DOI:
10.1039/C8RA10310K
(Paper)
RSC Adv., 2019,
9, 9533-9545
Novel magnetically separable anhydride-functionalized Fe3O4@SiO2@PEI-NTDA nanoparticles as effective adsorbents: synthesis, stability and recyclable adsorption performance for heavy metal ions
Received
16th December 2018
, Accepted 15th February 2019
First published on 26th March 2019
Abstract
In this paper, a novel adsorbent, Fe3O4@SiO2@PEI-NTDA, was first prepared by the immobilization of an amine and anhydride onto magnetic Fe3O4@SiO2 nanoparticles with polyethylenimine (PEI) and 1,4,5,8-naphthalenetetracarboxylic-dianhydride (NTDA) for the removal of heavy metal ions from aqueous solutions. The structure of Fe3O4@SiO2@PEI-NTDA was systematically investigated; the results confirmed that amine and anhydride groups were successfully covalently grafted onto the surface of Fe3O4@SiO2, which showed a homogenous core–shell structure with three layers of about 300 nm diameter (Fe3O4 core: 200 nm, nSiO2 layer: 20 nm, and PEI-NTDA layer: 20 nm). The adsorption performance of Fe3O4@SiO2@PEI-NTDA NPs was evaluated for single Pb2+ and coexisting Cd2+, Ni2+, Cu2+, and Zn2+ ions in an aqueous solution in a batch system. The amine and anhydride groups may have a synergistic effect on Pb2+ removal through electrostatic interactions and chelation; Fe3O4@SiO2@PEI-NTDA NPs exhibited preferable removal of Pb2+ with maximum adsorption capacity of 285.3 mg g−1 for Pb2+ at a solution pH of 6.0, adsorbent dosage of 0.5 g L−1, initial Pb2+ concentration of 200 mg L−1 and contact time of 3 h. The adsorption mechanism conformed well to the Langmuir isotherm model, and the adsorption kinetic data were found to fit the pseudo-second order model. Fe3O4@SiO2@PEI-NTDA NPs could be recovered easily from their dispersion by an external magnetic field and demonstrated good recyclability and reusability for at least 6 cycles with a high adsorption capacity above 204.5 mg g−1. The magnetic adsorbents showed high stability with a weight loss below 0.65% in the acid leaching treatment by 2 M HCl solution for 144 h. This study indicates that Fe3O4@SiO2@PEI-NTDA NPs are new promising adsorbents for the effective removal of Pb2+ in wastewater treatment.
1. Introduction
The discharge of wastewaters from various industrial activities, releasing many heavy metal contaminants, such as Cd2+, Ni2+, Cu2+ and Pb2+, has become one of the major environmental concerns in recent years.1 Heavy metal contaminants in such effluents are non-biodegradable and accumulate easily in living organisms through the food chain. Most heavy metals are known to be toxic; they may cause great damage to tissues and organs and even lead to dysmorphia and cancer even at low concentrations,2 posing a serious threat to human health, animals, plants and urban ecosystems. Among these heavy metals, Pb2+ is recognized as one of the most toxic elements; it can damage the kidney, liver, central nervous system, and especially the brain.3 Therefore, it is extremely essential to eliminate lead ions from waste water prior to its disposal. The maximum permissible levels of lead in drinking water have been established, including 0.010 mg L−1 by the EU and 0.015 mg L−1 by the US EPA, to protect natural water bodies from being contaminated by effluents containing such a heavy metal.4
Several methods have been developed to eliminate heavy metal ion contaminants from their wastewaters including reverse osmosis, co-precipitation,5 ion exchange,6 coagulation–flocculation,7 membrane processes,8 chemical oxidation,9 biological processes,10 chemical treatment,11 and adsorption.12 However, all these methods have their own limitations, such as time-consuming procedures, requirement for expensive equipment, and/or continuous need of chemical replenishment. Among these technologies mentioned above, adsorption has been considered to be one of the most common methods for the removal of heavy metal ions because of its ease of operation and high effectiveness. The key subject in adsorption technologies is to develop an efficient adsorbent with a rapid, selective, cost-effective and highly effective adsorption process; this has received intensive research attention. Thus, a variety of materials, such as activated carbons,13 zeolites,14 and biomaterials,15 have been investigated as adsorbents for heavy metal ion removal from wastewater. However, these adsorbents generally need to be separated from the water treatment system after the adsorption process by methods such as centrifugation or filtration. These inconvenient separation procedures prevent their large-scale applications in water treatment.
