of 46/NBu4Br/DBU as catalytic system at 95 °C was found to be optimal for this transformation. The optimized protocol tolerated a variety of terminal epoxides 48 and both electron-rich and electron-poor anilines 49 and provided the expected 5-substituted-3-aryl-2-oxazolidines 50 in moderate to excellent yields (Scheme 22). However, 2-substituted anilines were incompatible in this reaction. In addition, the reaction did not give good yields with disubstituted epoxides.
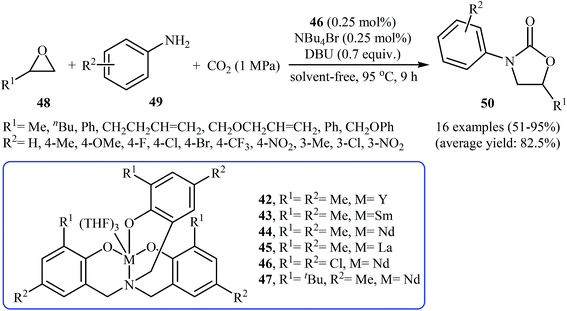 |
| Scheme 22 Yuan's synthesis of 5-substituted-3-aryl-2-oxazolidines 9. | |
Very recently, Sadeghzadeh, Zhiani, and Moradi reported the preparation of KCC-1 supported Cu(II)-β-cyclodextrin [KCC-1/β-CD/Cu(II) NPs] catalyst via functionalization of KCC-1 core–shell by Cu(II)-β-cyclodextrin complex as shown in Scheme 23.51 The catalyst has been fully characterized by various techniques, including TEM, SEM, TGA, FT-IR, ICP-MS, and BET, and its activity in the three-component cycloaddition of ethylene oxide, anilines 51, and CO2 (1 MPa) has been tested under solvent-free conditions and the corresponding 3-aryl-2-oxazolidines 52 were obtained in excellent yields (Scheme 24). It was found that the catalyst was highly reusable and could catalyze ten reaction cycles without detrimental loss of catalytic activity. The proposed mechanism by the authors for this carboxylative coupling reaction is represented in Scheme 25, and starts with the formation of ethylene carbonate intermediate A by the fixation of CO2 onto ethylene oxide. Meanwhile, the nucleophilic attack of aniline 51 on ethylene oxide gives the β-aminoalcohol intermediate B which undergoes addition to intermediate A to furnish intermediate C. Finally, intramolecular cyclization of intermediate C affords the desired 3-aryl-2-oxazolidine 52.
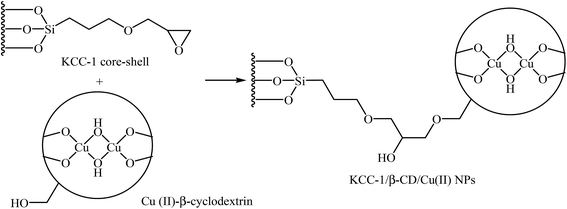 |
| Scheme 23 Schematic illustrations of the synthetic route of KCC-1/β-CD/Cu(II) NPs. | |
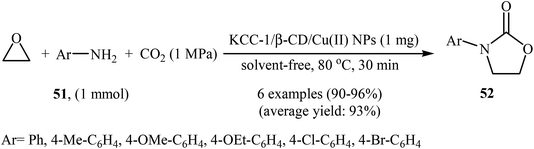 |
| Scheme 24 Cycloaddition of ethylene oxide, anilines 51, and CO2 catalyzed by KCC-1/β-CD/Cu(II) NPs. | |
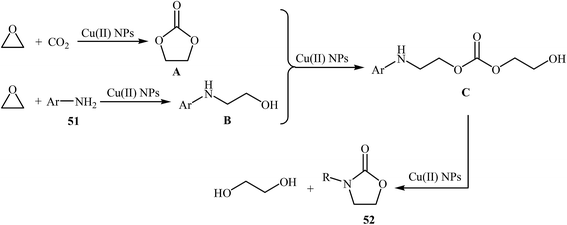 |
| Scheme 25 Plausible mechanism for formation of 3-aryl-2-oxazolidine 52. | |
5. Cycloaddition of CO2 with propargylic alcohols and primary amines
This section surveys literature methods for the synthesis of 5-methylene-2-oxazolidinones through the cycloaddition of CO2 with propargylic alcohols and primary amines under solvent-free conditions. The section is divided into two subsections. The first focuses exclusively on metal-catalyzed reactions, while the second covers catalyst-free reactions.
