DOI:
10.1039/C8RA10429H
(Paper)
RSC Adv., 2019,
9, 9145-9152
Efficient removal of sulfamerazine (SMR) by ozonation in acetic acid solution after enrichment SMR from water using granular activated carbon
Received
20th December 2018
, Accepted 13th March 2019
First published on 19th March 2019
Abstract
Sulfamerazine (SMR) as a persistent organic pollutant in waste streams is of growing environmental concern. This study explores the extraction SMR from water into an acetic acid (AA) solution using granular activated carbon (GAC), and removal of SMR by ozonation in AA solution. Systematic experiments have shown that GAC can be used as an adsorbent to transfer sulfamerazine from water to AA solution. SMR removal efficiency is 99.5% in 10% AA aqueous solution, which is better than in water. The removal rate of SMR in the AA solution decreased as the initial molar ratio of SMR and O3 increased. The removal rate of SMR decreased with Fe3+ present in the reactive system. The removal of SMR is dominated by indirect ozonation in water, while the SMR removal is an effect of both direct and indirect ozonation in AA solution. It is a very efficient process for the degradation of SMR in micro polluted water when using combined GAC adsorption–desorption in AA solution and ozonation of the resulting solution.
1. Introduction
During the last decade, antibiotics as emerging contaminants are of global concern because antibiotic residues in the environment have the ability to expose the public to serious health hazards.1 About 100
000–200
000 tons of antibiotics are produced annually for use in animal and human health, agriculture and aquaculture activities, which may aid the antibiotic resistance of microorganisms and destroy the environmental micro-ecosystem.2–4 Sulfonamides are one of the most popular groups of antibiotics that have been used for several decades in both human and veterinary medicine, for therapeutic treatment of infections related diseases.5–7 However, the residual sulfonamides can enter the environment through various pathways and persist for a long time. Even at low concentrations, the prolonged exposure to the sulfonamide antibiotics may cause harmful effects on humans and aquatic life, such as green algae, Daphnia magna, and lemna minor.8,9
Considering serious menace to the environment caused by low-concentration antibiotics in the aquatic system, the development of efficient removal methods in advanced water treatment is becoming an urgent matter.10 The removal of antibiotics has been tested using various processes such the photo-Fenton process, photochemical oxidation technique, UV-irradiation, electrochemical processes and adsorption.11–18 However, electrochemical and photo-Fenton processes seem suitable to treat toxic wastewaters with high concentrations of antibiotics; other techniques, to a certain extent, are blamed for the high investment and processing cost and low qualified rate in wastewater treatment.2,19 Adsorption has become an emerging method in waste removal because of its simplicity, low operation cost.20 The most widely reported adsorbents for antibiotic include ACs, CNTs, bentonite, ion exchange resins, BCs and so on. Considering the easy availability of raw materials, high surface area, degree of micro porosity and high adsorption capacity, activated carbon was used to adsorb sulfamerazine (SMR) in wastewater containing organic contaminants,21,22 and desorbed SMR from activated carbon by acid solution. Nevertheless, it is not appropriate to recycle or reuse acidic solutions containing antibiotics and other mixtures to desorb antibiotics from activated carbon. This method is not appropriate in complex water, containing low-concentration antibiotics, due to low recovery value.
Ozonation is a method for removing antibiotics and recalcitrant pollutants from wastewater, which has unique advantages like easy equipment, easy operation and high speed.23 It is widely acknowledged that ozone can react with various organic compounds in aqueous solution in two ways: a direct reaction with molecular ozone or a radical reaction involving hydroxyl radicals induced by ozone decomposition. Therefore, the removal of antibiotics in water by ozonation is affected by many factors, including solution pH, inorganic anions and cations, dissolved organic matter, ·OH scavenger and the type of water matrices.24,25 Conventional treatment systems dissolve ozone directly into a wastewater by gas/liquid contact. However, ozonation in water is not a universally efficient process, because current ozone-generating technology is incapable of producing gas concentrations of more than several percent and the solubility of ozone in water is relatively poor (approximately 0.2 mg L−1 per mg L−1 in contacting gas phase).26 Due to poor oxidation selectivity and, particularly, a low utilization ratio and the high energy consumption of ozone in conventional water/gas ozonation systems, more recent techniques increase production costs to added catalysts and/or irradiation to the oxidation medium to improve oxidation efficiency.27,28 G. Li et al.22 studies have shown that the removal efficiency was more than 80% for the studied SMR by an integrated ozonation and biological activated carbon filtration (O3-BAC) process, under the concentration of SMR is 1000 ng L−1 at ozone doses of 0.5 and 2.5 mg L−1. The removal rate at high concentrations of SMR was lower than that of low concentrations. As a result, these factors combine to impede the broad application of ozonation in wastewater treatment.29
In order to solve the above problems, as conventional adsorbent, granular activated carbon (GAC) was used to extract organic pollutants from wastewater. GAC loaded with organic pollutants was washed with organic solvents such as acetic acid (AA), acetone and tert-butyl alcohol. Organic pollutants were decomposed by ozonation more efficiently in organic solvents than those in water. In addition, using organic solvents prevents the unnecessary formation of ozonation by-products that occur in water. Because organic solvents or saturated carboxylic acids reacts slowly with ozone and in many cases not be completely oxidized,15 they can be reused to extract organic pollutants from adsorbents loaded with organic pollutants. In the previous studies, ozonation of chlorinated organic compounds in organic solvents as an effective process, was used in the efficient treatment of wastewater.30–32
There are little comprehensive studies known about the removal of SMR with ozonation in AA solution after extracted SMR using GAC from wastewater. In this paper, the purpose of the study is to investigate the primary factors that may influence the effect of ozonation removal of SMR in AA solution, with the aim of providing a critical application for the practical treatment of wastewater containing low-concentration antibiotics.