Hence, magnetic adsorbent materials, especially Fe3O4, have attracted wide interest in environmental remediation programs due to their easy and cost-effective separation processes only by using an external magnetic field and also because of their biocompatibility. However, naked Fe3O4 NPs, possessing high surface energy, tend to form aggregations quickly; this eliminates their adsorption properties and magnetic efficiency. Additionally, naked Fe3O4 is known to be prone to oxidation and is highly susceptible to leaching under acidic conditions. To stabilize and modify magnetic Fe3O4 particles and further improve their adsorption properties, composite materials have been fabricated in core–shell structures with polymers, silica-containing organic materials or other materials as a shell, such as Fe3O4@MOx (M = Si, Mn, Ti, and Al)16–18 and Fe3O4@polymer.19 One of the most important methods is the introduction of a dense SiO2 layer on the surface of magnetite Fe3O4 particles, which is very stable in acidic conditions and is inert to redox reactions; thus, it can effectively protect the inner magnetite cores from leaching in an acidic medium in practical applications.20 Additionally, SiO2 having abundant hydroxyl groups on its surface can be further modified by other functional groups to improve its properties. Recently, various functional groups have been anchored to the surface of Fe3O4@SiO2 composites to improve their adsorption properties, and these examples include EDTA-modified Fe3O4@SiO2,21 dimercaptosuccinic acid-coated Fe3O4@SiO2,22 and (3-aminopropyl)trimethoxysilane-modified Fe3O4@SiO2.23 Polyethylenimine (PEI), a hydrophilic polymer, has also been frequently used to improve the adsorption properties of materials towards metal ions because of the abundant functional amine groups in its structure.24 PEI has usually been grafted to other matrices such as insoluble polymers,25 biomass,26 and cellulose27 to prevent its dissociation from the surface of the matrices during adsorption operations; it has also been used to construct magnetic hybrid nanomaterials for environmental applications in heavy metal ion removal.28–30
In this work, to synthesize a magnetically separable adsorbent with high stability and adsorption capacity, a new type of magnetic silica material with amine and anhydride-functionalized layers was fabricated by covalently grafting a PEI polymer, followed by anhydride functionalization with 1,4,5,8-naphthalenetetracarboxylic-dianhydride (NTDA) onto the surface of Fe3O4@SiO2, as shown in Scheme 1. The PEI-NTDA layer on Fe3O4@SiO2 not only endowed the final magnetic adsorbents with hydrophilicity, reducing nonspecific adsorption during wastewater treatments, but also provided numerous amine and anhydride groups, which may synergistically coordinate or chelate with heavy metal ions. All these characteristics were expected to endow the final adsorbents with high adsorption capacity of heavy metal ions in wastewater treatments. The properties of the as-prepared Fe3O4@SiO2@PEI-NTDA adsorbent, including its stability in acid, magnetic performance, reusability, adsorption capacity and adsorption mechanism on Pb2+, were systematically evaluated in this work.
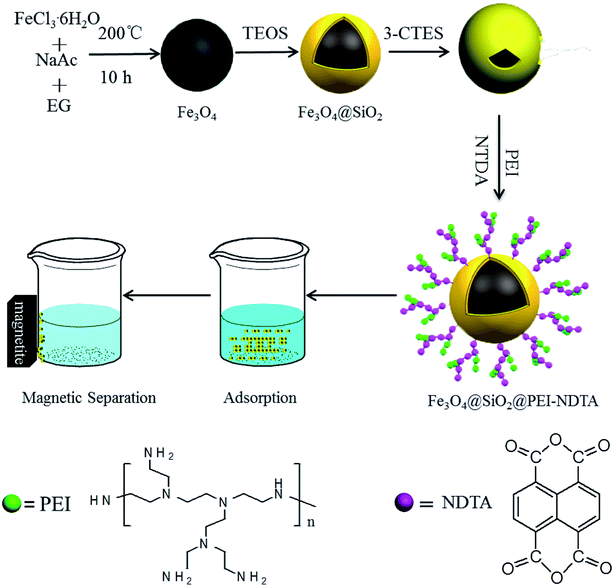 |
| Scheme 1 The diagram of the synthesis process of Fe3O4@SiO2@PEI-NTDA NPs and their application in Pb2+ removal. | |
2. Experimental
2.1. Chemicals
The chemicals used in this work were all of analytical grade and were used without any purification treatment. Ferric chloride hexahydrate (FeCl3·6H2O, Tianjin Fengchuan Chemical Reagent Science and Technology Co., Ltd., China), anhydrous sodium acetate (NaAc) and ethylene glycol (EG, both from Aladdin Reagent Co. Ltd., Shanghai, China) were used to prepare the magnetic Fe3O4 nanoparticles. Tetraethylorthosilicate (TEOS), ethanol, and ammonium hydroxide (25% w/w) were all purchased from Aladdin and used to prepare SiO2 on the surface of Fe3O4. Methane sulfonic acid (10%, Merck Chemical Technology Co., Ltd., Shanghai, China), 3-chloropropyltrimethoxysilane (Hua Kai Resin Co., Ltd.) and branched polyethyleneimine (PEI, molecular weight 10
000, 99%, Shanghai Hansi Chemical Industry Co., Ltd.) were used to graft PEI onto the surface of Fe3O4@SiO2. 1,4,5,8-Naphthalenetetracarboxylic-dianhydride (NTDA), N-methyl kelopyrrolidide (NMP) and methane sulfonic acid were purchased from Shanghai Macklin Biochemical Co., Ltd. and were used to functionalize Fe3O4@SiO2@PEI. The reagents HCl, HNO3, and NaOH were purchased from Tianjin Fengchuan. All solutions of Pb2+, Cd2+, Ni2+, Cu2+ and Zn2+ (Merck Chemical Technology Co., Ltd., Shanghai, China) were prepared using doubly distilled water.
