DOI:
10.1039/C9RA00116F
(Paper)
RSC Adv., 2019,
9, 7723-7728
Improving gas sensing performance by oxygen vacancies in sub-stoichiometric WO3−x
Received
6th January 2019
, Accepted 23rd February 2019
First published on 7th March 2019
Abstract
Sub-stoichiometric WO3−x has provided an alternative platform to investigate oxygen vacancies in gas sensors based on metal-oxides. We present an experimental study on the influence of sub-stoichiometric WO3−x phase upon gas sensing performance. High-quality WO3−x nanostructures with several x values (WO3, W19O55, W5O14, W18O49) were synthesized and used to fabricate H2S gas sensors. Temperature programmed desorption of oxygen (O2-TPD) shows that oxygen absorption behaviors of the as-prepared WO3−x nanostructures are affected by oxygen vacancies, which played a critical role in the detection of H2S at varying temperature range. We find that oxygen vacancies in sub-stoichiometric WO3−x facilitate the ionosorption process and in turn enhance the performance of the gas sensor.
Introduction
According to the “ionosorption model”, ionosorbed oxygen species (i.e. O2−, O−, O2−) on semiconductor surfaces determine the gas sensing behavior.1–3 So far, considerable efforts have been applied to facilitate the oxygen ionosorption for enhancing the gas sensing performance and various strategies are adopted to manipulate oxygen absorption, such as changing morphologies,4–6 changing sizes and dimensions of micro or nano materials,7–9 and using catalysts10,11 or UV-light irradiation.12,13 Recently, oxygen vacancies have been found to play a critical role in influencing the gas sensing process and determining the performance of gas sensors. For example, density functional theory (DFT) has been successfully employed to study the effect of oxygen deficiency on the electronic and structural properties of sub-stoichiometric tungsten oxides (WO3−x).14–17 F. Wang, et al., reported the computational results that revealed the strong dependence of WO3−x electronic properties on oxygen vacancies.15 J. Song, et al., simulated the kinetic and thermodynamic processes of H2 adsorption, activation, and dissociation on WO2.72 surface.16 High sensitive NO2 gas sensors based on W18O49 nanowires have been fabricated and the improvement of the sensing performance are due to oxygen vacancies in W18O49.18 Monoclinic W18O49 is a well-studied sub-stoichiometric phase and can easily be isolated in a pure form.19–21 In fact, sub-stoichiometric WO3−x materials have provided a powerful platform to investigate the gas sensing behaviour associated with oxygen vacancies in metal-oxides based gas sensors. However, it is a challenging task to control oxygen vacancies in sub-stoichiometric WO3−x accurately.
In this work, we synthesised several sub-stoichiometric WO3−x, including W19O55, W5O14 and W18O49, and fabricated gas sensors using the materials. We systematically studied the role of oxygen vacancies in sensing properties of the gas sensors. It was found that oxygen vacancies affected oxygen absorption on the surface of the WO3−x nanostructures, and facilitated the ionosorption process, favourable for improving device performance and lowering operating temperature. Manipulation of oxygen vacancies in metal oxides brings us an alternative way to improve the performance of gas sensing devices.
