DOI:
10.1039/C9RA00505F
(Paper)
RSC Adv., 2019,
9, 12209-12217
Fluorometric enhancement of the detection of H2O2 using different organic substrates and a peroxidase-mimicking polyoxometalate†
Received
21st January 2019
, Accepted 1st April 2019
First published on 17th April 2019
Abstract
Simple, sensitive and stable fluorometric sensors based on the polyoxotungstate intrinsic peroxidase (Na10[α-SiW9O34]) induced fluorescent enhancement of benzoic acid (BA), thiamine (TH) and 3-(4-hydroxyphenyl)propionic acid (HPPA) for the detection of hydrogen peroxide (H2O2) are developed for the first time. In three assays, the three non-fluorescent substrates BA, TH and HPPA were oxidized with the ·OH radicals decomposed from H2O2 under the catalysis of Na10[α-SiW9O34] under basic pH conditions. The optimal conditions for the detection of H2O2 were evaluated and possible mechanisms are also discussed. The fluorescence intensity increases were linearly related to the concentration of H2O2 in the ranges 1 × 10−8 to 1.6 × 10−6, 1.6 × 10−6 to 1 × 10−4, and 1 × 10−5to 2.5 × 10−4 M with BA, TH, and HPPA as substrates, respectively. Detection limits for the three systems were found to be 6.7 × 10−9, 2.2 × 10−7 and 9.6 × 10−6 M (3σ), respectively. The RSD values ranged from 2.57% to 4.66%, 0.82% to 4.06%, and 1.08% to 2.75%, respectively. The rates of recoveries were between 99.73% and 113.06%, 95.20% and 104.22%, and 95.28% and 128.76%, respectively. Moreover, the effects of interference were studied. The proposed work was successfully applied to the determination of H2O2 in water and a sensitive, rapid and easy to operate assay was built, which has great potential applications in environmental science.
1. Introduction
Hydrogen peroxide exists widely in biological systems, food production, and pharmaceutical, industrial and environmental applications.1–3 It is a natural product in oxidative metabolic processes in biology, being harmful to organisms when the concentration of H2O2 reaches 0.5 mmol L−1.4 It is also a source of toxic oxygen that produces ·OH radicals,5 which can lead to disease and senescence in a body. Therefore, the trace determination of H2O2 is very important in environmental analysis, biochemical analysis and clinical diagnostics. In recent years, various methods for the determination of H2O2 have been developed, such as fluorometry,6 spectrophotometry,7,8 chemiluminescence,9 liquid chromatography10 and electrochemistry.11,12 Among these methods, the horseradish peroxidase (HRP)-catalyzed reaction is the most widely used enzymatic reaction.13 The characteristics of the enzyme have been systematically studied with H2O2 as an oxidizing agent and in the presence of various substances as fluorogenic substrates.4 Although HRP has high specificity and sensitivity, its instability and high cost restrict its application.14 Compared to HRP, artificial enzyme mimics are more economic due to their high thermal stability, high tolerance of practical conditions, good adaptability to abiotic/biotic reactions and low cost of preparation and purification.
The development of artificial enzyme mimics as well as their expanding applications in various fields, such as pharmaceuticals, fine-chemical syntheses, chemical and biological sensing, and proteome analyses, have been actively pursued for decades.15 To date, a large number of artificial enzymes have been explored to mimic the structures and functions of naturally occurring enzymes including cyclodextrins, metal complexes, porphyrins, polymers, dendrimers, biomolecules and nanomaterials.16–28 Among these, polyoxometalates have aroused special attention due to their mimicking enzyme activity towards peroxidase substrates. Polyoxometalates (POMs) are a large family of inorganic metal-oxide cluster compounds with many remarkable physical and chemical properties and biological activities.29,30 In particular, POMs undergo a fast and reversible multi-electron redox process without any significant structural changes, that makes them useful as redox sensors. Based on the outstanding properties of POMs, POMs and their hybrids have been utilized in a colorimetric assay of HepG2 cells, H2O2 and glucose.31–38 However, by far the majority of POMs with intrinsic peroxidase-like activity are stable under acid aqueous conditions. While the complex practical conditions for H2O2 detection require high temperature and alkalinity (20–98 °C, pH ≥ 7),7,10 which hamper the applications of POMs. In a recent paper, we first reported the fluorescent quenching of CdTe quantum dots for the measurement of H2O2 with catalysis by Na10[α-SiW9O34] under basic pH aqueous conditions.39 Considering the HPR enzyme-mimicking properties of Na10[α-SiW9O34], new fluorometric methods based on it for the detection of H2O2 and several fluorogenic substrates under alkaline conditions can subsequently be developed. Additionally, more research is needed into the effects of the POM enzyme-mimicking reaction for biological and chemical analysis.
From a combination of the above reasons, herein we design for the first time new fluorometric enhancement methods that explore the catalytic effect of Na10[α-SiW9O34] to convert weakly fluorescent substrates into strongly fluorescent substrates in the presence of H2O2. Benzoic acid (BA),13 thiamine (TH)40 and 3-(4-hydroxyphenyl)propionic acid (HPPA)41 have been employed as fluorogenic substrates for hydrogen peroxide detection via the formation of fluorescent products. In this fluorometric assay, different analytical parameters, such as concentration of substrates and Na10[α-SiW9O34], buffer pH, and reaction time were optimized. The catalysis activities and kinetic mechanics of Na10[α-SiW9O34] were investigated upon the reaction of hydrogen peroxide with its oxidized substrates, BA, TH and HPPA. Under the optimal reaction conditions, the detection system of BA–Na10[α-SiW9O34]–H2O2 shows the most sensitive response to H2O2 over the other two systems. The present assays have been successfully applied to the determination of H2O2 in water.
