DOI:
10.1039/C8RA10470K
(Paper)
RSC Adv., 2019,
9, 6021-6026
A novel diarylethene-based fluorescent “turn-on” sensor for the selective detection of Mg2+†
Received
21st December 2018
, Accepted 13th February 2019
First published on 19th February 2019
Abstract
A new photochromic diarylethene derivative with a 4-methylphenol unit has been designed and synthesized. It displayed distinct photochromism and fluorescent ‘‘turn on’’ features to Mg2+ in acetonitrile solution. With the addition of Mg2+, there was an obvious increase of fluorescent emission intensity at 552 nm, accompanied by a clear change of fluorescent color from dark purple to green. Meantime, the 1
:
1 stoichiometry between the derivative and Mg2+ was verified by Job's plot and HRMS. Furthermore, the sensor was successfully applied in the detection of Mg2+ in practical samples. Moreover, based on the multiple-responsive fluorescence switching behaviors, it also could be used to construct a molecular logic circuit with UV/vis lights and Mg2+/EDTA as input signals and the emission at 552 nm as the output signal.
Introduction
Magnesium, as the fourth most plentiful cation in the human body, has been considered as an important biological ion for all living things in the past century.1–8 Meanwhile, it plays significant physiological roles in numerous cellular processes, such as enzymatic catalytic reactions, membrane protein activities including ion channels, and the regulation of Ca2+ signal.9–13 Moreover, magnesium plays a positive role in bone reconstruction and skeletal development.14–16 Abnormal levels of magnesium in serum or cells have been associated with a variety of diseases, including cardiovascular disease, diabetes, neurodegeneration and cancer.17–20 For instance, chronic magnesium deficiency may cause a lot of chronic diseases, such as diabetes, osteoporosis, hypertension and coronary heart disease.21,22 On the contrary, high levels of Mg2+ can lead to many age-related and neuronal diseases ranging from high blood pressure to Alzheimer's disease.23–25 In this regard, developing efficient and sensitive analytical methods for Mg2+ has attracted more and more interest in the biological sciences and chemistry.
In tradition, there are some analytical methods such as atomic absorption spectrum, ion-selective electrodes (ISES), and NMR for the detection of Mg2+.26–29 However, all of these methods need complex apparatus and high cost. So, the convenient and inexpensive method for the detection of Mg2+ in different environments is desired. Compared to these methods, fluorescence analysis is a very reliable and effective method to detect metal ions because of their high sensitivity, good selectivity, high reaction speed, and simple operation.30–32 Up to now, various fluorescence sensors for Mg2+ have been developed. These sensors have different receptor groups based on moieties including diaza-18-crown-6,33 benzo-15-crown-5,34 calix[4]arene,35 benzo-chromene,36 imidazo-1,10-phenanthroline,37 and other functional units.38–42 However, most of the reported Mg2+ sensors have poor selectivity to Mg2+ and Ca2+, due to their similar chemical properties, especially when the concentration of Ca2+ is much higher than that of Mg2+. Hence, it is vital to design highly selective, sensitive, and simple sensors that can recognize Mg2+ without the interference of Ca2+.43,44
Among the reported fluorescence sensors,45–54 diarylethene derivatives are the most promising candidates owing to their excellent thermal stability, remarkable fatigue resistance, and rapid response time.55–57 Furthermore, the recognized ions could induce a stable transformation between diarylethene molecule and complex, and these properties make it possible for the application in logic gates.58 Although much work in diarylethenes based on ion recognition has been finished,59–65 the sensors for the selective detection of Mg2+ based on diarylethenes are rarely reported.66
Herein, a new diarylethene-based fluorescent sensor (1o) for Mg2+ was developed. By investigating the fluorescent spectrum and color changes of this sensor under the stimulation of chemical substance and lights in acetonitrile solution, it could be found that the fluorescence of 1o could be effectively regulated by Mg2+ and lights. Moreover, a systematic discussion of its photochromic and fluorescent properties was also recorded in the full text in detail.