Experimental
Synthesis of tungsten oxide samples
WO3 powder was synthesized in a typical hydrothermal method as we had reported before.22 The sub-stoichiometric tungsten oxides (e.g. W19O55, W5O14, W18O49) were obtained by the reduction of WO3 powders with S powders. The chemical compositions in the sub-stoichiometric tungsten oxides were controlled by the growth temperature. In the growth system, the as-prepared WO3 powder was located in the middle of a vacuum tube furnace, while S powder was deposited at its upstream position. After evacuating pre-treatment and repeating flush with pure Ar gas, the tube furnace was heated up to the target temperature and dwelled for one hour.23 The raw WO3 powders were then annealed at 500 °C in atmospheric environment. W19O55 (dark-blue), W5O14 (purple-blue), and W18O49 (purple-red) were synthesized at 950, 1050, 1150 °C in the vacuum tube furnace, respectively. The corresponding colours of the resulting sub-stoichiometric tungsten oxides depend on their chemical compositions, as reported in literatures.19,24–26
Sample characterization and gas sensors measurements
The morphologies of the obtained sub-stoichiometric nanomaterials were examined by a field emission scanning electron microscope (SEM, FEI Sirion 200). Transmission electron microscopy (TEM) and high-resolution transmission electron microscopy (HRTEM, JEOL2100F transmission electron microscope) was adopted to study the crystallographic structures. The phase and composition were investigated by X-ray diffractometry (XRD, Bruker D8 Avance) with a Cu Kα radiation source (40 kV and 40 mA). X-ray photoelectron spectrometer (XPS, ESCALAB 250Xi, Thermo Scientific) was employed to identify the elements and chemical states by using sub-monochromatized Al KR X-ray as the excitation source. The spectrometer was calibrated with the binding energy of the C 1s line (284.5 eV). Gas sensing test was performed on the HW-30A system (Hanwei Electronics Co. Ltd., PR China).
Results and discussions
Morphology, structure and composition characterization
The XRD results of the as-prepared WO3, W19O55, W5O14, and W18O49 are present in Fig. 1. Fig. 2 illustrates the SEM and HRTEM images of the samples. Grown at different temperatures, the pristine WO3 crystal exhibits irregular rod-like shape as shown in Fig. 2(a) and the W19O55, W5O14, and W18O49 samples show the similar micro and nano rod shapes with flat and smooth surface. The crystal nucleus grows promptly along the preferential growth direction, leading to such symmetric structures. The sub-stoichiometric W19O55, W5O14, and W18O49 are well crystalline with clear grain boundaries and ordered crystal planes as shown in Fig. 2(b–d). The diffraction curve recorded in Fig. 1(a) (black line) can be well indexed to the phase of monoclinic WO3 (JCPDS no. 43-1035), with three main diffraction peaks located at 2θ = 23.12, 23.59, and 24.38°, corresponding to the preferential growth directions of [002], [020], [200], respectively. The (002) plane with d-spacing of 3.84 Å is further demonstrated by the HRTEM image in Fig. 2(e).27 The blue curve in Fig. 1(b) matches well with W19O55 (JCPDS no. 45-0167) and the strongest diffraction peak located at 2θ = 23.47° corresponds to the (010) facet with an interlayer distance of 3.7 Å as shown in Fig. 2(f).28 The diffraction peaks of the red curve in Fig. 1(c) are in good agreement with JCPDS no. 71-0292 file for W5O14. The main peak located at 2θ = 23.41° suggests the (001) planes with interlayer distance of 3.797 Å, which is a close match to the HRTEM result of 3.79 Å in Fig. 2(g). The W5O14 sample grown in a longitudinal direction along the (001) crystallographic axis has the high aspect ratio of nanorods as shown in the SEM image.24 At last, Fig. 1(d) depicts the XRD curve of monoclinic W18O49 (JCPDS no. 05-0392) with the main peak located at 23.45° corresponding to (010) planes, and the interlayer distance of 3.8 Å is consistent with the HRTEM value, 3.76 Å, as depicted in Fig. 2(h).18,19,29