2. Experimental section
2.1. Reagents and chemicals
All the chemicals used were of analytical grade without further purification. Benzoic acid (BA), thiamine (TH), 3-(4-hydroxyphenyl)propionic acid (HPPA) and Na2WO4·2H2O were obtained from the Guoyao Chemical Research Institute (Shenyang, China). Na2SiO3 was obtained from Sinopharm Chemical Reagent Co., Ltd. (Shanghai, China). Na3PO4, NaH2PO4, NaCl, HCl, Na2CO3 and hydrogen peroxide (H2O2, 30%) were purchased from Beijing Chemical Works (Beijing, China). The water used in the experiments was purified. The ρ of the water was 18 MΩ cm.
2.2. Instrumentation
The fluorescence measurements were performed on a Shimadzu RF-5301 PC fluorescence spectrofluorophotometer (Kyoto, Japan). A 1 cm path length quartz cuvette was used in the experiment. The widths of the excitation and emission slits were set to 5.0 and 5.0 nm, respectively. The Fourier Transform Infrared (FTIR) spectrum was recorded in the range 400–4000 cm−1 on KBr (FTIR IRAffinity-1s, Shimadzu, Japan). The pH measurements were performed by a PHS-25 pH meter (Shanghai INESA Scientific Instrument Co. Ltd, China). The purified water was obtained from a SMART-N Heal Force Water Purification System (Shanghai Canrex Analytic Instrument Co., Ltd, Pudong Shanghai China).
2.3. Synthesis of Na10[α-SiW9O34]
Na10[α-SiW9O34] was prepared according to the literature method.42 Briefly, 0.133 mol of Na2WO4·2H2O and 12.5 mmol of Na2SiO3 were dissolved in 200 mL of distilled hot water, and then HCl (6 M, 32.5 mL) was slowly added to the above solution with vigorous stirring for 30 min. The mixture was boiled to a volume of 75 mL and then the filtrate was collected. Then, 3.2 mol of Na2CO3 solution was slowly added into the filtrate under stirring. The precipitate was collected after 1 h. The solid was stirred with 250 mL of 4 M NaCl and dried under vacuum. The characteristic peaks of Na10[α-SiW9O34] (KBr pellet, cm−1) were 987, 936, 869, 836, 705, 655 (Fig. S1†), which are nearly consistent with the values reported in the literature.
2.4. H2O2 detection
100 μL of different substrates (the concentrations of BA, TH, and HPPA were 5, 20, and 80 mmol L−1, respectively), 100 μL of 8 mmol L−1 Na10[α-SiW9O34], 750 μL of 20 mmol L−1 phosphate buffer solution (pH = 11.0) were mixed at room temperature. 50 μL of various concentrations of H2O2 were added into the mixture, sequentially. After the addition of H2O2, the fluorescence values of the above solutions were measured by a fluorescence spectrophotometer (BA: λex = 295 nm, λem = 405 nm. TH: λex = 375 nm, λem = 440 nm. HPPA: λex = 330 nm, λem = 416 nm). The process of H2O2 addition to BA, TH and HPPA with Na10[α-SiW9O34] as catalyst is shown in Scheme 1.
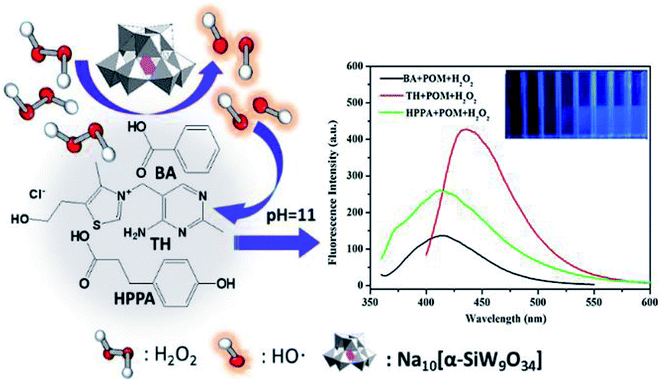 |
| Scheme 1 A schematic illustration of the processes of H2O2 addition to BA, TH and HPPA with Na10[α-SiW9O34] as catalyst. | |
3. Results and discussion
3.1. The peroxidase-like activity of the Na10[α-SiW9O34]
The peroxidase-like activity of Na10[α-SiW9O34] was verified by fluorometric experiments using BA, TH and HPPA as the substrates. Na10[α-SiW9O34] catalyzed the conversion of weakly fluorescent BA to produce fluorescent adduct hydroxylated benzoic acid (OHBA) in the presence of H2O2, which showed strong fluorescence at 405 nm when excited at 295 nm. As shown in Fig. 1A, when Na10[α-SiW9O34] was added into the BA–H2O2 solution, a strong fluorescence peak was obtained. However, there were no strong fluorescence peaks when the solution did not contain H2O2 and/or Na10[α-SiW9O34], which confirmed that the reaction between BA and H2O2 could be catalyzed by Na10[α-SiW9O34]. Thiamine is a non-fluorescent substrate, but thiochrome, the oxidation product of thiamine with H2O2, is strongly fluorescent, showing an excitation maximum at 375 nm and a fluorescence emission maximum at 440 nm. The fluorescence spectrum of the TH–Na10[α-SiW9O34]–H2O2 system is shown in Fig. 1B. In comparison, no fluorescence peaks were observed in the TH, TH–Na10[α-SiW9O34] or TH–H2O2 systems, which confirmed that the reaction between TH and H2O2 could also be catalyzed by Na10[α-SiW9O34]. HPPA is one of the most efficient substrates for evaluating peroxidase activity.43 More specifically, non-fluorescent HPPA can be oxidized by hydrogen peroxide to yield a strong fluorescent dimer via peroxidase catalysis. Following the addition of Na10[α-SiW9O34] to the HPPA–H2O2 system, a strong fluorescence peak was observed, as shown in Fig. 1C. Like the BA and TH systems, no fluorescence peaks were observed in the HPPA, HPPA–Na10[α-SiW9O34] and HPPA–H2O2 systems, which indicated that the reaction between HPPA and H2O2 could be catalyzed by Na10[α-SiW9O34].