5.1. Catalytic reactions
In 2009, Jiang and Zhao developed a silver-catalyzed approach to synthesize 2-oxazolidinones 55 through cycloaddition of CO2 with propargylic alcohols 53 and primary amines 54 under solvent-free conditions.52 Among the various metal catalysts like CuI, AgOAc, AgBF4, Ag2CO3; AgOAc was the most efficient for this transformation. Under optimized conditions [CO2 (8 MPa), AgOAc (5 mol%), 120 °C], the corresponding 4-alkylene-1,3-oxazolidin-2-ones 55 were obtained in good to excellent yields (Scheme 26). Interestingly, all the three kinds of internal propargylic alcohols (primary, secondary and tertiary propargylic alcohols) and various primary aliphatic amines were applicable to this reaction. However, internal propargylic alcohols with α-hydrogen whose R3 was alkyl group failed to enter into the reaction. A plausible mechanism that explains this transformation is depicted in Scheme 27 and involves the following steps: (i) initial formation of a propargylic carbonate intermediate A through the reaction of CO2 with propargylic alcohol 53; (ii) intramolecular 5-exo-cyclization of intermediate A to afford the α-alkylene cyclic carbonate B; (iii) nucleophilic addition of primary amine 54 to intermediate B to give 2- oxoalkylcarbamate C; (iv) intramolecular cyclization of C to provide 4-hydroxy cyclic carbamate D; and (v) dehydration of D to form the final product 55. In a related study, Zhao and colleagues reported the use of inexpensive commercially available CuCl as catalyst for three-component coupling of atmospheric CO2, terminal propargylic alcohols, and primary amines at 60 °C under solvent-free conditions.53
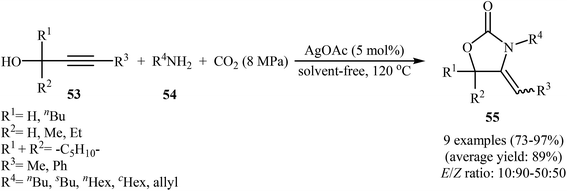 |
| Scheme 26 Ag-catalyzed three-component coupling of propargylic alcohols 53, amines 54, and CO2 reported by Jiang and Zhao. | |
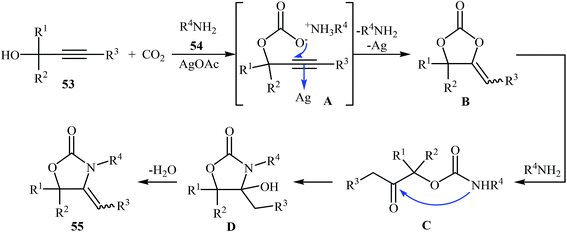 |
| Scheme 27 Mechanistic proposal for the formation of 2-oxazolidinones 55. | |
Later, tertiary terminal propargylic alcohols 56 and primary amines 57 have been found to react with 0.5 MPa of CO2 under solvent-free conditions leading to the formation of 5-methylene-2-oxazolidinones 58 with the catalytic system Ag2WO4 (1 mol%)/PPh3 (2 mol%), as depicted in Scheme 28a.54 However, internal propargylic alcohols failed to undergo the cyclization. In addition, sterically hindered primary amines (e.g. tBuNH2) also failed to give 2-oxazolidinone, providing instead low yield of the simple β-oxopropylcarbamate product. Furthermore, in the case of aromatic amines, the undehydrated products were obtained. As shown in Scheme 28b, this bifunctional silver tungstate catalyst simultaneously activate both the propargylic alcohol and CO2.