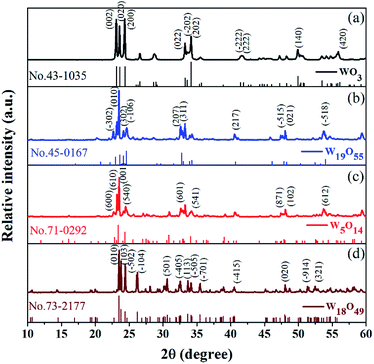 |
| Fig. 1 XRD curves of the as-prepared WO3 and sub-stoichiometric WO3−x, with standard JCPDS card marked below the corresponding curves. | |
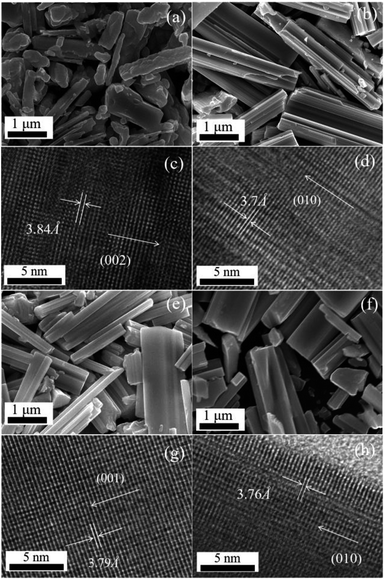 |
| Fig. 2 SEM morphologies and HRTEM images of WO3 (a and c), W19O55 (b and d), W5O14 (e and g), W18O49 (f and h). | |
X-ray photoelectron spectroscopy (XPS) provides an appropriate solution to investigate the chemical composition and element states. As we know, tungsten has a complex distribution of W 4f binding energies consisted of three pairs of doublets (six peaks). As exhibited in Fig. 3(a–d), the thin black line is raw data and the red one is the result of smooth fitting. The first doublet peaks (blue line) locate at 37.85 eV and 35.75 eV corresponding to W 4f5/2 and W 4f7/2, which confirms that the W ions are dominated by W6+ in WO3. No W5+ and W4+ peaks are observed in Fig. 3(a). However, on the other three samples synthesized at 950, 1050, 1150 °C, we observe the mixture of W6+, W5+ and W4+ oxidation states. As revealed in Fig. 3(b), the second doublet peaks (green dot-curve) appearing at 36.85 and 33.85 eV are attributed to the W5+ state. The third doublet peaks occur at 35.25 and 32.5 eV (see the navy dot line), implying the presence of the W4+ oxidation state.26 Thus, as shown by Fig. 3, the contents of W5+ and W4+ tend to increase gradually with increasing growth temperature. Moreover, we have concluded the O 1s-level XPS spectra of the four WO3−x samples in Fig. 3(e–h), the thin black line is raw data and the red one is the sum of four deconvolution spectra. The first peak (blue line) locate at 530.9 eV corresponding to oxygen atoms O2− in the lattice, and the second peak (green line) locate at 532 eV corresponding to adsorbed oxygen species and oxygen state O1−.30,31 Metal oxide surfaces that are exposed to the atmosphere are always hydrated, so that hydroxyl groups (–OH) and water molecules (H2O) signals are also found at 531.4, 532.8 eV, respectively.31 Our XPS results are in perfect agreement with the report by A. P. Shpak in 2007.31
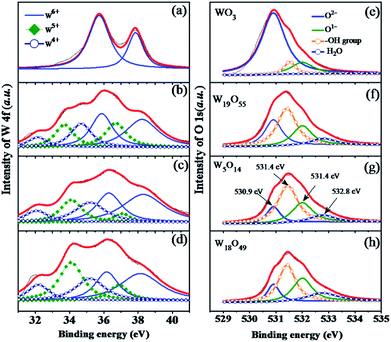 |
| Fig. 3 (a–d) The W 4f-level and (e–h) O 1s-level XPS deconvolution spectra of the as-prepared sub-stoichiometric tungsten oxides, WO3, W19O55, W5O14, and W18O49. | |
As we can see in Fig. 3(e), oxygen states of O2− are dominated in WO3 sample, the content of O1− or remnants of adsorbed oxygen species are much smaller than that of O2− state. However, the intensity ratio of O2−/O1− is increasing continuously from WO3 to W18O49, which further confirms the enhanced oxygen adsorption performance in the sub-stoichiometric tungsten oxide. This is the main reason why sensing performance is improved in WO3−x samples. However, the adsorption of H2O molecules in atmosphere and the formation of –OH groups are also enhanced in the non-stoichiometric WO3−x, which is supposed to suppress the sensing process. This explains why our sensing response of WO3−x samples are improved but with a limited improvement.