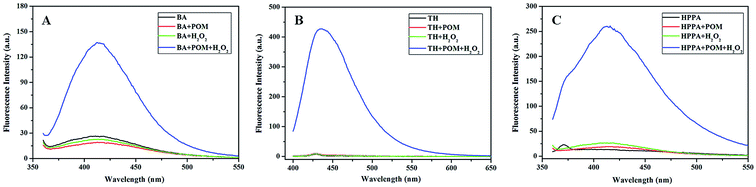 |
| Fig. 1 Fluorescence spectra of the (A) BA–Na10[α-SiW9O34]–H2O2 system, (B) TH–Na10[α-SiW9O34]–H2O2 system and (C) HPPA–Na10[α-SiW9O34]–H2O2 system. Reaction conditions: 8 mmol L−1 Na10[α-SiW9O34]; 100 mmol L−1 H2O2; 5 mmol L−1 BA; 20 mmol L−1 TH; 80 mmol L−1 HPPA; pH, 11.0; temperature, 25 °C; incubation time, 10 min. | |
3.2. Condition optimization
In order to optimize the possible analytical methods for H2O2 determination, the effects of experimental conditions, including reaction time, solution pH, the concentration of substrates, and the concentration of Na10[α-SiW9O34] were investigated.
3.2.1. Effect of reaction time. As shown in Fig. 2A, the fluorescence intensity of the generated OHBA rapidly increases with reaction time initially, and then tends towards saturation after 600 s, which indicates that the added H2O2 is completely consumed by oxidation of the substrate BA. Thus, 10 min was chosen as the optimal reaction time. Fig. 2B shows that the fluorescence intensity of the generated thiochrome changed from 0 to 600 seconds, and then tended towards to a constant after 600 s, indicating that the TH–Na10[α-SiW9O34]–H2O2 solution was stable during this period. Fig. 2C shows the effects of reaction time (0–900 seconds) on the fluorescence intensity of HPPA–Na10[α-SiW9O34] in the presence of H2O2. When H2O2 was added into an HPPA–Na10[α-SiW9O34] aqueous solution, the fluorescence intensity rapidly increased from 0 to 60 seconds, and then tended towards saturation after 600 s, which indicated that the added H2O2 was completely consumed by oxidation of the substrate HPPA. Thus, 10 min was chosen as the optimal reaction time in the three different substrate systems.
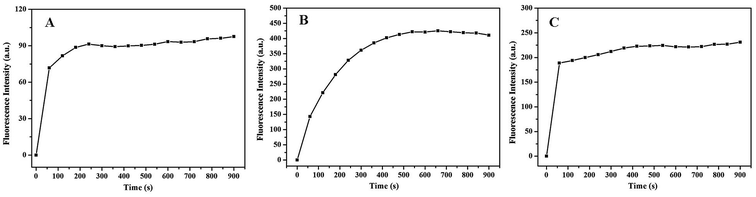 |
| Fig. 2 The effects of reaction time on the fluorescence intensities of the (A) BA–Na10[α-SiW9O34] system, (B) TH–Na10[α-SiW9O34] system and (C) HPPA–Na10[α-SiW9O34] system in the presence of H2O2. Reaction conditions: 8 mmol L−1 Na10[α-SiW9O34]; 100 mmol L−1 H2O2; 5 mmol L−1 BA; 80 mmol L−1 HPPA; pH, 11.0; temperature, 25 °C. | |
3.2.2. Effect of pH. The solution pH is an important factor affecting catalytic efficiency. To investigate the influence of pH on the different substrate–Na10[α-SiW9O34]–H2O2 systems, the fluorescence intensities were examined in buffer solutions with various pH values (5.0–11.0). As shown in Fig. 3, the fluorescence intensities of different substrate–Na10[α-SiW9O34]–H2O2 systems were much faster in basic solutions than in neutral or acidic solutions. Moreover, the BA–Na10[α-SiW9O34]–H2O2 system, TH–Na10[α-SiW9O34]–H2O2 system and HPPA–Na10[α-SiW9O34]–H2O2 system reached their maximum fluorescence intensities when the pH value was 11.0. Therefore, pH 11.0 was selected to be the optimal pH in the different substrate–Na10[α-SiW9O34]–H2O2 systems.