2.2. Apparatus
X-ray diffraction (XRD) patterns were acquired on a Bruker D8 Advance diffractometer using Cu Kα radiation in the angular range from 5° to 90° to analyze the crystal structures of the samples. Scanning electron microscopy (SEM) (JSM-6490LV) and field emission scanning electron microscopy (FESEM) (JEOL, JSM-7001F) were used to observe the surface structures of the samples. The core–shell structures and sizes of the samples were confirmed by high-resolution transmission electron microscopy (HRTEM) (JEOL, JEM-2100). Infrared absorption spectra were collected using a Fourier transform infrared (FT-IR) spectrometer (NICOLET 380) to analyze the compositions of the samples. A thermogravimetric analyzer (TGA, Diamond, Japan) was used to collect the weight loss curves of the samples at a heating rate of 10 °C min−1 to 1000 °C under an N2 flow of 200 mL min−1. Atomic absorption spectroscopy (AAS) (ContrAA-700) was used to determine the metal ion concentrations in the solutions. A high field vibrating sample magnetometer (LS7307-9309) was used to evaluate the magnetic properties of the samples.
2.3. Synthesis of Fe3O4 NPs
The magnetic Fe3O4 NPs were synthesized by the conventional solvothermal method.31 In a typical procedure, 1.80 g FeCl3·6H2O and 1.09 g NaAc were added to 30 mL EG with vigorous stirring for 30 min; then, the mixture was heated at 200 °C in a sealed Teflon-lined stainless-steel autoclave with a volume of 50 mL for 12 h and naturally cooled to room temperature. The product was magnetically separated and washed with ethanol and deionized water, sequentially. The Fe3O4 NPs were dried under vacuum at 60 °C for 12 h.
2.4. Synthesis of Fe3O4@SiO2 NPs
The Fe3O4@SiO2 NPs were fabricated as reported previously.32 Before coating Fe3O4 NPs with SiO2, freshly prepared 100 mg Fe3O4 particles were dispersed in 100 mL HCl aqueous solution with a concentration of 0.1 mol L−1; this mixture was placed in an ultrasonic bath for 15 min to form –OH groups on the outer surface of Fe3O4. Then, the hydroxylated Fe3O4 samples were washed thoroughly with deionized water to pH ∼ 7.0 and re-dispersed in an ethanol/H2O (80 mL/20 mL) mixture. NH3·H2O (1.0 mL) was added to the above dispersion; then, 0.5 mL TEOS was introduced. The mixture was placed in an ultrasonic bath for 10 min, followed by mechanical stirring for 6 h at room temperature. Then, the synthesized Fe3O4@SiO2 products were magnetically separated and washed with deionized water and ethanol several times, followed by vacuum drying at 60 °C for 12 h.
2.5. Synthesis of Fe3O4@SiO2@PEI-NTDA
In a representative procedure, Fe3O4@SiO2 NPs were treated with an aqueous solution of methane sulfonic acid (10%) as an activation reagent. The activated Fe3O4@SiO2 NPs and 0.1 mL 3-chloropropyltrimethoxysilane were mixed in 30 mL xylene. The mixture was added to a Teflon-lined stainless-steel autoclave with a volume of 50 mL in an oven and maintained at 130 °C for 24 h. After that, the autoclave was cooled to room temperature and opened; then, PEI solution (10%) was added. The autoclave was then resealed, and the reaction was continued at 130 °C for another 24 h. The crude Fe3O4@SiO2@PEI samples were separated and washed thoroughly with deionized water and ethanol. Vacuum drying was carried out at 60 °C for 12 h. Also, 50 mg of Fe3O4@SiO2@PEI NPs was suspended in 150 mL N-methyl kelopyrrolidide under vigorous mechanical stirring, followed by adding 0.134 g NTDA. The mixture was further maintained at 60 °C under stirring for 6 h. The products denoted as Fe3O4@SiO2@PEI-NTDA NPs were obtained by magnetic separation, followed by washing and vacuum drying at 60 °C for 12 h.