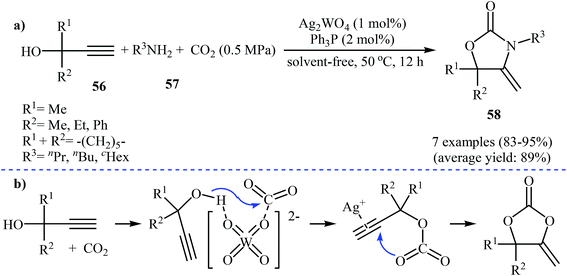 |
| Scheme 28 (a) Ag-catalyzed synthesis of 5-methylene-2-oxazolidinones 58 through three-component reaction of propargylic alcohols 56, primary amines 57, and CO2; (b) cooperative catalytic mechanism by Ag+ and WO42−. | |
Very recently, L.-N. He and colleagues reported one of the most striking examples of the preparation of 2-oxazolidinones 61 via copper-catalyzed three-component cascade reaction of propargylic alcohols 59, aminoethanols 60, and CO2 (Scheme 29).55 The highest conversion efficiency was obtained for the reactions containing CuI (5 mol%), 1,10-phen (5 mol%), and tBuOK (10 mol%) under solvent-free conditions at 80 °C. As shown in Scheme 30, a β-oxopropylcarbamate species was suggested as the key intermediate in this reaction.
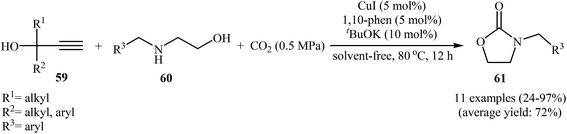 |
| Scheme 29 Cu-catalyzed synthesis of 2-oxazolidinones 61 through three-component cascade reaction of propargylic alcohols 59, aminoethanols 60, and CO2. | |
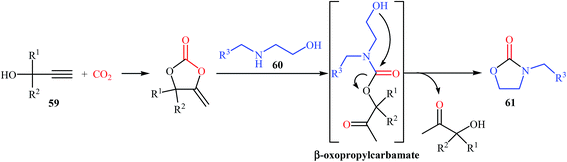 |
| Scheme 30 Mechanism proposed to explain the synthesis of 2-oxazolidinones 61 developed by He. | |
5.2. Catalyst-free reactions
In 2011, Xu, Zhao, and Jia found that synthesis of 4-alkylene-1,3-oxazolidin-2-ones through three-component coupling of propargylic alcohols, primary amines, and CO2, are possible even in the absence of any additional catalyst and organic solvent.56 Thus, treatment of terminal propargylic alcohols 62 with primary aliphatic amines 63 in the presence of pressurized CO2 (14 MPa) at 120 °C afforded 2-oxazolidinones 64 in good to high yields (Scheme 31). It should be mentioned that amine playing a dual role in this transformation; the substrate and the basic catalyst.