Experimental
General methods
All the reagents used in the synthesis process were from a variety of commercial sources without further purification. All cations were added in the form of their corresponding metal nitrates except for Sn2+ and Hg2+ (their counter anion ions were chloride ions). Metal ion solutions (0.1 mol L−1) were prepared by dissolving their respective metal salts in deionized water while performing the necessary dilutions according to each experimental device. Nuclear magnetic resonance hydrogen and carbon spectra were obtained with a Bruker AV400 spectrometer by using acetonitrile-d3 as the solvents and tetramethylsilane (TMS) as an internal standard. High resolution mass spectrometry was collected on a Bruker Amazon SL ion trap mass spectrometer (ESI). The melting point was determined by a WRS-1B melting point instrument. UV-vis absorption spectra were recorded with an Agilent 8453 UV/vis spectrometer. Fluorescence spectra were measured by using a Hitachi F-4600 fluorescence spectrophotometer with the slit width of 5.0 nm for both excitation and emission. Photoirradiation experiments were performed with an SHG-200 UV lamp and BMH-250 visible lamp. Absolute fluorescence quantum yields were measured using an Absolute PL Quantum Yield Spectrometer QYC11347-11. Infrared spectra were collected on a Bruker Vertex-70 spectrometer. The pH was measured with Ferromagnetic PHS-3C pH meter. All of experiments were conducted at room temperature without special instructions.
Synthesis of compound 1o
Compound 2 was synthesized according to the method reported in the previous work.67 Then compound 2 (0.094 g, 0.2 mmol) was dissolved in 5.0 mL absolute methanol, followed by the addition of 2-amino-4-methylphenol (0.025 g, 0.2 mmol). After the mixture was stirred at room temperature for 6.0 hours, the reaction system was placed in a freezer for overnight freezing (Scheme 1). It can be seen that a yellow solid precipitated and was washed three times with anhydrous methanol (5.0 mL × 3) and dried to get the target compound 1o (0.096 g, yield: 83%). Mp: 435–437 K. 1H NMR (400 MHz, acetonitrile-d3, TMS), δ (ppm): 2.02 (s, 3H), 2.12 (s, 3H), 2.24 (s, 3H), 2.29 (s, 3H), 6.84 (d, J = 8.4 Hz, 1H), 7.04 (d, J = 8.6 Hz, 1H), 7.32 (s, 2H), 7.58 (s, 1H), 8.08 (d, J = 8.6 Hz, 1H), 8.40 (d, J = 8.4 Hz, 1H), 8.79 (s, 1H), 8.94 (s, 1H) (Fig. S1†). 13C NMR (100 MHz, acetonitrile-d3, TMS), δ (ppm): 10.7, 12.2, 14.6, 20.3, 105.0, 115.2, 115.9, 122.4, 125.5, 125.8, 130.2, 130.9, 133.9, 135.6, 139.3, 144.6, 147.1, 147.6, 151.2, 154.6, 157.9, 159.1, 171.1 (Fig. S2†). HRMS: m/z = 578.1313 [M + H+]+. Calcd 578.1337 (Fig. S3†).
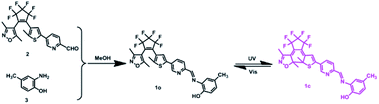 |
| Scheme 1 The synthetic route and photochromism of 1o. | |
Results and discussion
Photochromism of 1o
Fig. 1 shows the photochromic properties of 1o by UV/vis light studied in acetonitrile (2.0 × 10−5 mol L−1) at room temperature. Two absorption bands of compound 1o at 338 nm (ε = 2.94 × 104 mol−1 L cm−1) and 381 nm (ε = 2.67 × 104 mol−1 L cm−1) were observed, respectively. When irradiated with 297 nm light, the ring-closed isomer 1c was regenerated and a new absorption band centered at 536 nm (ε = 1.93 × 103 mol−1 L cm−1) appeared, owing to a larger π-electron delocalization formed in the molecule.68 When the photostationary state (PSS) was reached, an isosbestic point was observed at 407 nm, indicating a reversible two-component photochromic reaction.69 At the same time, there was a visible change of the color from colorless to pink. On the contrary, when irradiated with visible light (λ > 500 nm), the absorption spectrum and the color of the solution could be quickly recovered to that of 1o. The cyclization and cycloreversion quantum yields of 1o were determined to be 0.023 and 0.004, respectively, with 1,2-bis(2-methyl-5-phenyl-3-thienyl)perfluorocyclopentene as a reference70 On the other hand, the fatigue resistance of 1o was measured in acetonitrile by alternative irradiation of UV/vis lights at room temperature. The results showed that 10 times coloration–decoloration cycles between 1o and 1c could cause 20% degradation (Fig. S4†).