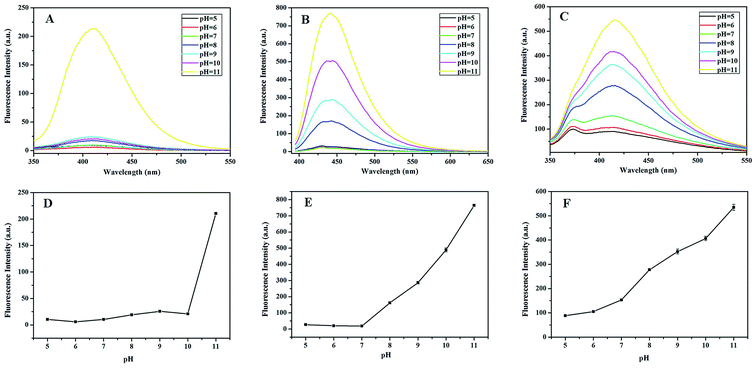 |
| Fig. 3 The effects of pH on the fluorescence intensities of the (A and D) BA–Na10[α-SiW9O34]–H2O2 system, (B and E) TH–Na10[α-SiW9O34]–H2O2 system and (C and F) HPPA–Na10[α-SiW9O34]–H2O2 system. Reaction conditions: 8 mmol L−1 Na10[α-SiW9O34]; 100 mmol L−1 H2O2; 5 mmol L−1 BA; 20 mmol L−1 TH; 80 mmol L−1 HPPA; temperature, 25 °C. | |
3.2.3. Effect of different substrate concentrations. To maximize the activity of Na10[α-SiW9O34], the effects of varying concentrations of the substrates were also investigated. Fig. 4A and D show the influence of BA concentrations on the fluorescence intensity of the reaction solution. For a given concentration of H2O2 (100 mmol L−1), the fluorescence intensity was enhanced with an increase in the BA concentration up to 5 mM, and then slowly increased beyond 5 mM of BA. Hence, 5 mM BA was selected as the optimal concentration. As shown in Fig. 4B and E, the relationship between the fluorescence intensity of the product and the concentration of TH was investigated between 0.3125 and 50 mM. When the concentration of TH reached 20 mM, the fluorescence intensity of the TH–Na10[α-SiW9O34]–H2O2 system reached its maximum. Thus, 20 mM TH was selected as the optimal concentration. As shown in Fig. 4C and F, the fluorescence intensity of the reaction system was enhanced as the concentration of HPPA increased to 80 mM, after which it decreased. Thus, 80 mM HPPA was selected as the optimal concentration.
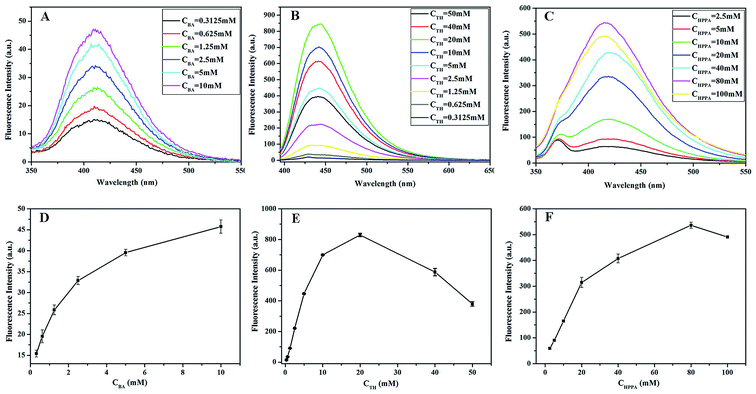 |
| Fig. 4 The effects of different substrate concentrations on the fluorescence intensities of the (A and D) BA–Na10[α-SiW9O34]–H2O2 system, (B and E) TH–Na10[α-SiW9O34]–H2O2 system, and (C and F) HPPA–Na10[α-SiW9O34]–H2O2 system. Reaction conditions: 8 mmol L−1 Na10[α-SiW9O34]; 100 mmol L−1 H2O2; pH, 11.0; temperature, 25 °C. | |
3.2.4. Effect of Na10[α-SiW9O34] concentration. In order to achieve the best performance, we investigated the effect of Na10[α-SiW9O34] concentrations (0.5–12 mM) on the fluorescence intensities of BA, TH and HPPA in the presence of H2O2. As shown in Fig. 5A and B, the fluorescence intensities all increased quickly with an increase in Na10[α-SiW9O34] concentrations up to 8 mM, and then moderately slowly increased beyond 8 mM of Na10[α-SiW9O34] in the BA and TH systems. Whereas, in the HPPA–Na10[α-SiW9O34]–H2O2 system, the fluorescence intensity first increased and then decreased, as shown in Fig. 5C. As shown in Fig. 5, when the concentration of Na10[α-SiW9O34] reached 8 mM, the effect of Na10[α-SiW9O34] on the fluorescence intensity in different substrate–Na10[α-SiW9O34]–H2O2 systems achieved the best activity. Thus, aiming to obtain the best catalytic activity of Na10[α-SiW9O34] and low economic cost, 8 mM Na10[α-SiW9O34] was selected as the optimal concentration.