From the Raman curves in Fig. 4, Raman characteristic peaks of WO3 are very clearly exhibited at 806 cm−1, 716 cm−1, 326 cm−1 and 272 cm−1.28,31 However, much wider peaks are observed at 300 and 800 cm−1 on the sub-stoichiometric tungsten oxide samples. Due to the distortions in the crystalline structure, it is difficult to assign each of the bands to certain vibrations, particularly for the bands below 300 cm−1 which might be deconvoluted into several deformation and lattice vibrations. As exhibited in Fig. 4(b–d), the main bands of W19O55, W5O14, and W18O49 are located at 816 cm−1, 805 cm−1 and 801 cm−1, respectively. The main band shifts to lower wave number (lower energy) with increasing x value, indicating that more content of reduced tungsten state (W4+, W5+) were formed in the sub-stoichiometric phases.28,29,32
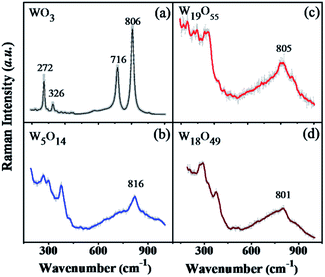 |
| Fig. 4 The Raman spectra of the prepared sub-stoichiometric tungsten oxides, WO3 (a), W19O55 (b), W5O14 (c), and W18O49 (d). | |
Gas sensing properties and discussion
The as-grown sub-stoichiometric tungsten oxides have stable chemical properties and have become an alternative platform to investigate the behaviour of oxygen vacancies in metal-oxides based gas sensors.23 Fig. 5 shows temperature-dependent sensitivity curves of WO3 (a), W19O55 (b), W5O14 (c), and W18O49 (d) when the sensors are exposed to different concentrations of H2S (1, 2, 3, 4, 10, 50 ppm), marked in different shades of colours. Firstly, it is found that the low-temperature performance of the sub-stoichiometric WO3−x sensors has a tendency to improve as the x value increases. The WO3 and W19O55 sensors show high response at temperature above 380 °C, while the W5O14 and W18O49 sensors hold the best performance approximately at 280 °C. The W18O49 sensor presents a broad band sensitivity curve with a broad shoulder extending to the low temperature side ranging from 150 to 250 °C approximately. W18O49 has the highest density of oxygen vacancies among the tested sensors, suggesting that low-temperature sensing performance could be improved as a result of increasing concentration of oxygen vacancies. Thus, oxygen deficiency facilitates chemisorption of oxygen molecules on the WO3−x surface. The W5O14 sensor has a narrow temperature-dependent response curve with its highest response at around 280 °C. As a general trend, it is found that increasing oxygen vacancies give rise to enhanced device performance at low temperature as shown in Fig. 5(a–d), which is beneficial for applications.