2. Materials and methods
2.1. Materials
The reagents used in this study include methanol (HPLC, TEDIA Company), sulfamerazine (SMR, Nanjing Boquan Technology Co., Ltd., purity higher than 95%) and acetic acid (AR, Sinopharm Chemical Reagent CO., Ltd). The GAC was purchased from Tianjing Activated Carbon Co., Ltd (Tianjing, China). The GAC was pulverized into a 100 mesh powder, and then was soaked in 0.01 mol L−1 HCl solution for 72 hours. The samples were then rinsed with deionized water to a neutral pH, dried at 120 °C in an oven and stored in a desiccator. Ozone generator (3–10 g h−1, Nanjing Woer Technology Co., Ltd.) with industrial oxygen (99.5%) was used to provide the source gas. The concentration of SMR was measured using a liquid chromatographer (LC-100P, Shanghai Wufeng Scientific Instruments Co., Ltd.).
2.2. Adsorption and desorption experiments
Adsorption experiment. The adsorption kinetics study of GAC was performed by adding 0.01, 0.02, 0.03, 0.04, 0.05, 0.06 g samples and 100 mL 150 mg L−1 SMR solutions to 250 mL glass vials. HCl was added drop wise to the solution for adjusting the pH to 2.1. Then, all samples were shaken at a constant agitation speed (220 rpm) at 25 ± 0.5 °C. Samples were obtained at 5, 10, 20, 40, 60, 90, 120, 180, 360, 540, 720, 1080, 1440, 2880, 7200 min, respectively.33 After reaching equilibrium, the solution was separated from the sorbent by centrifugation at 13
000 rpm for 5 min and filtered with a 0.45 μm pore size syringe filter. Freundlich isotherms were taken to analyze the adsorption capacity of GAC for SMR.
Extraction experiments. Acetic acid was used to desorb SMR from GAC. GAC (0.4 g L−1) containing SMR was placed in a 50 mL vial with 40 mL of AA solution (5%, 10% and 30% (v/v)). The vial was sealed with a Teflon coated butyl rubber septum and shaken at 120 rpm for 24 hours at 25 °C. Extract solution was separated by centrifugation. After three extractions, three rounds of extract solution containing SMR were combined for ozonation. After cleaning with water, GAC was used for next adsorption. After ozonation, acetic acid solution was used for the next extraction of SMR. GAC was not involved in the oxidative degradation of SMR by ozone in AA solution. After cleaning with water, GAC was used for next adsorption.In this work, pure oxygen was used as source to generate ozone constantly. Acetic acid solution (150 mL) containing SMR (200 mg L−1) was placed in the reactor with a G3 glass gas diffuser, where the available capacity of the reactor was 1 L. Thus, O3 was bubbled from the gas diffuser into the glass reactor at a speed of 0.05 m3 h−1, 4.81 mg O3 per min. Vapor–liquid contact oxidation between ozone and SMR occurred in the glass reactor. Similar experiments were carried out with AA solutions containing tertiary butyl alcohol (TBA) 20 mmol L−1 and Fe3+ respectively. Blank solution, without AA, was prepared by using sulfuric acid to adjust the pH to 2.0. The whole experiment was conducted in a closed environment without lights. Samples were collected at a certain time interval during each reaction, and a 5 μL, 0.025 mol L−1 Na2S2O3 solution was added to each sample immediately after collection, to terminate the oxidation reaction.