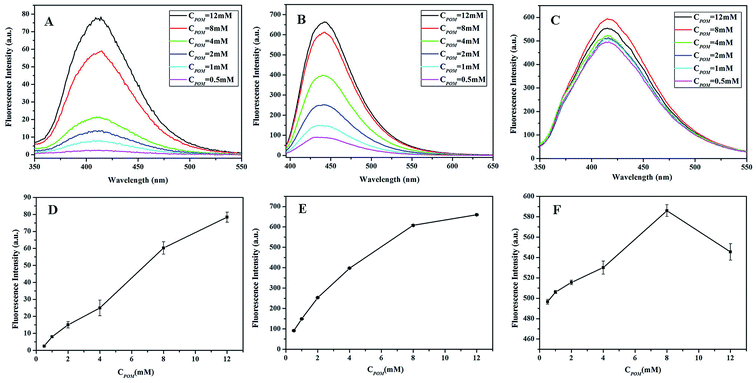 |
| Fig. 5 The effects of Na10[α-SiW9O34] concentrations on the fluorescence intensities of the (A and D) BA–Na10[α-SiW9O34]–H2O2 system, (B and E) TH–Na10[α-SiW9O34]–H2O2 system and (C and F) HPPA–Na10[α-SiW9O34]–H2O2 system. Reaction conditions: 100 mmol L−1 H2O2; 5 mmol L−1 BA; 20 mmol L−1 TH; 80 mmol L−1 HPPA; pH, 11.0; temperature, 25 °C. | |
3.3. Steady-state kinetic assay
For a further understanding of the influence of different substrates on the catalytic mechanism of Na10[α-SiW9O34] in the presence of H2O2, steady-state kinetic assays for different substrates were determined in detail. As shown in Fig. 6, the typical Michaelis–Menten curve was obtained for Na10[α-SiW9O34] in the presence of H2O2. Michaelis–Menten constant (KM) and maximum initial velocity (Vmax) were counted from the Michaelis–Menten curve using a Lineweaver–Burk plot. The kinetic parameters of BA, TH, and HPPA are given in Table 1. The KM of Na10[α-SiW9O34] with BA, TH and HPPA as substrates were 6.03 × 10−4, 7.95 × 10−3 and 2.12 × 10−2 M, respectively. The Vmax of Na10[α-SiW9O34] with BA, TH, and HPPA as substrates were 4.06 × 10−4, 3.68 × 10−5 and 5.75 × 10−5 M s−1, respectively. The KM of BA, TH, and HPPA using Na10[α-SiW9O34] as a catalyst with H2O2 as the substrate were 3.75 × 10−7, 8.31 × 10−5 and 2.51 × 10−2 M, respectively. The Vmax of BA, TH, and HPPA using Na10[α-SiW9O34] as a catalyst with H2O2 as the substrate were 5.82 × 10−4, 3.02 × 10−5 and 3.85 × 10−5 M s−1, respectively. The KM value of Na10[α-SiW9O34] with BA as the substrate was apparently lower than those values in the TH and HPPA systems. This indicated that BA has a higher affinity to Na10[α-SiW9O34] in comparison with TH and HPPA. The apparent KM value of BA with H2O2 as the substrate was apparently lower than those values in TH and HPPA. It was clear that BA has a higher affinity for H2O2 compared with those of TH and HPPA.
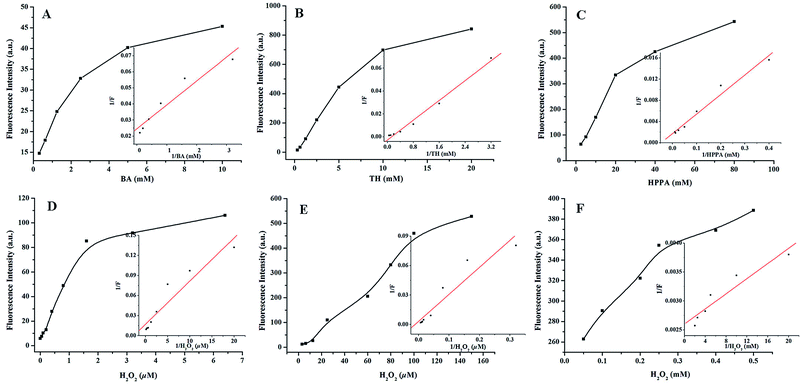 |
| Fig. 6 The steady-state kinetic assays and catalytic mechanisms of Na10[α-SiW9O34] as a catalyst in different substrates: (A and D) BA–Na10[α-SiW9O34]–H2O2 system, (B and E) TH–Na10[α-SiW9O34]–H2O2 system and (C and F) HPPA–Na10[α-SiW9O34]–H2O2 system. Reaction conditions: 8 mmol L−1 Na10[α-SiW9O34]; pH, 11.0; temperature, 25 °C; incubation time, 10 min. | |
Table 1 Comparison of the KM and Vmax values of BA, TH and HPPA using Na10[α-SiW9O34] as a catalyst
Enzyme |
Substrate |
KM (M) |
Vmax (M S−1) |
Na10[α-SiW9O34] |
BA |
6.03 × 10−4 |
4.06 × 10−4 |
Na10[α-SiW9O34] |
H2O2 |
3.75 × 10−7 |
5.82 × 10−4 |
Na10[α-SiW9O34] |
TH |
7.95 × 10−3 |
3.68 × 10−5 |
Na10[α-SiW9O34] |
H2O2 |
8.31 × 10−5 |
3.02 × 10−5 |
Na10[α-SiW9O34] |
HPPA |
2.12 × 10−2 |
5.75 × 10−5 |
Na10[α-SiW9O34] |
H2O2 |
2.51 × 10−2 |
3.85 × 10−5 |
3.4. Calibration curve for H2O2 detection
Under the optimized reaction conditions, the relationships between the fluorescence intensities and H2O2 concentrations in the BA–Na10[α-SiW9O34]–H2O2, TH–Na10[α-SiW9O34]–H2O2 and HPPA–Na10[α-SiW9O34]–H2O2 systems were investigated. As shown in Fig. 7D, the fluorescence intensity of BA–Na10[α-SiW9O34]–H2O2 increased linearly with increasing concentrations of H2O2. The linear regression equation was as follows: Y = 4.45 + 5.22 × 107 × CH2O2 with a correlation coefficient of 0.97403; the linear range was from 1 × 10−8 to 1.6 × 10−6 M. The lower limit of detection (3σ, LOD) of Na10[α-SiW9O34] for H2O2 was found to be 6.7 × 10−9 M. As shown in Fig. 7E, the fluorescence intensity of TH–Na10[α-SiW9O34]–H2O2 increased linearly with increasing concentrations of H2O2. The linear regression equation was as follows: Y = 8.41 + 4.35 × 106 × CH2O2 with a correlation coefficient of 0.99049; the linear range was from 1.6 × 10−6 to 1 × 10−4 M. The LOD of the Na10[α-SiW9O34] for H2O2 was found to be 2.2 × 10−7 M. Moreover, as shown in Fig. 7F, the fluorescence intensity of HPPA–Na10[α-SiW9O34]–H2O2 increased linearly with increasing concentration of H2O2. The linear regression equation was as follows: Y = 237.55 + 4.60 × 105 × CH2O2 with a correlation coefficient of 0.98489; the linear range was from 1 × 10−5 to 2.5 × 10−4 M. The LOD of Na10[α-SiW9O34] for H2O2 was found to be 9.6 × 10−6 M. Comparing these systems for the determination of H2O2, as shown in Table 2, the proposed method is superior in its low detection limit.