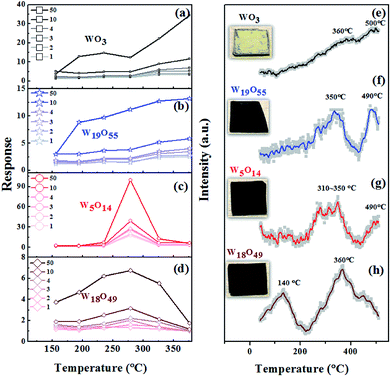 |
| Fig. 5 (a–d) Temperature-dependent response curves of the gas sensors based on WO3, sub-stoichiometric W19O55, W5O14, and W18O49 when exposed to different concentrations of H2S (1, 2, 3, 4, 10, 50 ppm, marked in different shades of colour). (e–h) The O2-TPD profiles for the four sensors and their corresponding photographs presented next to the curves. | |
To understand the oxygen absorption behaviours of the sub-stoichiometric tungsten oxide sensors, Fig. 5(e–h) display the temperature programmed desorption (O2-TPD) profiles of the WO3−x sensors, together with their photographs presented next to their corresponding curves. As the temperature increases, ambient oxygen molecules that are adsorbed on the tungsten-oxide surfaces undergo changes following the sequence of O2 → O2−→ O−→ O2−.2 Physically adsorbed oxygen molecules (O2) make ignorable contribution to gas sensing. The peaks of chemisorbed oxygen species (O2−, O−, O2−) are reported to centre around 400, 600 and >700 °C on the TPD curves.33 Thus, Fig. 5(e) shows that the pristine WO3 exhibits a broad desorption bands around 360 and 500 °C, attributed to the chemisorption of oxygen species O2− and O−. For the W19O55 sensor, the two peaks are shifted to lower temperature positions at ∼350 and ∼490, as shown in Fig. 5(f). In the case of W5O14, strong peaks are observed at 310–350 °C (see Fig. 5(g)), while the peak at ∼490 °C is rather weak. This indicates that a large amount of oxygen ions are chemisorbed in 310–350 °C, which might explain why there is a relatively strong response peak at ∼280 °C, close to 310–350 °C where there are a lot of chemisorbed oxygen ions. As x increases to 0.28 (W18O49), a strong peak occurs at the low temperature of 140 °C shown by Fig. 5(h). The peak at 350 or 360 °C remains essentially unchanged. These results indicate that oxygen deficiency enhances chemisorption of oxygen ions on WO3−x surface at low temperature by offering a large number of chemically active absorption sites, which in turn facilitates the ionosorption process and improves the low-temperature gas sensing sensitivity.34–36 Thus, both the temperature-dependent response curves and the O2-TPD profiles measured on the sub-stoichiometric WO3−x sensors evidence that the working temperature of the gas sensors can be lowered down by using sub-stoichiometric nano WO3−x.
The W5O14 sensor presents the best performance over the narrow temperature range around 280 °C. However, W18O49 possesses the highest concentration of oxygen vacancies among all the sub-stoichiometric WO3−x ones studied here, and the W18O49 sensor presents considerable response at low temperature. Obviously, the x value and the sensing sensitivity of the WO3−x sensor do not have a simple relationship. Some other factors might affect device performance, such as specific surface area, crystallographic planes on the surface, and density of interfacial states, according to the published works.18,37 Qin's work suggests that agglomeration of W18O49 at a comparatively high operating temperature weakens the gas diffusion, which in turn gives rise to low response.34 Overall speaking, W18O49 is a metal-like material with abnormally high carrier concentration.19,38 It is expected that the carrier concentration in W18O49 is comparable to the change of electron population induced by the target gas molecules. This leads to a relatively small resistance change when the sensor is exposed to the target gas, compared to the result measured on the W5O14 sensor. The W18O49 sensor, with the highest concentration of oxygen vacancies, thus possesses relatively low sensing response compared to the W5O14 sensor, though its low-temperature performance is quite good.
The response and recovery times of the sensors are also investigated. Fig. 6(a–d) presents the dynamic response curves of the WO3, W19O55, W5O14, and W18O49 sensors measured by the HW-30A gas sensing test system at given temperatures. The measured response (recovery) times are 67 s (65 s), 53 s (37 s), 21 s (11 s), and 32 s (15 s) for the WO3, W19O55, W5O14, and W18O49 sensors, respectively. This overall results show the tendency that the use of sub-stoichiometric tungsten oxides accelerates response–recovery processes.