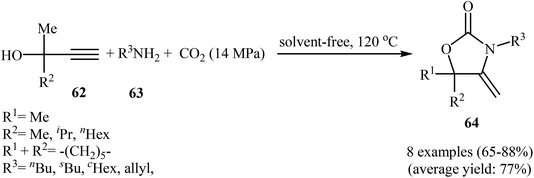 |
| Scheme 31 Catalyst and solvent-free coupling of propargylic alcohols 62, primary amines 63, and CO2. | |
6. Conclusion
Carbon dioxide is not only the primary anthropogenic greenhouse gas, but also plentiful, safe, nontoxic, nonflammable, and renewable C1 resource for producing value-added organic compounds. One of the most promising and environmentally friendly methodologies in this area is the direct synthesis of 2-oxazolidinone derivatives using CO2 as phosgene replacement. However, for the synthesis of titled compounds through CO2 incorporation reactions, organic solvents were usually necessary. Needless to say that utilizing most of the organic solvents cause serious environmental pollution and safety problems. Thus, the development of CO2-based 2-oxazolidinone synthesis under solvent-free conditions is highly desirable from the standpoint of green chemistry. As shown in this review, in recent years, numerous catalytic systems have been developed that could effectively catalyze the solvent-free incorporation of CO2 into 2-oxazolidinones. Interestingly, most of these catalysts could be easily recovered and reused for several reaction runs without observable loss of their catalytic activity and yield, providing more sustainable processes for the chemical fixation of CO2. Despite all these successes, most of the reactions covered here have been carried out at high reaction temperature and/or high CO2 pressure. Thus, there is still further need for the discovery of novel and truly efficient catalytic systems, which can allow the CO2-based 2-oxazolidinone synthesis under milder conditions. We hope that this review will stimulate further thinking and growth in the domain.
Conflicts of interest
There are no conflicts to declare.
Acknowledgements
Authors wish to acknowledge from Dr Shahriar Sarhandi for his scientific comment on our manuscript.
References
-
(a) M. A. Ciufolini, Farmaco, 2005, 60, 627–641 CrossRef CAS PubMed;
(b) J. P. Michael, Nat. Prod. Rep., 2008, 25, 166–187 RSC;
(c) M. Ishikura, T. Abe, T. Choshi and S. Hibino, Nat. Prod. Rep., 2013, 30, 694–752 RSC.
-
(a) V. Bhardwaj, D. Gumber, V. Abbot, S. Dhiman and P. Sharma, RSC Adv., 2015, 5, 15233–15266 RSC;
(b) A. Marella, O. P. Tanwar, R. Saha, M. R. Ali, S. Srivastava, M. Akhter, M. Shaquiquzzaman and M. M. Alam, Saudi Pharm. J., 2013, 21, 1–12 CrossRef PubMed;
(c) N. Kaushik, N. Kaushik, P. Attri, N. Kumar, C. Kim, A. Verma and E. Choi, Molecules, 2013, 18, 6620–6662 CrossRef CAS PubMed;
(d) M. Baumann and I. R. Baxendale, Beilstein J. Org. Chem., 2013, 9, 2265–2319 CrossRef PubMed;
(e) E. Vessally and M. Abdoli, J. Iran. Chem. Soc., 2016, 13, 1235–1256 CrossRef CAS;
(f) M. Abdoli, A. Angeli, M. Bozdag, F. Carta, A. Kakanejadifard, H. Saeidian and C. T. Supuran, J. Enzyme Inhib. Med. Chem., 2017, 32, 1071–1078 CrossRef CAS PubMed.
- C. Lamberth and J. Dinges, The significance of heterocycles for pharmaceuticals and agrochemicals, Bioactive Heterocyclic Compound Classes: Pharmaceuticals, 2012, pp. 1–20 Search PubMed.
- A. Gomtsyan, Chem. Heterocycl. Compd., 2012, 48, 7–10 CrossRef CAS.
-
(a) B. Charlesworth and A. J. Dowson, Expert Opin. Pharmacother., 2002, 3, 993–1005 CrossRef PubMed;
(b) P. Rosenzweig, A. Patat, O. Curet, G. Durrieu, C. Dubruc, I. Zieleniuk and E. Legangneux, J. Affective Disord., 1998, 51, 305–312 CrossRef CAS;
(c) H. Kapfhammer, P. Hoff, H. Golling, E. Rüther and M. Schmauss, Pharmacopsychiatry, 1986, 19, 247–248 CrossRef;
(d) J. Kan, A. Malone and M. S. Benedetti, J. Pharm. Pharmacol., 1978, 30, 190–192 CrossRef CAS PubMed.
- I. Altuntas, N. Delibas, D. Doguc, S. Ozmen and F. Gultekin, Toxicol. In Vitro, 2003, 17, 153–157 CrossRef CAS PubMed.