2.3. Analytical methods
The textural properties of the GAC were characterized using scanning electron microscope (SEM) (JSM-5610LV, Jeol Ltd., Japan). The concentration of ozone in solution was determined by the indigo method. The SMR in solution was determined by liquid chromatography with a UV/Vis detector and an ODS-C18 column. The detection was made with a 1.0 mL min−1 mobile phase (with a concentration of 0.01 mol L−1, a pH of 3.0, the ratio between phosphate buffer solution and acetonitrile of 20/80, and H3PO4 used to adjust the pH of the water phase) at 280 nm, and a column temperature of 30 °C for SMR. Calibration curves remained linear through the detection limit range from 0.02 mg L−1 to at least 5 mg L−1. The average rate of SMR recovery was 98.5%. All samples were measured in triplicates. Relative standard deviation was less than 10%. The injection volume was 0.02 mL.
HPLC-MS methods have been were observed during the ozonation degradation of sulfamerazine in reversed-phase mode on an Agilent ZORBAX SB-C18 column (1.8 μm, 50 × 4.6 mm; Agilent) using a 1260 Infinity LC system (Agilent, USA). Mobile phases consisted of 0.1% trifluoroacetic acid (TFA) in water (eluent A) and acetonitrile (eluent B). The following gradient was applied: 0 min – 20% B, 1 min – 20% B, 3 min – 80% B, 4 min – 80% B, 4.1 min – 20% B, 7.1 min – 20% B. The MS was operated in the positive ESI mode. The MS parameters were as follow: capillary 4500 V, source temperature 100 °C, desolvation temperature 350 °C, RF voltage 0.2 V, cone backflush flow 50 L h−1, cracking voltage 135 V, sheath gas (nitrogen) 0.75 L min−1, auxiliary gas (nitrogen) gas 0.15 L min−1. The MS spectra were acquired over an m/z range of 50–400.
3. Results and discussion
3.1. Adsorption and extraction of SMR by GAC
The porous structure of the surface can be seen by SEM of GAC (Fig. 1a). Moreover, a large number of porous and loose structures can be observed on the surface of the GAC after being soaked with acid (Fig. 1b and c). GAC has gained widespread use for the adsorption of pollutants main due to its large surface area, porous structure and highly active surface.34 Therefore, the strong adsorption ability of GAC is utilized for SMR adsorption experiments.
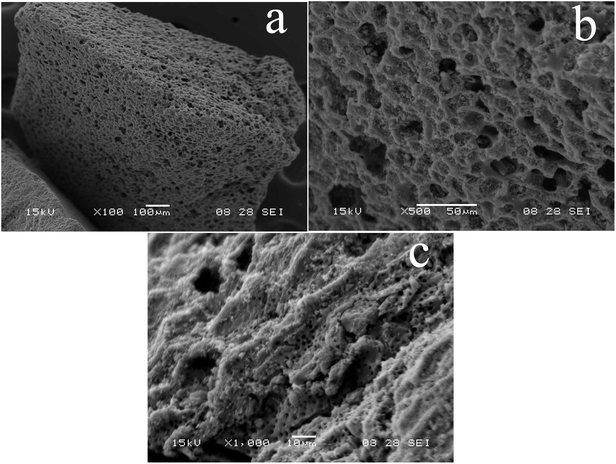 |
| Fig. 1 Scanning electron microscope of granular activated carbon. (a) scale bar is 100 μm, (b) scale bar is 500 μm, (c) scale bar is 1000 μm. | |
The adsorptive capacity of GAC to SMR at different concentrations was different (Fig. 2a). There was a positive correlation between concentration of GAC and adsorption capacity. When the concentration of GAC is 0.6 g L−1, the maximum adsorption capacity can reach 165.67 mg g−1 compared with other concentration conditions. The amount SMR adsorbed by GAC varies with time. But the adsorption will reach equilibrium state after 1440 min of reaction time. Using the Freundlich isotherm to evaluate the adsorption of SMR by GAC.19 Fig. 2b presents the Langmuir isotherm of GAC with SMR is shown. The correlation coefficient of fitting is 0.998. The results show that GAC has a good ability to adsorb SMR from water.