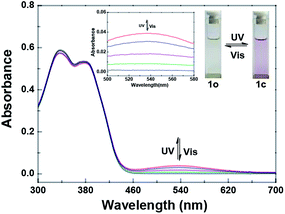 |
| Fig. 1 Changes in the absorption spectra of 1o upon irradiation with UV/vis lights in acetonitrile (2.0 × 10−5 mol L−1). | |
Selectivity of 1o to metal ions
The experiments of fluorescence selectivity of 1o toward various metal ions (5.0 equiv. of 1o) such as Al3+, Cu2+, Sn2+, Ca2+, K+, Ag+, Ni2+, Ba2+, Zn2+, Mn2+, Cd2+, Sr2+, Hg2+, Co2+, Cr3+, Fe3+, Pb2+, and Mg2+ in acetonitrile were performed. As shown in Fig. 2, upon excitation at 350 nm, the fluorescence of 1o was notably changed only when Mg2+ was added. The addition of other metal ions resulted in no obvious effects on the fluorescence emission of 1o, except for the addition of Cd2+. These results showed the ability of 1o for distinguishing Mg2+ from other metals ions. Therefore, the diarylethene 1o could be used as a selective fluorescent sensor for Mg2+ in acetonitrile. In addition, the experiments of fluorescence response of 1o to Mg2+ in aqueous solution were also performed. As shown in Fig. S5,† there are no obvious changes in fluorescence with the addition of Mg2+, due to the poor water solubility of 1o.
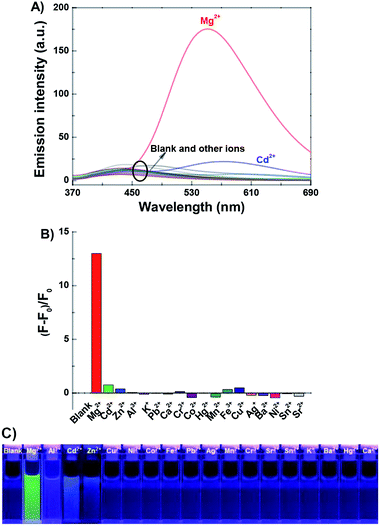 |
| Fig. 2 Upon addition various metal ions to 1o (2.0 × 10−5 mol L−1 in acetonitrile): (A) fluorescence emission spectral changes; (B) emission intensity changes; (C) fluorescent photos (λex = 350 nm). | |
Fluorescence studies of 1o toward Mg2+
The fluorescence titration experiment of 1o toward Mg2+ in acetonitrile was also investigated. As shown in Fig. 3A, 1o exhibited a very weak emission at 442 nm with 350 nm excitation. With the gradual addition of Mg2+, the emission increased inch by inch, accompanied by a clear red shift from 442 nm to 552 nm. When the amount of Mg2+ reached to 3.0 equivalents of 1o, the fluorescence intensity achieved its maximum (Fig. S6†), and the absolute quantum yield of fluorescence was determined to be 0.013. At the same time, the fluorescent color changed from dark purple to green, which was coincident with the changes in the fluorescence spectra. Reversely, with the addition of EDTA (10.0 equivalents of 1o), the fluorescence spectrum recovered gradually to that of 1o, indicating that the complexation reaction between 1o and Mg2+ was reversible. The weak fluorescence of the original 1o was attributed to the C
N bond isomerization, which has long been known as the dominant decay process.71,72 However, a stable chelate 1o–Mg2+ (1o′) was formed in the presence of Mg2+. The isomerization of C
N bond was inhibited, which increased the rigidity of the molecule, resulting in the chelation enhanced fluorescence (CHEF) effect.73
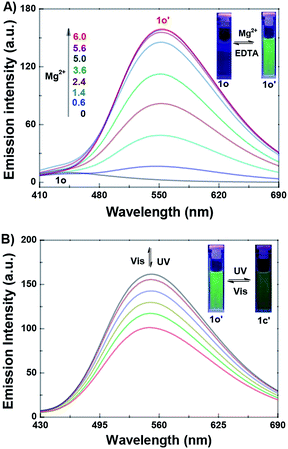 |