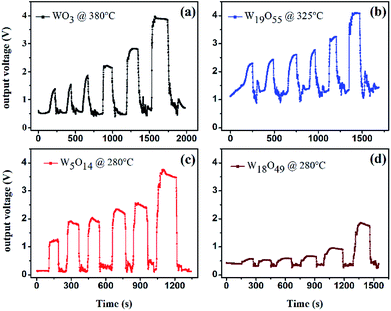 |
| Fig. 6 Dynamic response curves of the sub-stoichiometric WO3−x sensors: (a) WO3 at 380 °C, (b) W19O55 at 325 °C, (c) W5O14 and (d) W18O49 at 280 °C after exposed to 1, 2, 3, 4, 10, 50 ppm H2S. | |
Conclusions
We have demonstrated that oxygen deficiencies facilitate the low-temperature oxygen ionosorption process, leading to the improvement of sensing performance of the WO3−x-based gas sensors. High-quality sub-stoichiometric WO3−x with several x values (WO3, W19O55, W5O14, and W18O49) was synthesized and H2S gas sensors were fabricated using the materials. The W18O49 sensor shows good device performance in a wide temperature range, particularly at the temperature as low as 150 °C. The W5O14 gas sensor presents very high response value in a narrow temperature range centred at 280 °C. Our work shows that the sub-stoichiometric WO3−x can be used to enhance the low temperature sensing performance through controlling the concentration of oxygen vacancies.
Conflicts of interest
There are no conflicts to declare.
Acknowledgements
This work is financially supported by National Key R&D Program of China (2016YFA0202200), National Natural Science Foundation of China (Grant no. 11574335, 61471345, 51772213), the Frontier Science Research Project (Key Programs) of Chinese Academy of Sciences (Grant no. QYZDJ-SSW-SLH018).
References
- A. Gurlo and R. Riedel, Angew. Chem., Int. Ed., 2007, 46, 3826–3848 CrossRef CAS PubMed.
- A. Gurlo, ChemPhysChem, 2006, 7, 2041–2052 CrossRef CAS PubMed.
- N. H. Al-Hardan, M. J. Abdullah, A. Abdul Aziz, H. Ahmad and L. Y. Low, Vacuum, 2010, 85, 101–106 CrossRef CAS.
- S. W. Choi, J. Y. Park and S. S. Kim, Nanotechnology, 2009, 20, 465603 CrossRef PubMed.
- X. Song, Z. Wang, Y. Liu, C. Wang and L. Li, Nanotechnology, 2009, 20, 075501 CrossRef PubMed.
- H. G. Moon, Y. S. Shim, D. H. Kim, H. Y. Jeong, M. Jeong, J. Y. Jung, S. M. Han, J. K. Kim, J. S. Kim, H. H. Park, J. H. Lee, H. L. Tuller, S. J. Yoon and H. W. Jang, Sci. Rep., 2012, 2, 588 CrossRef PubMed.
- D. Zhang, Z. Liu, C. Li, T. Tang, X. Liu, S. Han, B. Lei and C. Zhou, Nano Lett., 2004, 4, 1919–1924 CrossRef CAS.
- Y. Zhou, G. Xie, T. Xie, H. Yuan, H. Tai, Y. Jiang and Z. Chen, Appl. Phys. Lett., 2014, 105, 033502 CrossRef.
- J. D. Fowler, M. J. Allen, V. C. Tung, Y. Yang, R. B. Kaner and B. H. Weiller, ACS Nano, 2009, 3, 301–306 CrossRef CAS PubMed.
- L. Xu, R. Xing, J. Song, W. Xu and H. Song, J. Mater. Chem. C, 2013, 1, 2174 RSC.
- Z. Meng, H. Jian Xing and O. Chung Wo, Nanotechnology, 2012, 23, 315503 CrossRef PubMed.
- D. Jung, M. Han and G. S. Lee, ACS Appl. Mater. Interfaces, 2015, 7, 3050–3057 CrossRef CAS PubMed.
- D. Jung, M. Han and G. S. Lee, Sens. Actuators, A, 2014, 211, 51–54 CrossRef CAS.
- D. B. Migas, V. L. Shaposhnikov and V. E. Borisenko, J. Appl. Phys., 2010, 108, 093714 CrossRef.
- F. Wang, C. Di Valentin and G. Pacchioni, Phys. Rev. B, 2011, 84, 073103 CrossRef.
- J. Song, Z.-F. Huang, L. Pan, J.-J. Zou, X. Zhang and L. Wang, ACS Catal., 2015, 5, 6594–6599 CrossRef CAS.