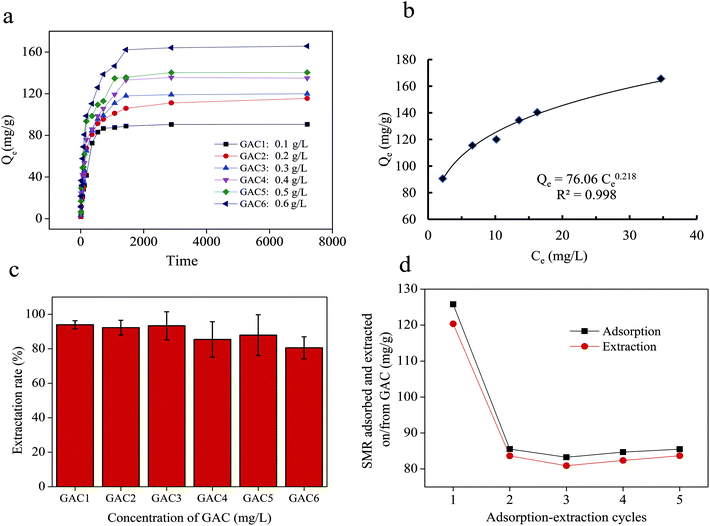 |
| Fig. 2 (a) Effect of time on the adsorption of SMR with different initial GAC concentrations (150 mg L−1 of SMR, 25 ± 0.5 °C), (b) Freundlich adsorption isotherm of GAC for SMR, (c) the extraction rate of SMR from GAC in 10% (v/v) AA solution under different concentration of GAC, (d) the relationship between the amount of SMR adsorbed by, and extracted from GAC (10% (v/v) AA solution). | |
The GAC adsorbed with SMR was extracted in 10% (v/v) AA solution for 4 h, then the concentration of SMR dissolved in AA solution was determined. The extraction rate of SMR in 10% (v/v) AA solution were 93.92%, 92.24%, 93.32%, 85.42%, 87.89% and 80.56%, respectively, when the concentration of GAC were 0.1, 0.2, 0.3, 0.4, 0.5, 0.6 g L−1 (Fig. 2c).
To better evaluate changes in adsorbability and extractability, the adsorption and extraction of SMR was repeated five times. Fig. 2d reveals the amount of SMR adsorbed by and extracted from GAC. GAC adsorption capacity were 125.78, 85.52, 83.26, 84.69 and 85.5 mg g−1 and the extraction capacity were 120.36, 83.62, 80.91, 82.37, 83.68 mg g−1 in five adsorption–extraction cycles, respectively. The first step has a considerably higher amount of adsorption than the second step (67.99% of first step). This may be due to the fact that some AA solution occupies the holes on the surface of GAC after the first step of extraction, which results in the decrease of adsorption sites on the surface of GAC. However, it was observed that the following adsorption and extraction remained relatively stable. Regarding the ratio of extraction and adsorption, it held almost constant for all five steps and the SMR adsorbed on GAC was completely extracted after the second step. The ratio of each extraction to adsorption is 0.96, 0.98, 0.97, 0.97 and 0.98, respectively. The results show that the SMR adsorbed by GAC in water can be extracted well in AA solution under the condition of repeated use. These results indicate that GAC can be used as an adsorbent to transfer SMR from water in AA solution.
3.2. Effects of acetic acid concentration on the removal of SMR
As can be seen from Fig. 3, SMR removal in AA solution is more efficient than that in water as the initial concentration of SMR is 200 mg L−1, the ozone flow rate is 0.05 m3 h−1, and the ozone generation rate is 4.81 mg min−1. There are two reasons for removal rate of SMR is higher in AA solution than in water: AA reduces the ozone decomposition rate, ozone has a larger solubility in AA solution. Furthermore, the SMR removal efficiency is better in 5% AA solution than that of in 10% or 30% AA solution. This phenomenon could be explained that there may exist concurrent direct and indirect ozone oxidation reactions when ozone is in the acidic medium. Thus, the increase in the concentration of acetic acid gradually reduces the ozone oxidation reaction rate,35 which further reduces the overall removal rate of SMR. According to account the extraction SMR from GAC, AA concentration was fixed at 10% in the following experiment. A very important point is that the removal of SMR does not mean its combination with AA but its degradation. Two intermediates were observed during the ozonation degradation of sulfamerazine, which are completely degraded during the ozonation for reactive time more than 8 minutes. No other intermediates were detected by HPLC-MS when reactive time more than 8 minutes. In addition, it is important to point out that TOC can not be used in this test to monitor mineralization of the SMR because of the huge concentration of AA.