| Fig. 3 (A) Fluorescence spectral changes of 1o (2.0 × 10−5 mol L−1 in acetonitrile) induced by Mg2+ (0–6.0 × 10−5 mol L−1); (B) fluorescence spectral changes of 1o′ (2.0 × 10−5 mol L−1 in acetonitrile), upon irradiation with UV/vis lights (λex = 350 nm). | |
The fluorescence switching property of 1o′ was also investigated. As shown in Fig. 3B, the emission intensity of 1o′ gradually decreased with the irradiation of 297 nm light, due to the formation of closed-ring isomer 1c–Mg2+ (1c′).74 When the PSS was reached, the fluorescence of 1o′ was quenched by 37%, accompanied by the fluorescent color changed from green to dark green. Reversely, the fluorescence spectrum of 1o′ could be recovered upon irradiation with visible light (λ > 500 nm). In another way, the fluorescence spectral responses of the closed-ring isomer 1c to Mg2+ were also studied (Fig. S7†). With the addition of Mg2+, the emission intensity of 1c was obviously enhanced, and the emission peak shifted from 442 nm to 552 nm. When the amount of Mg2+ reached 3.0 equivalents, the fluorescence spectrum was consistent with that from 1o′ with 297 nm light. Meanwhile, the fluorescent color changed from dark to dark green. Reversely, upon the addition of EDTA (10.0 equivalents of 1o), the fluorescence spectrum of 1c′ recovered immediately to that of 1c, showing that the complexation–decomplexation reaction between 1c and Mg2+ was also reversible.
Additionally, to evaluate the effects of pH on the sensor, the fluorescence spectral changes of 1o–Mg2+ over different pH values were also studied (Fig. S8†), the pH was adjusted by dropping an appropriate amount of the aqueous solution of HCl and NaOH into acetonitrile, and was measured with Ferromagnetic PHS-3C pH meter. The results showed the optimal pH range for the fluorescence emission of 1o–Mg2+ is 7–9. At the same time, the experiments of fluorescence response of 1o to Mg(NO3)2, MgCl2, and Mg(ClO4)2 were also performed. As shown in Fig. S9,† the emission intensity of 1o + Mg(NO3)2 was much higher than that of 1o + MgCl2 and 1o + Mg(ClO4)2. The results indicated the counter anions have certain influence on the sensing of Mg2+.
Complexation mechanism of 1o with Mg2+
Job's plot analysis was performed to prove the complexation of 1o–Mg2+ according to the previous report.75 The result showed that the emission intensity approached the maximum when the molar fraction of [Mg2+]/([1o] + [Mg2+]) was about 0.5, suggesting a 1
:
1 binding stoichiometry between 1o and Mg2+ in acetonitrile (Fig. 4). Meanwhile, the binding constant (Ka) of 1o and Mg2+ was determined to be 1.14 × 102 L mol−1 with the slope and intercept of the linearity (R = 0.998) (Fig. S10†). According to the reported method,76 the limit of detection of 1o toward Mg2+ was calculated to be 3.58 × 10−7 mol L−1 (Fig. S11†). Therefore, 1o could serve as a highly sensitive fluorescent sensor for the detection of Mg2+ in acetonitrile.