-
(a) D. K. Hutchinson, Curr. Top. Med. Chem., 2003, 3, 1021–1042 CrossRef CAS PubMed;
(b) D. Shinabarger, Expert Opin. Invest. Drugs, 1999, 8, 1195–1202 CrossRef CAS PubMed.
- M. M. Heravi and V. Zadsirjan, Tetrahedron: Asymmetry, 2013, 24, 1149–1188 CrossRef CAS.
- L. Aurelio, R. T. Brownlee and A. B. Hughes, Chem. Rev., 2004, 104, 5823–5846 CrossRef CAS PubMed.
-
(a) L. N. Pridgen, J. Prol Jr, B. Alexander and L. Gillyard, J. Org. Chem., 1989, 54, 3231–3233 CrossRef CAS;
(b) P. G. Wuts and L. E. Pruitt, Synthesis, 1989, 622–623 CrossRef CAS;
(c) Y. Wu and X. Shen, Tetrahedron: Asymmetry, 2000, 11, 4359–4363 CrossRef CAS.
-
(a) J.-M. Liu, X.-G. Peng, J.-H. Liu, S.-Z. Zheng, W. Sun and C.-G. Xia, Tetrahedron Lett., 2007, 48, 929–932 CrossRef CAS;
(b) X. Peng, F. Li and C. Xia, Synlett, 2006, 1161–1164 CAS.
- E. Vessally, M. Nikpasand, S. Ahmadi, P. D. K. Nezhad and A. Hosseinian, J. Iran. Chem. Soc., 2019, 16, 617–627 CrossRef CAS.
-
(a) N. Kindermann, T. Jose and A. W. Kleij, Top. Curr. Chem., 2017, 375, 15 CrossRef PubMed;
(b) X. Liu and L.-N. He, Top. Curr. Chem., 2017, 375, 21 CrossRef PubMed;
(c) J. E. Gómez and A. W. Kleij, Curr. Opin. Green. Sustain. Chem., 2017, 3, 55–60 CrossRef;
(d) Q.-W. Song, Z.-H. Zhou and L.-N. He, Green Chem., 2017, 19, 3707–3728 RSC;
(e) N. A. Tappe, R. M. Reich, V. D'Elia and F. E. Kühn, Dalton Trans., 2018, 47, 13281–13313 RSC;
(f) Z. Zhang, J.-H. Ye, D.-S. Wu, Y.-Q. Zhou and D.-G. Yu, Chem.–Asian J., 2018, 13, 2292–2306 CrossRef CAS PubMed.
-
(a) E. Vessally, S. Soleimani-Amiri, A. Hosseinian, L. Edjlali and M. Babazadeh, J. CO2 Util., 2017, 21, 342–352 CrossRef CAS;
(b) E. Vessally, M. Babazadeh, A. Hosseinian, S. Arshadi and L. Edjlali, J. CO2 Util., 2017, 21, 491–502 CrossRef CAS;
(c) E. Vessally, R. Mohammadi, A. Hosseinian, L. Edjlali and M. Babazadeh, J. CO2 Util., 2018, 24, 361–368 CrossRef CAS;
(d) S. Farshbaf, L. Z. Fekri, M. Nikpassand, R. Mohammadi and E. Vessally, J. CO2 Util., 2018, 25, 194–204 CrossRef CAS;
(e) K. Didehban, E. Vessally, M. Salary, L. Edjlali and M. Babazadeh, J. CO2 Util., 2018, 23, 42–50 CrossRef CAS;
(f) E. Vessally, A. Hosseinian, M. Babazadeh, L. Edjlali and R. Hosseinzadeh-Khanmiri, Curr. Org. Chem., 2018, 22, 315–322 CrossRef CAS;
(g) M. Daghagheleh, M. Vali, Z. Rahmani, S. Sarhandi and E. Vessally, Chem. Rev. Lett., 2018, 1, 23–30 Search PubMed;
(h) S. Sarhandi, M. Daghagheleh, M. Vali, R. Moghadami and E. Vessally, Chem. Rev. Lett., 2018, 1, 9–15 Search PubMed;
(i) S. Mohammadi, M. Musavi, F. Abdollahzadeh, S. Babadoust and A. Hosseinian, Chem. Rev. Lett., 2018, 1, 49–55 CrossRef.