2.6. Adsorption experiments
The adsorption properties of the Fe3O4@SiO2@PEI-NTDA samples were estimated by batch experiments. In a typical process, a certain amount of Fe3O4@SiO2@PEI-NTDA was added to a plastic tube containing 50 mL aqueous solution at room temperature with different initial concentrations of heavy metal ions (Pb2+, Cd2+, Ni2+, Cu2+, and Zn2+), pH values, adsorption times, adsorbent dosages and coexisting pH values and adsorbent dosages. The tube was sealed and left for a certain contact time. After the adsorption experiments, the adsorbents were magnetically separated and the metal concentration in the residual solution was tested to determine the adsorption capacity of the adsorbent according to the following equation: |
 | (1) |
Here, Q stands for the adsorption capacity of the adsorbent (mg g−1), Ci stands for the initial metal ion concentration in the solution (mg L−1), Ct refers to the residual metal ion concentration (mg L−1), M stands for the mass (g) of the adsorbent, and V stands for the volume of the solution (L).
In order to evaluate the selectivity of the prepared Fe3O4@SiO2@PEI-NTDA adsorbent for Pb2+ over other metal ions, namely, Cd2+, Ni2+, Cu2+, and Zn2+, adsorption experiments were conducted with a contact time of 3 h by adding 25 mg Fe3O4@SiO2@PEI-NTDA NPs to 50 mL solution (pH = 6.0) containing Pb2+, Cd2+, Ni2+, Cu2+, and Zn2+, each of which had an initial concentration of 200 mg L−1.
2.7. Leaching test
The material stability of Fe3O4@SiO2@PEI-NTDA NPs was evaluated by the ratio of the mass of Fe3O4 leached by HCl solution to the total mass of the Fe3O4@SiO2@PEI-NTDA adsorbent. The specific process is as follows: 10 mg of the Fe3O4@SiO2@PEI-NTDA adsorbent was immersed in 50 mL of HCl solution for a certain interval; the Fe3O4@SiO2@PEI-NTDA NPs were removed magnetically from the solution, and the residual solution was used to test the Fe ion concentration by AAS technology. The Fe ions in the solution, which were considered to have leached from Fe3O4@SiO2@PEI-NTDA NPs by acid, were concerted into the mass of Fe3O4. The percentage was calculated by the mass of leached Fe3O4 to the total mass of Fe3O4@SiO2@PEI-NTDA NPs to evaluate their stability under acidic conditions.
2.8. Desorption and recycling studies of Fe3O4@SiO2@PEI-NTDA
Adsorption–desorption cycling experiments were carried out to investigate the reusability of the adsorbents. For one adsorption–desorption cycling procedure, 25 mg Fe3O4@SiO2@PEI-NTDA sorbent was dispersed in 50 mL of aqueous solution with an initial Pb2+ concentration of 100 mg L−1 at an initial pH value of 6.0. After a contact time of 3 hours, the adsorbents were separated magnetically and the residual solution was subjected to Pb2+ measurements to calculate the adsorption capacity. The Pb2+-loaded sorbent was followed by the desorption experiment, in which the Pb2+-loaded sorbent was added to 30 mL of 0.5 M HCl solution under mechanical stirring at 350 rpm and at room temperature for 1 h. The Fe3O4@SiO2@PEI-NTDA NPs were obtained by magnetic separation and washed to neutral pH with deionized water to prepare for the next adsorption–desorption cycling experiment. The adsorption–desorption cycling experiments were carried out for six times.
3. Results and discussion
3.1. The synthesis and structure of Fe3O4@SiO2@PEI-NTDA NPs
Fe3O4 NPs, serving as the magnetic core of the adsorbent, were fabricated by a conventional solvothermal method. Fe3O4 NPs were coated with an SiO2 layer by a conventional sol–gel method by hydrolyzing and condensing TEOS. Fe3O4@SiO2 NPs were modified with a common silane coupling agent, 3-chloropropyltrimethoxysilane (CPTES), the Cl end groups of which can be used to react with the –NH– groups of a PEI polymer to form covalent grafting of branched PEI on the surface of Fe3O4@SiO2. Afterward, the synthesized Fe3O4@SiO2@PEI particles were further anhydride-functionalized with NTDA by the reaction between the –NH– groups and the anhydride groups of NTDA to construct Fe3O4@SiO2@PEI-NTDA NPs.