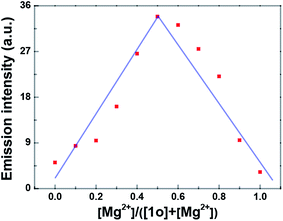 |
| Fig. 4 Job's plot showing the 1 : 1 complex of 1o and Mg2+ in acetonitrile (2.0 × 10−5 mol L−1). | |
Furthermore, 1H NMR titration experiments were carried out in acetonitrile-d3 to further prove the coordination of 1o and Mg2+. As shown in Fig. 5, with the addition of Mg2+, the Hb signal displayed a downfield shift of 0.01 ppm from 6.84 ppm to 6.85 ppm, and the Ha resonance signal at 7.32 ppm disappeared completely, indicating the coordinated bond of O–Mg2+ was formed. At the same time, the Hc on the pyrimidine displayed a shift of 0.02 ppm from 8.40 ppm to 8.38 ppm, showing the formation of the coordinate bond of N–Mg2+. These results indicated that the O of the hydroxyl group, the N of pyrimidine are the most likely binding sites. Moreover, the HRMS analysis was also carried out to confirm the interaction between 1o and Mg2+. The testing sample was prepared by adding Mg2+ to 1o in acetonitrile, and the result indicated that a signal located at m/z = 663.0922 was consistent well with the ensemble [1o + Mg2+ + NO3−]+ (m/z calcd: 663.0987) (Fig. S12†). In addition, the IR spectral experiments of 1o and 1o–Mg2+ have been also performed at room temperature. As shown in Fig. S13,† the peak at 1608 cm−1 corresponds to C
N stretching. Upon the complexation of 1o and Mg2+, the peak at 1608 cm−1 shifted to 1635 cm−1 due to the rigidification of the imine bond. Besides, the peak at 3440 cm−1 assigned to the stretching vibration of –OH shifted to 3408 cm−1. The strong absorption peak at 1383 cm−1 was attributed to the added NO3−. These results further proved that 1o and Mg2+ formed the 1
:
1 complex. Based on these results, the proposed binding mode was shown in Scheme 2.
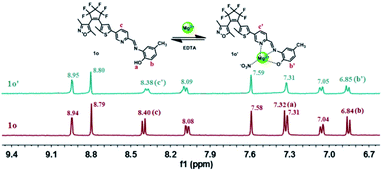 |
| Fig. 5 Changes in 1H NMR of 1o and 1o′ in acetonitrile-d3 (Inset shows the proposed binding mode of 1o′ complex). | |
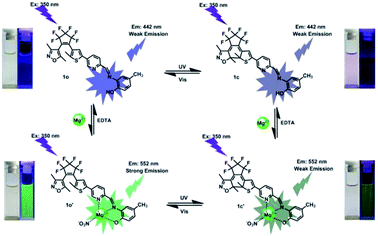 |
| Scheme 2 Dual-controlled fluorescent-switching behavior of 1o induced by Mg2+/EDTA and UV/vis light. | |
Application in practical sample and logic circuit
To study the practical application ability of 1o in Mg2+ detection, the competitive experiments for the fluorescence response of 1o in the presence of Mg2+ and other metal ions were carried out in acetonitrile. As shown in Fig. S14,† the fluorescence response of 1o to Mg2+ was not affected by other competing metal ions, expect for Cu2+, Co2+, Ni2+. The results indicated that 1o has reasonable anti-interference ability on sensing Mg2+ in acetonitrile. Meantime, the application of 1o to real samples was also researched. The Mg2+ content in actual water samples from the Gan-Jiang River in Nanchang, Jiangxi province was measured. Table 1 showed the results determined with 1o after the addition of a moderate amount of Mg2+. The recoveries ranged from 94.3% to 103%. The results indicated that 1o could be used to detect Mg2+ in real samples with high accuracy. Furthermore, on the basis of the photoswitching characteristics of 1o modulated by either UV/vis lights or chemical reagents stimuli in acetonitrile, a logic circuit was constructed with four input signals including In1: 297 nm light, In2: >500 nm light, In3: Mg2+, In4: EDTA and one output signal (Opt: emission intensity at 552 nm) (Fig. S15, Table S1†).77,78 These results show that sensor 1o has certain practical value in real sample detection and logic circuit.