- S. Pulla, C. M. Felton, P. Ramidi, Y. Gartia, N. Ali, U. B. Nasini and A. Ghosh, J. CO2 Util., 2013, 2, 49–57 CrossRef CAS.
- X.-F. Liu, M.-Y. Wang and L.-N. He, Curr. Org. Chem., 2017, 21, 698–707 CrossRef CAS.
-
(a) S. Arshadi, E. Vessally, M. Sobati, A. Hosseinian and A. Bekhradnia, J. CO2 Util., 2017, 19, 120–129 CrossRef CAS;
(b) S. Arshadi, E. Vessally, A. Hosseinian, S. Soleimani-amiri and L. Edjlali, J. CO2 Util., 2017, 21, 108–118 CrossRef CAS;
(c) A. Hosseinian, S. Farshbaf, R. Mohammadi, A. Monfared and E. Vessally, RSC Adv., 2018, 8, 17976–17988 RSC;
(d) A. Hosseinian, S. Ahmadi, R. Mohammadi, A. Monfared and Z. Rahmani, J. CO2 Util., 2018, 27, 381–389 CrossRef CAS;
(e) E. Vessally, A. Hosseinian, L. Edjlali, M. Babazadeh and K. Didehban, Mini-Rev. Org. Chem., 2018, 15, 315–323 CrossRef CAS;
(f) S. Shahidi, P. Farajzadeh, P. Ojaghloo, A. Karbakhshzadeh and A. Hosseinian, Chem. Rev. Lett., 2018, 1, 37–44 Search PubMed;
(g) S. Farshbaf, L. Sreerama, T. Khodayari and E. Vessally, Chem. Rev. Lett., 2018, 1, 56–67 Search PubMed.
- E. L. Baker, J. Occup. Med., 1994, 36, 1079–1092 CrossRef CAS.
- K. Tanaka and F. Toda, Chem. Rev., 2000, 100, 1025–1074 CrossRef CAS PubMed.
- M. A. Martins, C. P. Frizzo, D. N. Moreira, L. Buriol and P. Machado, Chem. Rev., 2009, 109, 4140–4182 CrossRef CAS PubMed.
- M. S. Singh and S. Chowdhury, RSC Adv., 2012, 2, 4547–4592 RSC.
-
(a) F. M. Ismail, D. O. Levitsky and V. M. Dembitsky, Eur. J. Med. Chem., 2009, 44, 3373–3387 CrossRef CAS PubMed;
(b) G. S Singh, Mini-Rev. Med. Chem., 2016, 16, 892–904 CrossRef.
-
(a) L. Degennaro, P. Trinchera and R. Luisi, Chem. Rev., 2014, 114, 7881–7929 CrossRef CAS PubMed;
(b) A. Padwa and S. S. Murphree, ARKIVOC, 2006, 3, 6–33 Search PubMed.
- Y. Du, Y. Wu, A.-H. Liu and L.-N. He, J. Org. Chem., 2008, 73, 4709–4712 CrossRef CAS PubMed.
- Z.-Z. Yang, L.-N. He, S.-Y. Peng and A.-H. Liu, Green Chem., 2010, 12, 1850–1854 RSC.
- Y.-N. Zhao, Z.-Z. Yang, S.-H. Luo and L.-N. He, Catal. Today, 2013, 200, 2–8 CrossRef CAS.
- R. A. Watile, D. B. Bagal, K. M. Deshmukh, K. P. Dhake and B. M. Bhanage, J. Mol. Catal. A: Chem., 2011, 351, 196–203 CrossRef CAS.