The surface structures of the synthesized samples were observed by SEM. As shown in Fig. 1a, the prepared Fe3O4 samples are in good spherical shape and have a rough surface and a uniform particle size with diameter of about 200 nm. The diameter of Fe3O4 NPs can also be verified by the TEM images in Fig. 1d, where it ranges from 180 to 220 nm. As shown in Fig. 1b, Fe3O4@SiO2 NPs have relatively smooth surfaces and large diameters compared to uncoated Fe3O4 NPs. Moreover, the TEM images in Fig. 1e show that the prepared Fe3O4@SiO2 NPs have well-defined core–shell structures with homogeneous dense SiO2 shells with thickness of approximately 20 nm. These results indicate that the SiO2 layers were formed successfully on the surface of Fe3O4 spherical particles. This dense SiO2 layer was expected to serve as a protective layer, protecting the magnetic Fe3O4 core from corrosion and oxidation in the adsorption process. The SEM images in Fig. 1c show that the Fe3O4@SiO2@PEI-NTDA samples have homogeneous spherical particles, which are larger than those of Fe3O4@SiO2, and this can also be verified by the TEM images. As shown in Fig. 1f, Fe3O4@SiO2@PEI-NTDA NPs with diameters ranging from 200 to 300 nm have a core–shell structure with a dark core, which is assigned to Fe3O4. The light-transparent shell with irregular edges has thickness of about 30 nm, which is greater than that of the SiO2 shell, indicating the successful grafting of PEI and successive modification with NTDA on Fe3O4@SiO2. The successful grafting of PEI/NTDA was also confirmed by the EDXS measurements. As shown in Fig. 1g, the appearance of C, N and O signals can be attributed to the –NH– and –COOH groups on PEI and NTDA. This outer PEI-NTDA layer is hydrophilic and contains numerous functional groups, which are expected to improve the adsorption capacity of the adsorbent by chelating with heavy metal ions for wastewater treatment.
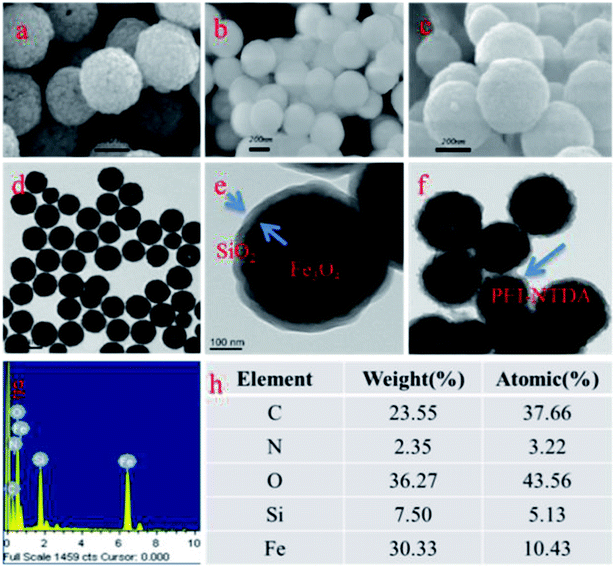 |
| Fig. 1 SEM (a–c) and TEM (d–f) images of Fe3O4 NPs, Fe3O4@SiO2, and Fe3O4@SiO2@PEI-NTDA NPs, respectively; EDXS analysis (g and h) of Fe3O4@SiO2@PEI-NTDA. | |
Fe3O4 in the prepared composites has a face-centered cubic structure,33,34 which is consistent with the JCPDS card (file no. 19-0629). Additionally, the positions of these characteristic peaks of Fe3O4 did not change in the XRD patterns of Fe3O4@SiO2 and Fe3O4@SiO2@PEI-NTDA, indicating that the binding process of the introduction of SiO2 and the NTDA-functionalized PEI layer did not affect the crystal structure of Fe3O4. However, the characteristic diffraction peaks of Fe3O4 became relatively weak in the latter functionalized composites Fe3O4@SiO2 and Fe3O4@SiO2@PEI-NTDA, which may be due to the coating of amorphous SiO2 and PEI.