Table 1 Application in actual water sample detection for Mg2+
Sample no. |
Mg2+ added (μM) |
Mg2+ determined (μM) |
Recovery (%) |
1 |
4 |
4.11 |
103 |
2 |
8 |
7.63 |
95.4 |
3 |
12 |
11.32 |
94.3 |
4 |
16 |
16.39 |
102 |
Conclusions
In summary, a highly selective fluorescent sensor toward Mg2+ based on diarylethene and 4-methylphenol unit was successfully developed. This sensor exhibited outstanding photochromic and fluorescent switching properties in acetonitrile solution. The application results indicated that the sensor could be used to detect Mg2+ in real samples. Furthermore, a logic circuit was designed with the fluorescence intensity at 552 nm as output signal, the UV/vis lights and Mg2+/EDTA as input signals. All of the results will be helpful for the design and synthesis of new sensors for the recognition of Mg2+ with high selectivity in the future.
Conflicts of interest
There are no conflicts of interest to declare.
Acknowledgements
The authors are grateful for the financial support from the National Natural Science Foundation of China (41867053), the “5511” science and technology innovation talent project of Jiangxi (2016BCB18015), the key project of Natural Science Foundation of Jiangxi Province (20171ACB20025), the Project of the Science Funds of the Education Office of Jiangxi (GJJ160773), the Young Talents Project of Jiangxi Science and Technology Normal University (2015QNBJRC004), the Project of Jiangxi Science and Technology Normal University Advantage Sci-Tech Innovative Team (2015CXTD002).
Notes and references
- M. J. Cromie, Y. Shi and T. Latifi, Cell, 2006, 125, 71–84 CrossRef CAS PubMed.
- G. Farruggia, S. Iotti and L. Prodi, J. Am. Chem. Soc., 2006, 128, 344–350 CrossRef CAS PubMed.
- L. Jin, Z. Guo and Z. Sun, Sens. Actuators, B, 2012, 161, 714–720 CrossRef CAS.
- D. Ray, A. Nag and A. Jana, Inorg. Chim. Acta, 2010, 363, 2824–2832 CrossRef CAS.
- B. O'Rourke, P. H. Backx and E. Marban, Science, 1992, 257, 245–248 CrossRef.
- C. Schmitz, A. L. Perraud and C. O. Johnson, Cell, 2003, 114, 191–200 CrossRef CAS PubMed.
- Y. Zhao, A. M. Ren and L. Y. Zou, Theor. Chem. Acc., 2011, 130, 61 Search PubMed.
- S. Ishijima, A. Uchibori and H. Takagi, Arch. Biochem. Biophys., 2003, 412, 126–132 CrossRef CAS PubMed.
- H. C. Politi and R. R. Preston, NeuroReport, 2003, 14, 659–668 CrossRef PubMed.
- H. Rubin, Arch. Biochem. Biophys., 2007, 458, 16–23 CrossRef CAS PubMed.
- F. I. Wolf, A. Torsello and S. Fasanella, Mol. Aspects Med., 2003, 24, 11–26 CrossRef CAS PubMed.
- K. Seki, K. Aizawa and T. Sugaoi, Chem. Lett., 2008, 37, 872–873 CrossRef CAS.
- B. O'Rourke, P. H. Backx and E. Marban, Science, 1992, 257, 245–248 CrossRef.
- R. Bogoroch and L. F. Belanger, Anat. Rec., 1975, 183, 437–447 CrossRef CAS PubMed.
- H. O. Trowbridge and J. L. Seltzer, J. Periodontal Res., 1967, 2, 147–153 CrossRef CAS PubMed.
- L. Wang, W. Qin and X. Tang, J. Phys. Chem. A, 2011, 115, 1609–1616 CrossRef CAS PubMed.
- N. E. L. Saris, E. Mervaala and H. Karppanen, Clin. Chim. Acta, 2000, 294, 1–26 CrossRef CAS.
- J. A. M. Maier, Mol. Aspects Med., 2003, 24, 137–146 CrossRef CAS PubMed.
- M. Barbagallo and L. J. Dominguez, Arch. Biochem. Biophys., 2007, 458, 40–47 CrossRef CAS PubMed.
- T. Hashimoto, K. Nishi and J. Nagasao, Brain Res., 2008, 1197, 143–151 CrossRef CAS PubMed.
- R. Swaminathan, Clin. Biochem. Rev., 2003, 24, 47 CAS.