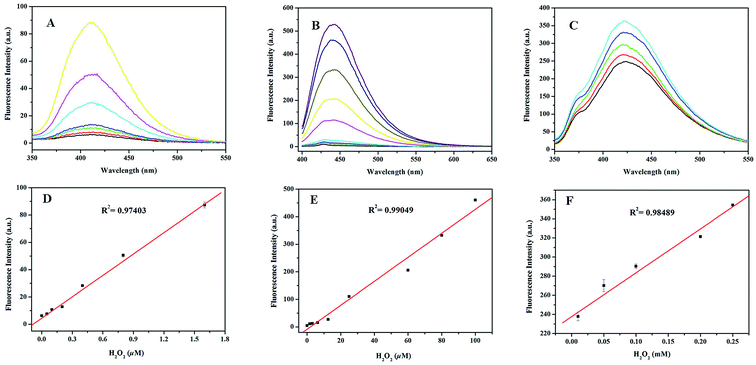 |
| Fig. 7 Linear calibration plots for H2O2: (A and D) BA–Na10[α-SiW9O34]–H2O2 system, (B and E) TH–Na10[α-SiW9O34]–H2O2 system, and (C and F) HPPA–Na10[α-SiW9O34]–H2O2 system. Reaction conditions: 8 mmol L−1 Na10[α-SiW9O34]; 5 mmol L−1 BA; 20 mmol L−1 TH; 80 mmol L−1 HPPA; pH, 11.0; temperature, 25 °C; incubation time, 10 min. | |
Table 2 Comparison of different fluorescent systems for the detection of H2O2
System |
Linear rangea |
Detection limita |
Reference |
mol L−1. |
BA–BFO MNPs system |
2.0 × 10−8 to 2.0 × 10−5 |
4.5 × 10−9 |
13 |
BA–·OH |
1.1 × 10−5 to 1.1 × 10−3 |
1.0 × 10−6 |
44 |
CuO NPs/HPPA |
5.0 × 10−5 to 4.0 × 10−4 |
8.1 × 10−7 |
41 |
Hb–H2O2–thiamine |
1.0 × 10−7 to 8.0 × 10−5 |
2.6 × 10−8 |
40 |
HRP–Cy.7.Cl |
1.8 × 10−7 to 7.2 × 10−6 |
5.6 × 10−8 |
4 |
HBcAb–HRP–CdTe QDs |
1.0 × 10−7 to 1.5 × 10−4 |
6.9 × 10−8 |
45 |
CdTe–Na10[α-SiW9O34]–H2O2 |
7.8 × 10−9 to 2.5 × 10−7 |
3.8 × 10−9 |
39 |
BA–Na10[α-SiW9O34]–H2O2 |
1.0 × 10−8 to 1.6 × 10−6 |
6.7 × 10−9 |
This work |
TH–Na10[α-SiW9O34]–H2O2 |
1.6 × 10−6 to 1.0 × 10−4 |
2.2 × 10−7 |
This work |
HPPA–Na10[α-SiW9O34]–H2O2 |
1.0 × 10−5 to 2.5 × 10−4 |
9.6 × 10−6 |
This work |
3.5. Interference study
For further evaluating the detection selectivity of BA–Na10[α-SiW9O34], TH–Na10[α-SiW9O34] and HPPA–Na10[α-SiW9O34] systems for H2O2 determination, investigations were carried out at H2O2 concentrations of 5 × 10−7, 5 × 10−5 and 5 × 10−4 mol L−1 with various coexisting substrates added. The results for BA–Na10[α-SiW9O34]–H2O2 are shown in Table 3. And the results for TH–Na10[α-SiW9O34]–H2O2 and HPPA–Na10[α-SiW9O34]–H2O2 systems are shown in Tables S1 and S2.† It can be observed that none of the substances shows any obvious interference. Thus, these proposed fluorometric methods display a high selectivity for the determination of H2O2.