The FT-IR spectra of all samples were obtained in the range from 4000−1 to 400 cm−1 to investigate the surface functional groups and confirm the chemical compositions of the modified NPs, as depicted in Fig. 2b. For pure Fe3O4 NPs, an obvious adsorption peak appears at 584 cm−1, which is attributed to the characteristic Fe–O bond vibration from Fe3O4. After the sol–gel reaction with TEOS in the presence of Fe3O4, a new strong adsorption peak appeared at around 1100 cm−1, which was attributed to the stretching vibrations of Si–O–Si and Si–O–H; this indicates the presence and successful coating of the SiO2 layer on the samples of Fe3O4@SiO2 and their successive samples of Fe3O4@SiO2@PEI and Fe3O4@SiO2@PEI-NTDA.35 It can be observed in the FT-IR spectra of Fe3O4 and Fe3O4@SiO2 NPs that two peaks for the stretching vibration of hydroxyl (–OH) groups appear at 1609 cm−1 and 3440 cm−1, indicating that there are hydroxyl groups on the surfaces of Fe3O4 and Fe3O4@SiO2 NPs. After the graft reaction with PEI, two new peaks at 2919 and 2840 cm−1 may be assigned to aliphatic C–H bonds; the bands at 1450 and 1320 cm−1 may belong to the stretching vibrations of C–H and C–N bonds, respectively.28 The characteristic adsorption peaks of primary amines (–NH) appear at 1656 cm−1 and 1612 cm−1, and a broader adsorption peak in the region of 3300–3600 cm−1 may be ascribed to the overlapping of the N–H vibration of PEI with the O–H stretching of SiO2. All these results indicate the successful grafting of PEI. After reaction with NTDA, a new strong peak appears at 1680–1780 cm−1, which may be attributed to the symmetric and asymmetric stretchings of –C
O bonds;36 also, the peak at 1320 cm−1 (C–N asymmetric) indicates the successful functionalization of anhydride on the surface of Fe3O4@SiO2.
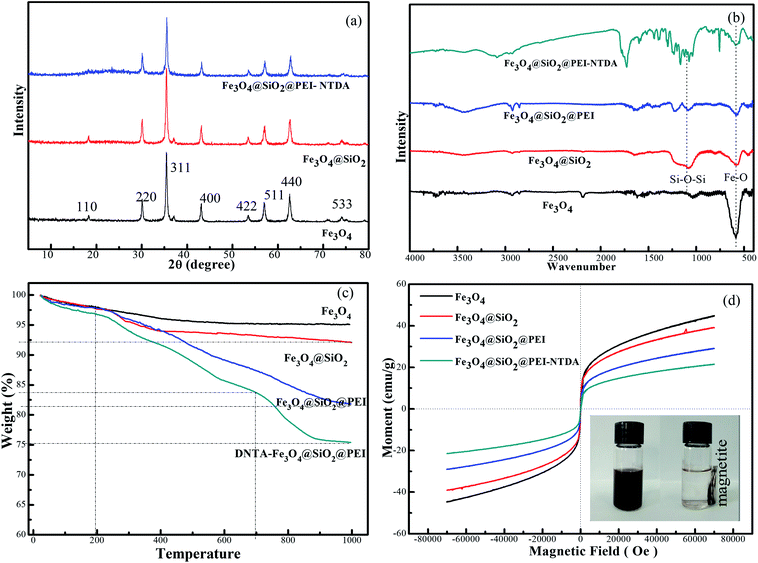 |
| Fig. 2 XRD patterns (a), FT-IR spectra (b), TGA curves (c) and magnetization curves (d) of the samples. | |
TGA analyses of Fe3O4, Fe3O4@SiO2, Fe3O4@SiO2@PEI and Fe3O4@SiO2@PEI-NTDA were also performed (Fig. 2c). It can be seen that Fe3O4 NPs displayed a weight loss of 4.83% in the range of 25–400 °C and remained stable above 400 °C. The obvious weight loss of Fe3O4@SiO2 above 200 °C compared to that for pure Fe3O4 can be attributed to the structure decomposition of SiO2. Also, the TGA curve of Fe3O4@SiO2@PEI shows a dramatic decline above 400 °C, indicating the pyrolysis of PEI. The TGA curve of Fe3O4@SiO2@PEI-NTDA displays one more step than that of Fe3O4@SiO2@PEI above 700 °C, which can be attributed to NTDA. The weight losses of Fe3O4@SiO2, Fe3O4@SiO2@PEI and Fe3O4@SiO2@PEI-NTDA at 1000 °C were 7.94%, 18.18% and 24.61%, respectively. These results also verified that PEI was grafted successfully on the surface of Fe3O4@SiO2 and NTDA was successfully anchored on PEI.