- W. Jahnen-Dechent and M. Ketteler, Clin. Kidney J., 2012, 5, 3–14 CrossRef PubMed.
- R. M. Touyz, Front. Biosci., 2004, 9, 1278–1293 CrossRef CAS.
- Y. Rayssiguier, E. Gueux and W. Nowacki, Magnesium Res., 2006, 19, 237–243 CAS.
- M. Barbagallo, L. J. Dominguez and A. Galioto, Mol. Aspects Med., 2003, 24, 39–52 CrossRef CAS PubMed.
- J. Zhu, Y. Qin and Y. Zhang, Anal. Chem., 2009, 82, 436–440 CrossRef PubMed.
- H. Hifumi, A. Tanimoto and D. Citterio, Analyst, 2007, 132, 1153–1160 RSC.
- M. Ishida, Y. Naruta and F. Tani, Angew. Chem., Int. Ed., 2010, 49, 91–94 CrossRef CAS PubMed.
- S. Patra and P. Paul, Dalton Trans., 2009, 40, 8683–8695 RSC.
- K. B. Kim, H. Kim and E. J. Song, Dalton Trans., 2013, 42, 16569–16577 RSC.
- S. Goswami, A. Manna and S. Paul, Dalton Trans., 2013, 42, 8078–8085 RSC.
- V. K. Gupta, N. Mergu and L. K. Kumawat, Sens. Actuators, B, 2015, 207, 216–223 CrossRef CAS.
- G. Farruggia, S. Iotti and L. Prodi, J. Am. Chem. Soc., 2007, 129, 1470 CrossRef CAS.
- H. Hama, T. Morozumi and H. Nakamura, Tetrahedron Lett., 2007, 48, 1859–1861 CrossRef CAS.
- K. C. Song, M. G. Choi and D. H. Ryu, Tetrahedron Lett., 2007, 48, 5397–5400 CrossRef CAS.
- H. M. Kim, P. R. Yang and M. S. Seo, J. Org. Chem., 2007, 72, 2088–2096 CrossRef CAS PubMed.
- Y. Liu, Z. Y. Duan and H. Y. Zhang, J. Org. Chem., 2005, 70, 1450–1455 CrossRef CAS PubMed.
- B. J. Sanghavi, W. Varhue and J. L. Chávez, Anal. Chem., 2014, 86, 4120–4125 CrossRef CAS PubMed.
- B. J. Sanghavi, S. Sitaula and M. H. Griep, Anal. Chem., 2013, 85, 8158–8165 CrossRef CAS PubMed.
- B. J. Sanghavi, S. M. Mobin and P. Mathur, Biosens. Bioelectron., 2013, 39, 124–132 CrossRef CAS PubMed.
- B. J. Sanghavi and A. K. Srivastava, Electrochim. Acta, 2010, 55, 8638–8648 CrossRef CAS.
- B. J. Sanghavi and A. K. Srivastava, Electrochim. Acta, 2011, 56, 4188–4196 CrossRef CAS.
- P. S. Hariharan and S. P. Anthony, RSC Adv., 2014, 4, 41565–41571 RSC.
- H. Sharma, N. Kaur and A. Singh, J. Mater. Chem. C, 2016, 4, 5154–5194 RSC.
- V. K. Gupta, N. Mergu and L. K. Kumawat, Sens. Actuators, B, 2016, 223, 101–113 CrossRef CAS.
- M. Liu, X. Yu and M. Li, RSC Adv., 2018, 8, 12573–12587 RSC.
- G. T. Selvan, V. Chitra and V. M. V. Enoch Israel, New J. Chem., 2018, 42, 902–909 RSC.
- J. H. Hu, J. B. Li and Y. Sun, RSC Adv., 2017, 7, 29697–29701 RSC.
- Y. Ma, H. Liu and S. Liu, Analyst, 2012, 137, 2313–2317 RSC.
- G. Men, C. Chen and S. Zhang, Dalton Trans., 2015, 44, 2755–2762 RSC.
- Q. Lin, J. J. Gruskos and D. Buccella, Org. Biomol. Chem., 2016, 14, 11381–11388 RSC.