Table 3 The interference study for the determination of H2O2 (5 × 10−7 mol L−1) by the proposed method
Coexisting substance |
Content (mol L−1) |
ΔIa/I (%) |
ΔI = I0 − I, where I0 and I are the fluorescence intensities of the BA–Na10[α-SiW9O34]–H2O2 system in the absence and presence of interfering species. |
Na+ |
5 × 10−3 |
1.25 |
K+ |
5 × 10−3 |
−1.96 |
NH4+ |
5 × 10−3 |
−1.26 |
SO42− |
2 × 10−3 |
−2.80 |
Mn2+ |
2 × 10−3 |
2.90 |
Cl− |
2 × 10−3 |
2.38 |
CO32− |
2 × 10−3 |
−7.50 |
Glucose |
2 × 10−3 |
−2.47 |
Citric acid |
2 × 10−3 |
1.77 |
3.6. Determination of H2O2 in water samples
In order to evaluate the feasibility of the proposed methods, we tested the content of H2O2 in water samples under the optimum conditions. Each sample was analyzed at the same time with all the methods in order to avoid any possible differences caused by degradation of the analyte in the sample. The results of the BA–Na10[α-SiW9O34]–H2O2 system are presented in Table 4, where it can be seen that the data for recoveries was between 99.73% and 113.06%. The RSD of detection ranged from 2.57% to 4.66%. The results of the TH–Na10[α-SiW9O34]–H2O2 system are shown in Table S3.† For each sample, three parallel experiments were conducted, and the RSD of detection ranged from 0.82% to 4.06%. The recoveries of the samples were between 95.20% and 104.22%. The results for the HPPA–Na10[α-SiW9O34]–H2O2 system are listed in Table S4.† From Table S4,† the recoveries of H2O2 were found to range from 95.28% to 128.76%, and the RSD of detection ranges from 1.08% to 2.75%. The results demonstrated that these proposed methods for H2O2 determination were acceptable and suitable.
Table 4 Results of the analyses of H2O2 in water samples, when the substrate was BA
Sample |
Added (μM) |
Detected (μM) |
Recovery (%) |
RSD (n = 3, %) |
1 |
0.4 |
0.455 ± 0.0212 |
113.06 |
4.66 |
2 |
0.8 |
0.878 ± 0.0239 |
109.46 |
2.73 |
3 |
1.6 |
1.599 ± 0.0411 |
99.73 |
2.57 |
4. Conclusions
In summary, we established for the first time new fluorometric enhancement methods for the detection of hydrogen peroxide on the basis of the catalytic activation of Na10[α-SiW9O34] in alkaline H2O2 bleaching systems. H2O2 can be decomposed into ·OH radicals in the presence of Na10[α-SiW9O34], which could turn non-fluorescent substrates into a strongly fluorescent product. Under the optimal reaction conditions, linear correlation was established between fluorescence intensity and the concentration of H2O2. These methods will help in monitoring H2O2 doses and its related products, such as hydroxyl radicals, under complex practical conditions, but also in the further detection of the meaningful fluorogenic substrates, BA, TH and HPPA.
Conflicts of interest
There are no conflicts to declare.
Acknowledgements
This work was financially supported by the NSFC (81402719) and the Norman Bethune Program of Jilin University (2015228).
References
- Y. Tao, E. G. Ju, J. S. Ren and X. G. Qu, Chem. Commun., 2014, 50, 3030–3032 RSC.
- H. Q. Chen, H. P. Yu, Y. Y. Zhou and L. Wang, Spectrochim. Acta, Part A, 2007, 67, 683–686 CrossRef PubMed.
- X. S. Chai, Q. X. Hou, Q. Luo and J. Y. Zhu, Anal. Chim. Acta, 2004, 507, 281–284 CrossRef CAS.
- B. Tang, L. Zhang and K. H. Xu, Talanta, 2006, 68, 876–882 CrossRef CAS PubMed.
- B. Tang, L. Zhang and Y. Geng, Talanta, 2005, 65, 769–775 CrossRef CAS PubMed.
- G. C. Van de Bittner, E. A. Dubikovskaya, C. R. Bertozzi and C. J. Chang, Proc. Natl. Acad. Sci. U. S. A., 2010, 107, 21316–21321 CrossRef CAS PubMed.
- P. A. Tanner and A. Y. S. Wong, Anal. Chim. Acta, 1998, 370, 279–287 CrossRef CAS.
- B. Tang, Y. Wang, Y. Sun and H. X. Shen, Spectrochim. Acta, Part A, 2002, 58, 141–148 CrossRef.
- J. M. Lin and M. Yamada, Anal. Chem., 1999, 71, 1760–1766 CrossRef CAS PubMed.
- H. F. Yue, X. Bu, M. H. Huang, J. Young and T. Raglione, Int. J. Pharm., 2009, 375, 33–40 CrossRef CAS PubMed.
- L. S. Jahnke, Anal. Biochem., 1999, 269, 273–277 CrossRef CAS PubMed.
- K. Hensley, K. S. Williamson, M. L. Maidt, S. P. Gabbita, P. Grammas and R. A. Floyd, HRC J. High Resolut. Chromatogr., 1999, 22, 429–437 CrossRef CAS.
- W. Luo, Y. S. Li, J. Yuan, L. H. Zhu, Z. D. Liu, H. Q. Tang and S. S. Liu, Talanta, 2010, 81, 901–907 CrossRef CAS PubMed.
- X. Chen, D. Li, H. Yang, Q. Zhu, H. Zheng and J. Xu, Anal. Chim. Acta, 2001, 434, 51–58 CrossRef CAS.