The magnetization curves of the samples were all tested at room temperature (Fig. 2d). It can be observed that there are no hysteresis loops in the magnetization curves for all of the four samples. All of the samples are superparamagnetic. The magnetic saturation (Ms) value was found to be 44.7 emu g−1 for Fe3O4. Along with the modification processes on Fe3O4, the nonmagnetic layer sizes increased due to silica coating, PEI grafting and NTDA modification, due to which the Ms values decreased accordingly to 39.2 emu g−1 for Fe3O4@SiO2, to 29.4 emu g−1 for Fe3O4@SiO2@PEI, and to 21.6 emu g−1 for Fe3O4@SiO2@PEI-NTDA.37 Nevertheless, the value of Fe3O4@SiO2@PEI-NTDA NPs was still high enough, due to which they could be completely separated from their aqueous dispersion by a magnet near the glass vial, affording a clear and transparent solution (inset photographs in Fig. 2d). These results indicate that Fe3O4@SiO2@PEI-NTDA NPs are ready for use as magnetically separable adsorbents for application in water treatment.
3.2. Adsorption properties of Fe3O4@SiO2@PEI-NTDA for Pb2+ heavy metal ion removal
In order to illustrate the advantages of Fe3O4@SiO2@PEI-NTDA, batch experiments were carried out to test its adsorption properties for Pb2+ heavy metal ion removal at a temperature of 298 K, solution pH value of 6.0, sorbent dosage of 0.5 g L−1, and contact time of 3 h. The results compared with those of Fe3O4@SiO2 and Fe3O4@SiO2@PEI are presented in Fig. 3. It can be observed that the adsorption capacity for Pb2+ removal is very low on Fe3O4@SiO2 NPs, becomes higher on Fe3O4@SiO2@PEI and reaches the highest value on Fe3O4@SiO2@PEI-NTDA NPs; these results can be ascribed to the binding capability of the amino groups and functionalized carboxyl groups in the outer PEI-NTDA layer. This indicates that the modification methods employed in this work were effective to improve the adsorption properties of the magnetic sorbent Fe3O4.
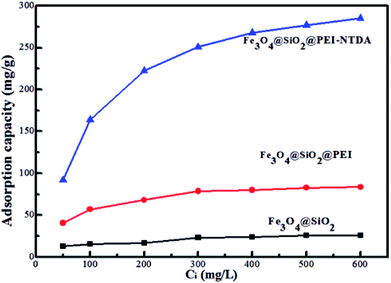 |
| Fig. 3 Adsorption capabilities of Fe3O4@SiO2, Fe3O4@SiO2@PEI, and Fe3O4@SiO2@PEI-NTDA for removal of Pb2+ from water. Adsorption conditions: sorbent dosage of 0.5 g L−1, solution pH value of 6.0, equilibrium time of 3 h, temperature of 298 K. | |
Then, the influencing factors, including pH value, adsorbent dosage, initial Pb2+ concentration, contact time, and coexisting heavy ions, were optimized for the Pb2+ adsorption performance of Fe3O4@SiO2@PEI-NTDA NPs. It has been reported that the adsorbent dosage has a significant effect on the interactions between the metal ions in the solution and the adsorption sites in the adsorbents38 and further influences the adsorption capacity. Therefore, the Fe3O4@SiO2@PEI-NTDA dosage was optimized by batch adsorption experiments by varying the adsorbent doses from 0.125 g L−1 to 1.0 g L−1. The changes in the adsorption capacities with the adsorbent dosage are presented in Fig. 4a. The results showed that the adsorption capacity of Fe3O4@SiO2@PEI-NTDA increased greatly with increasing dosage from 0.125 g L−1; above the dosage of 0.5 g L−1, continued increase in the dosage to 1.0 g L−1 resulted in a sharp decrease in the capacity. This can be explained as follows: when maintaining a constant initial Pb2+ concentration in the solution, the primary increase in the dosage may provide more available adsorption sites, leading to higher removal efficiency and higher adsorption capacity. When the dosage of the adsorbents matched the total quantity of Pb2+ in the solution, the adsorption capacity reached the highest value. Afterwards, further increase in the dosage may result in excessive adsorption sites and aggregation of the adsorbents, reducing the effective active adsorption sites for the removal of Pb2+ and hence the adsorption capacity.21 It can be concluded from Fig. 4a that Fe3O4@SiO2@PEI-NTDA NPs exhibit a higher adsorption capacity with a dosage of about 0.5 g L−1, and the following adsorption experiments were conducted at this optimum dosage.