- Y. Chen, R. Luo, Z. Yang, X. Zhou and H. Ji, Sustainable Energy Fuels, 2018, 2, 125–132 RSC.
- H.-F. Jiang, J.-W. Ye, C.-R. Qi and L.-B. Huang, Tetrahedron Lett., 2010, 51, 928–932 CrossRef CAS.
- X.-Y. Dou, L.-N. He and Z.-Z. Yang, Synth. Commun., 2012, 42, 62–74 CrossRef CAS.
- D. B. Nale, S. Rana, K. Parida and B. M. Bhanage, Appl. Catal., A, 2014, 469, 340–349 CrossRef CAS.
- V. B. Saptal and B. M. Bhanage, ChemSusChem, 2016, 9, 1980–1985 CrossRef CAS PubMed.
- W. Chen, L.-x. Zhong, X.-w. Peng, R.-c. Sun and F.-C. Lu, ACS Sustainable Chem. Eng., 2014, 3, 147–152 CrossRef.
- Y. Wu, L.-N. He, Y. Du, J.-Q. Wang, C.-X. Miao and W. Li, Tetrahedron, 2009, 65, 6204–6210 CrossRef CAS.
- X.-Z. Lin, Z.-Z. Yang, L.-N. He and Z.-Y. Yuan, Green Chem., 2015, 17, 795–798 RSC.
- R. Luo, Z. Yang, W. Zhang, X. Zhou and H. Ji, Sci. China: Chem., 2017, 60, 979–989 CrossRef CAS.
- T. Y. Ma and S. Z. Qiao, ACS Catal., 2014, 4, 3847–3855 CrossRef CAS.
- H. Xu, X. F. Liu, C. S. Cao, B. Zhao, P. Cheng and L. N. He, Adv. Sci., 2016, 3, 1600048 CrossRef PubMed.
- K. Lauder, A. Toscani, N. Scalacci and D. Castagnolo, Chem. Rev., 2017, 117, 14091–14200 CrossRef CAS PubMed.
-
(a) E. Vessally, RSC Adv., 2016, 6, 18619–18631 RSC;
(b) E. Vessally, L. Edjlali, A. Hosseinian, A. Bekhradnia and M. D. Esrafili, RSC Adv., 2016, 6, 49730–49746 RSC;
(c) E. Vessally, A. Hosseinian, L. Edjlali, A. Bekhradnia and M. D. Esrafili, RSC Adv., 2016, 6, 71662–71675 RSC;
(d) E. Vessally, A. Hosseinian, L. Edjlali, A. Bekhradnia and M. D Esrafili, Curr. Org. Synth., 2017, 14, 557–567 CrossRef CAS;
(e) E. Vessally, A. Hosseinian, L. Edjlali, A. Bekhradnia and M. D. Esrafili, RSC Adv., 2016, 6, 99781–99979 RSC;
(f) E. Vessally, S. Soleimani-Amiri, A. Hosseinian, L. Edjlali and A. Bekhradnia, RSC Adv., 2017, 7, 7079–7091 RSC;
(g) M. Babazadeh, S. Soleimani-Amiri, E. Vessally, A. Hosseinian and L. Edjlali, RSC Adv., 2017, 7, 43716–43736 RSC;
(h) S. Arshadi, E. Vessally, L. Edjlali, R. Hosseinzadeh-Khanmiri and E. Ghorbani-Kalhor, Beilstein J. Org. Chem., 2017, 13, 625–638 CrossRef CAS PubMed;
(i) E. Vessally, A. Hosseinian, L. Edjlali, E. Ghorbani-Kalhor and R. Hosseinzadeh-Khanmiri, J. Iran. Chem. Soc., 2017, 14, 2339–2353 CrossRef CAS;
(j) S. Arshadi, E. Vessally, L. Edjlali, E. Ghorbani-Kalhor and R. Hosseinzadeh-Khanmiri, RSC Adv., 2017, 7, 13198–13211 RSC;
(k) E. Vessally, A. Hosseinian, L. Edjlali, M. Babazadeh and R. Hosseinzadeh-Khanmiri, Iran. J. Chem. Chem. Eng., 2017, 36, 1–13 CAS;
(l) E. Vessally, R. Hosseinzadeh-Khanmiri, E. Ghorbani-Kalhor, M. Es' haghi and A. Bekhradnia, RSC Adv., 2017, 7, 19061–19072 RSC;
(m) E. Vessally, M. Babazadeh, K. Didehban, A. Hosseinian and L. Edjlali, Curr. Org. Chem., 2018, 22, 286–297 CrossRef CAS;
(n) E. Vessally, M. Babazadeh, K. Didehban, A. Hosseinian and L. Edjlali, Curr. Org. Chem., 2017, 21, 2561–2572 CrossRef CAS;
(o) S. Soleimani-Amiri, E. Vessally, M. Babazadeh, A. Hosseinian and L. Edjlali, RSC Adv., 2017, 7, 28407–28418 RSC;
(p) E. Vessally, M. Babazadeh, A. Hosseinian, L. Edjlali and L. Sreerama, Curr. Org. Chem., 2018, 22, 199–205 CrossRef CAS.