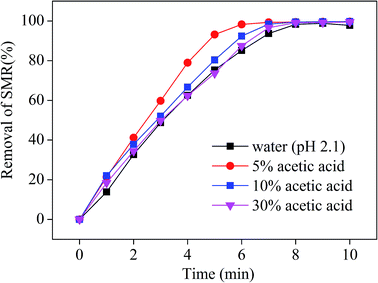 |
| Fig. 3 Effects of concentration of acetic acid on the removal of SMR by ozonation. | |
3.3. Effects of the initial molar ratio of SMR and O3 on the removal of SMR
To investigate the effect of the initial molar ratio of SMR and O3 on TC removal, the different SMR/O3 ratios were given (1.21
:
1, 1.51
:
1, 1.82
:
1 and 2.12
:
1, respectively) in the 10% AA solution with the O3 flux at 0.05 m3 h−1 and O3 generation rate at 4.81 mg min−1. As shown in Fig. 4 shows that the removal rate of SMR in the AA solution decreases as the initial molar ratio of SMR and O3 increases. More O3 is needed to be consumed as a result of the initial molar ratio of SMR and O3 increases.36 In the meantime the concentration of intermediates of SMR oxidation increases, further consuming O3.37 The results showed that the removal efficiency of SMR was worse with the increase of the ratio of SMR and O3. SMR with high concentration extracted into AA solution can be better removed by increasing ozone concentration. However, considering the economic benefits, SMR can be better removed when the ratio of SMR and O3 is 1.82
:
1 than that of other ratio conditions.
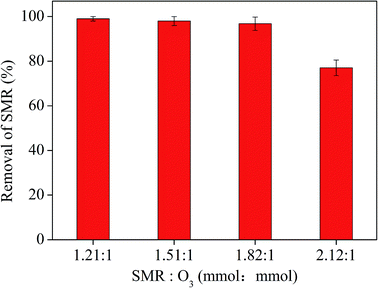 |
| Fig. 4 Effect of the initial molar ratio of SMR and O3 on the removal of SMR in acetic acid aqueous solution. | |
3.4. Effects of the free radical scavenger on the removal of SMR
Free radical scavengers are compounds which compete with other hazardous organics for ·OH during single-phase ozonation reactions, such as tertiary butyl alcohol (TBA). It was found that TBA can significantly reduces the degradation efficiency of pollutants in the process of treating industrial wastewater.38 In this study, TBA with a concentration of 20 mmol L−1 in both water (adjusting pH to 2.1 with sulfuric acid) and 10% AA solutions was used to investigate the effects of a free radical scavenger on the removal of SMR. This effect was evaluated with an initial SMR concentration of 200 mg L−1, an O3 flux of 0.05 m3 h−1, and an ozone generation rate of 4.81 mg min−1. The effect of TBA on the removal of SMR in the 10% AA solution and aqueous phases is depicted in Fig. 5. Compared with the reactive system of no TBA, SMR removal by ozonation is considerably inhibited by the addition of TBA at the beginning of the process. In the presence of TBA, as a whole, the inhibition effect of on SMR removal is similar in both AA solution and water. This results might be due to the strong affinity between TBA and ·OH (k = 5 × 108 mol dm−3 s−1), which inhibits the production of ·OH and further inhibits indirect ozonation.39 The above result illustrates that SMR removal in water is dominated by indirect ozonation, while the SMR removal in AA solution is an effect of both direct and indirect ozonation.40
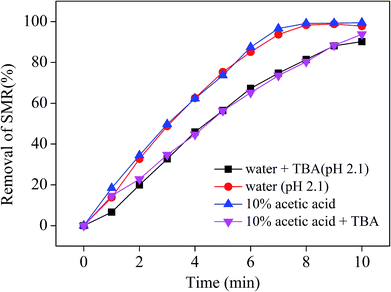 |
| Fig. 5 Effect of free radical scavenger on the removal of SMR by ozonation in 10% acetic acid and aqueous phases. | |
3.5. Effects of metal ion on the removal of SMR by ozonation in acetic acid
In previous work it was found that conventional metal ions with invariable valence had little catalytic effect on ozonation, while obvious changes in ozonation rate were observed in the reactive system containing ions with variable valence.41 The kinetics and yield of ·OH formation in ozone decomposition were affected by the addition of variable valence metal ions under different conditions.42 Various metals can present in AA solution when AA is used as a solvent for the desorption process. Iron is a transition metal widely existing in natural and waste waters. In this work, a variable valence, iron was selected to investigate the effect of metal ions on SMR removal by ozonation in 10% AA solution. Fe3+ was added to the solution with an initial SMR concentration of 200 mg L−1, O3 flux of 0.05 m3 h−1, and O3 generation rate of 4.81 mg min−1. Fig. 6a shows that Fe3+ ions significantly reduces the SMR removal rate by ozonation in AA solution. Some researchers have pointed that the metal–tetracycline complex has low reactivity with ozone because of the formation of stable complexes between tetracycline and metal ions.32,43,44 In contrast with water, the effect of Fe3+ ions on ozonation in AA solution implies that the ozonation mechanism of SMR in AA solution is not same as that in water. For this reason, experiments were carried out to determine electrical conductivity (EC) of a 10% AA solution containing SMR and Fe3+, which can investigate the interaction between metal ions and SMR. The difference between the electrical conductivity of acetic acid containing SMR and that of acetic acid without SMR was defined as the absolute value of electrical conductivity. The relationship between the absolute value of EC and the concentration of Fe3+ ions is shown in Fig. 6b. Compared with the system of without SMR, the value of electrical conductivity increased with the increase of Fe3+ ions. This might be connected to the fact that metal–SMR complex was not produced in AA solution with the presence of Fe3+.