- Y. Qin, D. Hua and M. Liu, J. Alloys Compd., 2014, 587, 227–233 CrossRef CAS.
- Y. Qin, W. Xie, Y. Liu and Z. Ye, Sens. Actuators, B, 2016, 223, 487–495 CrossRef CAS.
- S. Cong, F. Geng and Z. Zhao, Adv. Mater., 2016, 28, 10518–10528 CrossRef CAS PubMed.
- G. Xi, S. Ouyang, P. Li, J. Ye, Q. Ma, N. Su, H. Bai and C. Wang, Angew. Chem., 2012, 124, 2445–2449 Search PubMed.
- Y. Li, Y. Bando and D. Golberg, Adv. Mater., 2003, 15, 1294–1296 Search PubMed.
- W. Yu, Y. Sun, T. Zhang, K. Zhang, S. Wang, X. Chen and N. Dai, Part. Part. Syst. Charact., 2016, 33, 15–20 CrossRef CAS.
- J. Qian, Z. Peng, Z. Shen, Z. Zhao, G. Zhang and X. Fu, Sci. Rep., 2016, 6, 25574 CrossRef CAS PubMed.
- M. Remškar, J. Kovac, M. Viršek, M. Mrak, A. Jesih and A. Seabaugh, Adv. Funct. Mater., 2007, 17, 1974–1978 CrossRef.
- Z. Shen, Z. Zhao, J. Wen, J. Qian, Z. Peng and X. Fu, J. Nanomater., 2018, 2018, 1–12 CrossRef.
- Z. Shen, Z. Zhao, J. Qian, Z. Peng and X. Fu, J. Mater. Res., 2016, 31, 1065–1076 CrossRef CAS.
- C.-Y. Su, H.-C. Lin, T.-K. Yang, C.-H. Chang and C.-K. Lin, Mater. Trans., 2009, 50, 2593–2597 CrossRef CAS.
- J. Qian, Z. Zhao, Z. Shen, G. Zhang, Z. Peng and X. Fu, RSC Adv., 2016, 6, 8061–8069 RSC.
- K. Thummavichai, L. Trimby, N. Wang, C. D. Wright, Y. Xia and Y. Zhu, J. Phys. Chem. C, 2017, 121, 20498–20506 CrossRef CAS.
- J. C. Dupin, D. Gonbeau, P. Vinatier and A. Levasseur, Phys. Chem. Chem. Phys., 2000, 2(6), 1319–1324 RSC.
- A. Shpak, M. Korduban, M. M. Medvedskij and V. O. Kandyba, J. Electron Spectrosc. Relat. Phenom., 2007, 156–158, 172–175 CrossRef CAS.
- C. Jian, L. Dongyu, Z. Weihong, X. Fangyan, Z. Jun, G. Li, L. Xiao, D. Shaozhi and X. Ningsheng, J. Phys. D: Appl. Phys., 2008, 41, 115305 CrossRef.
- X. Huang, Y. Peng, X. Liu, K. Li, Y. Deng and J. Li, Catal. Commun., 2015, 69, 161–164 CrossRef CAS.
- Y. Qin, M. Hu and J. Zhang, Sens. Actuators, B, 2010, 150, 339–345 CrossRef CAS.
- J. Liu, Y. Gao, X. Wu, G. Jin, Z. Zhai and H. Liu, Sensors, 2017, 17, 1852 CrossRef PubMed.
- J. Wu, Q. Huang, D. Zeng, S. Zhang, L. Yang, D. Xia, Z. Xiong and C. Xie, Sens. Actuators, B, 2014, 198, 62–69 CrossRef CAS.
- C. T. Pan, C. Y. Su and Y. C. Luo, Microsyst. Technol., 2016, 23, 2113–2123 CrossRef.
- E. Salje and B. Güttler, Philos. Mag. B, 1984, 50, 607–620 CAS.
|
This journal is © The Royal Society of Chemistry 2019 |
Click here to see how this site uses Cookies. View our privacy policy here.