- M.-Y. Wang, Q.-W. Song, R. Ma, J.-N. Xie and L.-N. He, Green Chem., 2016, 18, 282–287 RSC.
- S. M. Sadeghzadeh, R. Zhiani and S. Emrani, Appl. Organomet. Chem., 2018, 32, e3941 CrossRef.
- S. M. Saadati and S. M. Sadeghzadeh, Catal. Lett., 2018, 148, 1692–1702 CrossRef CAS.
- A. Liu, L. He, S. Peng, Z. Pan, J. Wang and J. Gao, Sci. China: Chem., 2010, 53, 1578–1585 CrossRef CAS.
- N.-K. Kim, H. Sogawa, M. D. Felicia and T. Takata, Polym. J., 2019, 51, 351–357 CrossRef CAS.
- B. Wang, E. H. Elageed, D. Zhang, S. Yang, S. Wu, G. Zhang and G. Gao, ChemCatChem, 2014, 6, 278–283 CrossRef CAS.
- B. Wang, Z. Luo, E. H. Elageed, S. Wu, Y. Zhang, X. Wu, F. Xia, G. Zhang and G. Gao, ChemCatChem, 2016, 8, 830–838 CrossRef CAS.
- M. Lv, P. Wang, D. Yuan and Y. Yao, ChemCatChem, 2017, 9, 4451–4455 CrossRef CAS.
- S. M. Sadeghzadeh, R. Zhiani and S. Emrani, Catal. Lett., 2018, 148, 119–124 CrossRef CAS.
- B. Xu, P. Wang, M. Lv, D. Yuan and Y. Yao, ChemCatChem, 2016, 8, 2466–2471 CrossRef CAS.
- S. M. Sadeghzadeh, R. Zhiani and M. Moradi, ChemistrySelect, 2018, 3, 3516–3522 CrossRef CAS.
- H.-F. Jiang and J.-W. Zhao, Tetrahedron Lett., 2009, 50, 60–62 CrossRef CAS.
- J. Xu, J. Zhao, Z. Jia and J. Zhang, Synth. Commun., 2011, 41, 858–863 CrossRef CAS.
- Q.-W. Song, B. Yu, X.-D. Li, R. Ma, Z.-F. Diao, R.-G. Li, W. Li and L.-N. He, Green Chem., 2014, 16, 1633–1638 RSC.
- X. D. Li, Y. Cao, R. Ma and L. N. He, J. CO2 Util., 2018, 25, 338–345 CrossRef CAS.
- J. X. Xu, J. W. Zhao and Z. B. Jia, Chin. Chem. Lett., 2011, 22, 1063–1066 CrossRef CAS.
|
This journal is © The Royal Society of Chemistry 2019 |
Click here to see how this site uses Cookies. View our privacy policy here.