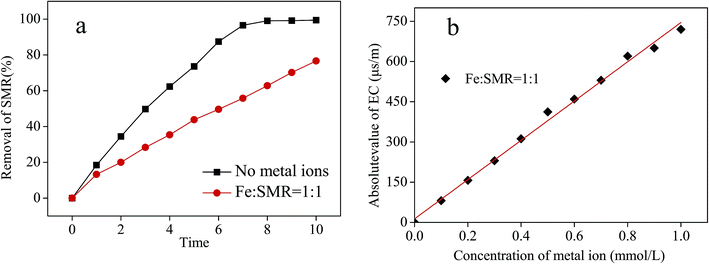 |
| Fig. 6 Effect of metal ion on SMR removal in acetic acid solution (a) and the relationship between the value of electrical conductivity and the concentration of Fe3+ (b). | |
The removal efficiency of SMR was inhibited by the increase of iron ion concentration, with a reduction rate of about 22.83 (Fig. 6a). Some studies have shown that the formation rate of ·OH by ozone in aqueous solution with iron ions is higher than that in the solution without iron ions, under neutral and alkaline conditions.42 However, in this study, SMR was oxidized by ozone in AA solution under acidic conditions. The degradation mechanism of SMR in acetic acid solution may be different from that in water. The mechanism of iron affecting ozonation in AA solution needs further study.
3.6. Effect of reusing AA solvent on the desorption and removal of SMR
To investigate the effect of reusing AA solution on the desorption and removal of SMR, the AA solution was reused in desorption and experiments. Effect of reusing AA solution on desorption and removal of SMR is showed in Fig. 7. During the process of desorption and removal of SMR, the rate of desorption and the rate of removal reach 89.1% and 90.3% respectively, after AA solution was repeated for five desorption cycles. Therefore, as to the concentration of SMR by using by GAC, AA solution is economically sound, efficient and recyclable for desorption and ozonation of SMR in micro-polluted water body.
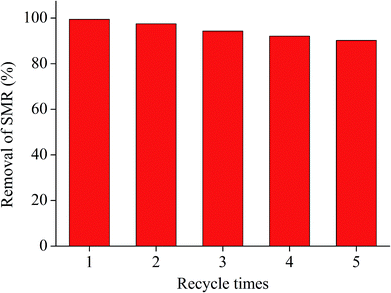 |
| Fig. 7 Reusability of acetic acid solution for the removal of SMR. | |
3.7. The degradation mechanism of SMR removal by ozonation
Three degradation intermediates were identified and denoted as C1, C2 and C3 according to the eluted sequence in the chromatograms (Table 1). C1, with a molecular ion of m/z 156.1, could arise from the cleavage of the S–HN bond in SMR. C2, with a molecular ion of m/z 109.1, was identified to be 2-amino-6-methylpyrimidine, which derived from the other half of the S–HN bond break in SMR. C3, with a molecular ion of m/z 93.1, was identified to be aniline, which derived from the cleavage of the C–S bond in C1.45 No additional peaks appeared in the chromatogram after 8 minutes of reaction.
Table 1 Degradation product of SMR by ozonation
Product |
Formula |
m/z (measured) |
m/z (theoretical) |
Possible structure |
SMR |
C11H12N4O2S |
265.07 |
264.30 |
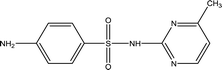 |
C1 |
C6H6NO2S+ |
157.01 |
156.01 |
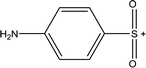 |
C2 |
C5H7N3 |
110.07 |
109.06 |
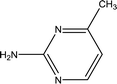 |
C3 |
C6H7N |
92.04 |
93.06 |
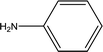 |
Overall, the degradation pathway of SMR during ozonation were proposed based on the three intermediates (Fig. 8). Firstly, S–HN bond cleavage to form [C6H6NSO2]+ and 2-amino-6-methylpyrimidine (C5H7N3). Then, the S–C bond of C1 is cleaved to form aniline (C6H7N) and sulfate ions. Finally, C2 and C3 are further decomposed in a strong oxidation system.