- X. Zhu, C. He and D. Dong, Dalton Trans., 2010, 39, 10051–10055 RSC.
- G. Zhang, J. J. Gruskos and M. S. Afzal, Chem. Sci., 2015, 6, 6841–6846 RSC.
- G. Wang, J. Qin and L. Fan, J. Photochem. Photobiol., A, 2016, 314, 29–34 CrossRef CAS.
- M. Irie, T. Fukaminato, T. Sasaki, N. Tamai and T. Kawai, Nature, 2002, 420, 759 CrossRef CAS PubMed.
- H. Tian and S. Yang, Chem. Soc. Rev., 2004, 33, 85–97 RSC.
- K. Matsuda and M. Irie, J. Photochem. Photobiol., C, 2004, 5, 169–182 CrossRef CAS.
- S. Z. Pu, H. Ding and G. Liu, J. Phys. Chem. C, 2014, 118, 7010–7017 CrossRef CAS.
- Q. Zou, X. Li and J. Zhang, Chem. Commun., 2012, 48, 2095–2097 RSC.
- H. Liu and Y. Chen, Eur. J. Org. Chem., 2009, 30, 5261–5265 CrossRef.
- Q. Zou, J. Jin and B. Xu, Tetrahedron, 2011, 67, 915–921 CrossRef CAS.
- Z. Zhou, H. Yang and M. Shi, ChemPhysChem, 2007, 8, 1289–1292 CrossRef CAS PubMed.
- Z. Zhou, S. Xiao and J. Xu, Org. Lett., 2006, 8, 3911–3914 CrossRef CAS PubMed.
- Z. Li, C. Zhang and Y. Ren, Org. Lett., 2011, 13, 6022–6025 CrossRef CAS PubMed.
- S. Z. Pu, D. Jiang and W. Liu, J. Mater. Chem., 2012, 22, 3517–3526 RSC.
- S. Q. Cui, Z. Tian and S. Z. Pu, RSC Adv., 2016, 6, 19957–19963 RSC.
- S. Z. Pu, Z. P. Tong, G. Liu and R. J. Wang, J. Mater. Chem. C, 2013, 1, 4726–4739 RSC.
- M. Irie, Chem. Rev., 2000, 100, 1685–1716 CrossRef CAS.
- Z. X. Li, L. Y. Liao and W. Sun, J. Phys. Chem. C, 2008, 112, 5190–5196 CrossRef CAS.
- S. Z. Pu, J. Xu and L. Shen, Tetrahedron Lett., 2005, 46, 871–875 CrossRef CAS.
- Z. Wang, S. Q. Cui, S. Y. Qiu and S. Z. Pu, Spectrochim. Acta, Part A, 2018, 205, 21–28 CrossRef CAS PubMed.
- W. K. Dong, X. L. Li, L. Wang, Y. Zhang and Y. J. Ding, Sens. Actuators, B, 2016, 229, 370–378 CrossRef CAS.
- E. T. Feng, Y. Y. Tu, C. B. Fan, G. Liu and S. Z. Pu, RSC Adv., 2017, 7, 50188–50194 RSC.
- Z. Wang, S. Q. Cui, S. Y. Qiu and S. Z. Pu, RSC Adv., 2018, 8, 29295–29300 RSC.
- J. S. Wu, W. M. Liu and X. Q. Zhuang, Org. Lett., 2007, 9, 33–36 CrossRef CAS PubMed.
- H. Wang, B. Wang and Z. Shi, Biosens. Bioelectron., 2015, 65, 91–96 CrossRef CAS PubMed.
- Z. Wang, S. Q. Cui, S. Y. Qiu and S. Z. Pu, J. Photochem. Photobiol., A, 2018, 367, 212–218 CrossRef CAS.
- Z. Wang, S. Q. Cui, S. Y. Qiu and S. Z. Pu, Tetrahedron, 2018, 74, 7431–7437 CrossRef CAS.
Footnote |
† Electronic supplementary information (ESI) available. See DOI: 10.1039/c8ra10470k |
|
This journal is © The Royal Society of Chemistry 2019 |