- E. Kuah, S. Toh, J. Yee, Q. Ma and Z. Q. Gao, Chem.–Eur. J., 2016, 22, 8404–8430 CrossRef CAS PubMed.
- R. Breslow and L. E. Overman, J. Am. Chem. Soc., 1970, 92, 1075–1077 CrossRef CAS PubMed.
- Y. Aiba, J. Sumaoka and M. Komiyama, Chem. Soc. Rev., 2011, 40, 5657–5668 RSC.
- R. P. Bonarlaw and J. K. M. Sanders, J. Am. Chem. Soc., 1995, 117, 259–271 CrossRef CAS.
- G. P. Royer and I. M. Klotz, J. Am. Chem. Soc., 1969, 91, 5885–5886 CrossRef CAS.
- X. Zhang, H. P. Xu, Z. Y. Dong, Y. P. Wang, J. Q. Liu and J. C. Shen, J. Am. Chem. Soc., 2004, 126, 10556–10557 CrossRef CAS PubMed.
- D. J. Cram and J. M. Cram, Science, 1974, 183, 803–809 CrossRef CAS PubMed.
- J. M. Lehn and C. Sirlin, J. Chem. Soc., Chem. Commun., 1978, 949–951 RSC.
- Z. Y. Dong, Y. G. Wang, Y. Z. Yin and J. Q. Liu, Curr. Opin. Colloid Interface Sci., 2011, 16, 451–458 CrossRef CAS.
- G. Wulff and A. Sarhan, Angew. Chem., Int. Ed. Engl., 1972, 11, 341–342 CAS.
- T. Takagish and I. M. Klotz, Biopolymers, 1972, 11, 483–491 CrossRef PubMed.
- T. Pan and O. C. Uhlenbeck, Biochemistry, 1992, 31, 3887–3895 CrossRef CAS PubMed.
- R. R. Breaker and G. F. Joyce, Chem. Biol., 1994, 1, 223–229 CrossRef CAS PubMed.
- S. J. Pollack, J. W. Jacobs and P. G. Schultz, Science, 1986, 234, 1570–1573 CrossRef CAS PubMed.
- D. L. Long, R. Tsunashima and L. Cronin, Angew. Chem., Int. Ed., 2010, 49, 1736–1758 CrossRef CAS PubMed.
- M. T. Pope and A. Müller, Angew. Chem., Int. Ed., 1991, 30, 34–48 CrossRef.
- J. J. Wang, X. G. Mi, H. Y. Guan, X. H. Wang and Y. Wu, Chem. Commun., 2011, 47, 2940–2942 RSC.
- S. Liu, J. Q. Tian, L. Wang, Y. W. Zhang, Y. L. Luo, H. Y. Li, A. M. Asiri, A. O. Al-Youbi and X. P. Sun, Chempluschem, 2012, 77, 541–544 CrossRef CAS.
- J. J. Wang, D. X. Han, X. H. Wang, B. Qi and M. S. Zhao, Biosens. Bioelectron., 2012, 36, 18–21 CrossRef CAS PubMed.
- D. Li, H. Y. Han, Y. H. Wang, X. Wang, Y. G. Li and E. B. Wang, Eur. J. Inorg. Chem., 2013, 1926–1934 CrossRef CAS.
- C. L. Sun, X. L. Chen, J. Xu, M. J. Wei, J. J. Wang, X. G. Mi, X. H. Wang, Y. Wu and Y. Liu, J. Mater. Chem. A., 2013, 1, 4699–4705 RSC.
- Z. Sun, H. Z. Bie, M. J. Wei, J. J. Wang, X. G. Mi, X. H. Wang and Y. Wu, Chin. Chem. Lett., 2013, 24, 76–78 CrossRef CAS.
- Y. Ji, J. Xu, X. L. Chen, L. Han, X. H. Wang, F. Chai and M. S. Zhao, Sens. Actuators, B, 2015, 208, 497–504 CrossRef CAS.
- Z. Ma, Y. F. Qiu, H. H. Yang, Y. M. Huang, J. J. Liu, Y. Lu, C. Zhang and P. A. Hu, ACS Appl. Mater. Interfaces, 2015, 7, 22036–22045 CrossRef CAS PubMed.
- R. Tian, B. Y. Zhang, M. M. Zhao, Q. Ma and Y. F. Qi, Talanta, 2018, 188, 332–338 CrossRef CAS PubMed.
- C. L. Xu and Z. J. Zhang, Anal. Sci., 2001, 17, 1449–1451 CrossRef CAS PubMed.
- H. H. Deng, X. Q. Zheng, Y. Y. Wu, X. Q. Shi, X. L. Lin, X. H. Xia, H. P. Peng, W. Chen and G. L. Hong, Analyst, 2017, 142, 3986–3992 RSC.
- A. Téazéa, G. Hervéa, R. G. Finke and D. K. Lyon, Inorg. Synth., 1990, 27, 85–96 Search PubMed.
- K. Zaitsu and Y. Ohkura, Anal. Biochem., 1980, 109, 109–113 CrossRef CAS PubMed.
- F. Si, X. Zhang and K. L. Yan, RSC Adv., 2014, 4, 5860–5866 RSC.
- T. T. Gong, J. F. Liu, Y. W. Wu, Y. Xiao, X. H. Wang and S. Q. Yuan, Biosens. Bioelectron., 2017, 92, 16–20 CrossRef CAS PubMed.
Footnote |
† Electronic supplementary information (ESI) available. See DOI: 10.1039/c9ra00505f |
|
This journal is © The Royal Society of Chemistry 2019 |