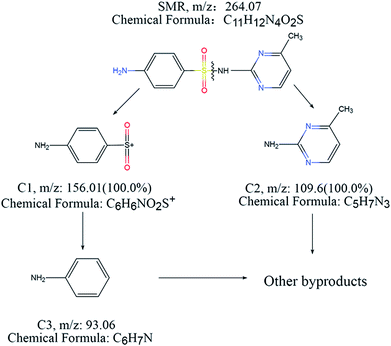 |
| Fig. 8 Proposed degradation pathways of SMR during ozonation. | |
4. Conclusion
The following conclusions can be drawn: GAC can be used as an adsorbent to transfer SMR from water to acetic acid. Acetic acid solution is a better desorbing agent for GAC carried with SMR, and a better reaction medium for SMR removal by ozonation. Acetic acid solution and GAC can be recycled. Ozonation of solutions of SMR in acetic acid allows the complete removal of this pollutant and also the depletion of the reaction intermediates. The removal rate of SMR in the AA solution decreases as the initial molar ratio of SMR and O3 increases. The ozonation mechanism of SMR is dominated by both direct and indirect ozonation in acetic acid solution. Fe3+ can inhibit the degradation of SMR by ozone in an acetic acid solution. Combined GAC adsorption–desorption in AA solution and ozonation of the resulting solution is a very efficient process for the degradation of diluted wastes.
Conflicts of interest
There are no conflicts to declare.
Acknowledgements
We gratefully acknowledge financial support by the National Natural Science Foundation of China (Grant No. 41373111), Jiangsu Provincial Key Laboratory of Carbon and Nitrogen Cycle Processes and Pollution Control, and Jiangsu Center for Collaborative Innovation in Geographical Information Resource Development and Application.
References
- E. Dimiņa, M. Akermanis and U. Dumpis, Proc. Latv. Acad. Sci., Sect. B, 2009, 63(4–5), 253 Search PubMed.
- V. Homem and L. Santos, J. Environ. Manage., 2011, 92(10), 2304 CrossRef CAS PubMed.
- V. Sharma, R. V. Kumar, K. Pakshirajan and G. Pugazhenthi, J. Powder Technol., 2017, 321, 259 CrossRef CAS.
- C. Reyes, J. Fernandez, J. Freer, M. A. Mondaca, C. Zaror, S. Malato and H. D. Man-silla, J. Photochem. Photobiol., A, 2006, 184(1–2), 141 CrossRef CAS.
- W. Baran, E. Adamek, A. Sobczak and A. Makowski, Appl. Catal., B, 2009, 90(3–4), 516 CrossRef CAS.
- J. D. Orwa, J. W. Matofari, P. S. Muliro and P. Lamuka, International Journal of Food Contamination, 2017, 4(1), 5 CrossRef.
- R. Li, Y. L. Zhang, W. L. Chu, Z. X. Chen and J. L. Wang, RSC Adv., 2018, 8(24), 13546–13555 RSC.
- H. M. Khan, H. Bae and J. Y. Jung, J. Hazard. Mater., 2010, 181(1–3), 659 CrossRef PubMed.
- M. R. Azhar, H. R. Abid, H. Periasamy, M. O. Sun, V. Tadé and S. B. Wang, J. Colloid Interface Sci., 2017, 500(15), 88 CrossRef CAS PubMed.
- M. C. Ncibi and M. Sillanpää, J. Hazard. Mater., 2015, 298, 102 CrossRef CAS PubMed.
- I. Michael, E. Hapeshi, C. Michael, A. R. Varela, S. Kyriakou and C. M. Manaia, et al., Water Res., 2012, 46(17), 5621 CrossRef CAS PubMed.
- E. Elmolla and M. Chaudhuri, J. Hazard. Mater., 2009, 170(2–3), 666 CrossRef CAS PubMed.
- E. Elmolla and M. Chaudhuri, J. Hazard. Mater., 2010, 173(1–3), 445 CrossRef CAS PubMed.
- W. Li, X. Peng, H. Wang, Z. Liang and Z. Luan, Fresenius Environ. Bull., 2011, 20(1), 93–98 Search PubMed.
- M. Mehrjouei, S. Müller and D. Möller, Chem. Eng. J., 2015, 263(1), 209 CrossRef CAS.
- G. Moussavi, A. Alahabadi, K. Yaghmaeian and M. Eskandari, Chem. Eng. J., 2013, 217(2), 119 CrossRef CAS.
- J. Rivera-Utrilla, G. Prados-Joya, M. Sánchez-Polo, M. A. Ferro-García and I. Bautista-Toledo, J. Hazard. Mater., 2009, 170(1), 298 CrossRef CAS PubMed.
- J. Chen, J. P. Wang and G. C. Zhang, Chin. J. Environ. Eng., 2012, 6(12), 4493 CAS.
- Z. Aksu and O. Tunç, Process Biochem., 2005, 40(2), 831 CrossRef CAS.
- M. R. Azhar, H. R. Abid, H. Sun, V. Periasamy, M. O. Tadé and S. B. Wang, J. Colloid Interface Sci., 2016, 478, 344 CrossRef CAS PubMed.
- M. B. Ahmed, J. L. Zhou, H. H. Ngo and W. Guo, Sci. Total Environ., 2015, 532, 112 CrossRef CAS PubMed.
- S. Xie, J. Y. Li and Q. X. Zhao, Chin. J. Environ. Eng., 2012, 6(2), 483 CAS.
- A. R. Rahmani, D. Nematollahi, M. R. Samarghandi, M. T. Samadi and G. Azarian, J. Electroanal. Chem., 2018, 808, 82 CrossRef CAS.
- D. B. Mawhinney, B. J. Vanderford and S. A. Snyder, Environ. Sci. Technol., 2012, 46(13), 7102 CrossRef CAS PubMed.
- C. V. Gómez-Pacheco, M. Sánchez-Polo, J. Rivera-Utrilla and J. López-Penalver, Chem. Eng. J., 2011, 178, 115 CrossRef.
- D. B. Ward, C. Tizaoui and M. J. Slater, Ozone: Sci. Eng., 2004, 26(5), 475–486 CrossRef CAS.
- J. Nawrocki and B. Kasprzyk-Hordern, Appl. Catal., B., 2010, 99(1), 27 CrossRef CAS.
- G. Li, W. W. Ben, H. Ye, D. Zhang and Z. M. Qiang, Chem. Eng. J., 2018, 341, 327–334 CrossRef CAS.
- D. B. Ward, C. Tizaoui and M. J. Slater, J. Hazard. Mater., 2005, 125(1–3), 65–79 CrossRef CAS PubMed.
- Y. Nakano, K. Okawa, W. Nishijima and M. Okada, Water Res., 2003, 37(11), 2595 CrossRef CAS PubMed.
- K. Okawa, M. Sasaki, Y. Nakano, W. Nishijima and M. Okada, J. Jpn. Soc. Water Environ., 2003, 26(12), 855 CrossRef CAS.
- S. Y. Li, T. Mei, Y. Peng, S. Y. Ge, G. X. Wang, Y. P. Zhu, L. X. Ni and Y. Zhang, Ozone: Sci. Eng., 2015, 37(5), 405 CrossRef CAS.
- Y. Liu, X. H. Liu, W. P. Dong, L. L. Zhang, Q. Kong and W. L. Wang, Sci. Rep., 2017, 7, 12437 CrossRef PubMed.
- M. Naushad, T. Ahamad, B. M. Al-Maswari, A. A. Alqadami and S. M. Alshehri, Chem. Eng. J., 2017, 330, 1351–1360 CrossRef CAS.
- I. Arslan-Alaton and S. J. Dogruel, J. Hazard. Mater., 2004, 112(1–2), 105 CrossRef CAS PubMed.
- A. Goi, Y. Veressinina and M. Trapido, Ozone: Sci. Eng., 2009, 31(1), 28 CrossRef CAS.
- N. M. Mahmoodi and M. Arami, J. Photochem. Photobiol., A, 2006, 182(1), 60–66 CrossRef CAS.
- C. J. Wang, N. Klamerth, S. A. Messele, A. Singh, M. Belosevic and M. G. El-Din, Water Res., 2016, 100, 476–485 CrossRef CAS PubMed.
- U. von-Gunten, Water Res., 2003, 37(7), 1443 CrossRef CAS.
- J. Staehelin and J. Hoigne, Environ. Sci. Technol., 1985, 19(12), 1206 CrossRef CAS PubMed.
- K. Okawa, T. Y. Tsai, Y. Nakano, W. Nishijima and M. Okada, Chemosphere, 2005, 58(4), 523 CrossRef CAS PubMed.
- Y. Guo, H. J. Wang, B. Wang, S. B. Deng, J. Huang, Y. Gang and Y. J. Wang, Water Res., 2018, 142, 383 CrossRef CAS PubMed.
- S. Cortés, J. Sarasa, P. Ormad, R. Gracia and J. L. Ovelleiro, Ozone: Sci. Eng., 2000, 22(4), 415–426 CrossRef.
- A. Albert and C. W. Rees, Nature, 1956, 177(4505), 433 CrossRef CAS.
- W. W. Ben, Y. W. Shi, W. W. Li, Y. Zhang and Z. M. Qiang, Chem. Eng. J., 2017, 327, 743 CrossRef CAS.
|
This journal is © The Royal Society of Chemistry 2019 |