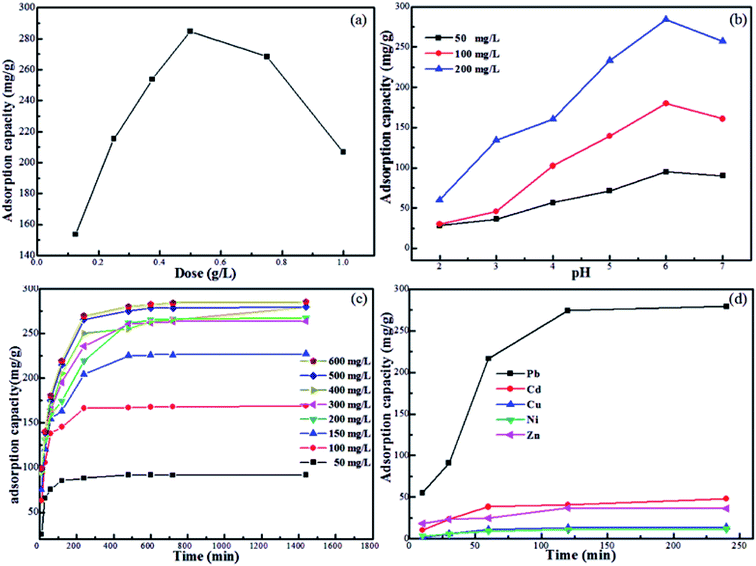 |
| Fig. 4 Effects of (a) dosage, (b) pH, (c) contact time and initial Pb2+ concentration, and (d) coexisting heavy ions on Pb2+ adsorption of Fe3O4@SiO2@PEI-NTDA NPs. | |
It is very important to select an appropriate pH for the adsorption process of Pb2+ removal because the pH value has significant influences on the surface charges of the sorbents in aqueous solutions, the ionization degree of the heavy metal ions and therefore the speciation of the adsorbate.39 The optimized pH of Pb2+ adsorption was investigated (Fig. 4b). At low pH values below 6.0, it can be observed that the adsorption capacities of Fe3O4@SiO2@PEI-NTDA increased obviously on increasing the solution pH and then decreased when the pH further increased from 6.0. At low pH values, the concentration of hydrogen (H+) or hydronium (H3O+) ions increased with the decrease in the pH value, and competitive adsorption existed between the hydrogen (H+) or hydronium (H3O+) ions and Pb2+ on the surface of the Fe3O4@SiO2@PEI-NTDA adsorbents;40 thus, the metal ions were inaccessible. On increasing pH, the competitive effect of H3O+ decreased, due to which the uptake of Pb2+ occurred more easily on the free binding sites. In addition, the speciation of lead is also dependent on the pH value. At pH values of 2.0–6.0, lead species exist exclusively as Pb2+ ions in the solution; above pH of 6.0, they will undergo hydrolysis to Pb(OH)+ and the insoluble precipitate Pb(OH)2.41 The predominant adsorbing forms of lead are Pb2+ ions, which may interact with Fe3O4@SiO2@PEI-NTDA via the carboxyl, hydroxyl and amino functional groups by a complexation mechanism. It can be observed that the maximum removal (285.3 mg g−1) is achieved at about pH = 6.0. Therefore, further adsorption experiments were all carried out with an initial pH value of 6.0.
Adsorption equilibrium time experiments were conducted at a fixed adsorbent dosage of 0.5 g L−1 and an initial pH value of 6.0 for Pb2+ ion removal while varying the initial Pb2+ concentration from 50 to 600 mg L−1. It can be observed from Fig. 4c that the adsorption of Pb2+ ions increases rapidly with contact time for the first 100 min at the beginning of the adsorption; this can be attributed to the abundant active adsorption sites that are available for easily interacting with Pb2+ ions. After that, the adsorption rate tended to become almost constant and finally, sorption equilibrium for the adsorption of Pb2+ ions was established in a dynamic balance between the concentration of Pb2+ ions in the solution and the adsorption sites on the adsorbent.
The adsorption rate and capacity of Fe3O4@SiO2@PEI-NTDA increased on increasing the initial metal ion concentration of the solution. This can be explained by the fact that a higher initial Pb2+ ion concentration will provide a larger driving force between the solid–liquid interfaces to overcome the resistance during the mass transfer process, resulting in a higher adsorption rate. For all the initial Pb2+ ion concentrations employed in this work, an adsorption time of 200 min was sufficient to reach adsorption equilibrium. The competitive adsorption performance on Fe3O4@SiO2@PEI-NTDA was investigated in the presence of coexisting Pb2+, Cd2+, Ni2+, Cu2+, and Zn2+ ions. As presented in Fig. 4d, Fe3O4@SiO2@PEI-NTDA NPs exhibit much higher removal efficiency for Pb2+ than that for other metal ions, which is in the following order: Pb2+ > Cd2+ > Zn2+ > Cu2+ > Ni2+. According to the Pearson hard–soft acid–base theory, Pb2+ can be classified as a borderline acid and prefers bonding to ligands containing N donor atoms. Fe3O4@SiO2@PEI-NTDA with a large number of N atoms in the polymer resin may coordinate to Pb2+ rather than to Cd2+, which is classified as a soft acid.
In order to determine the rate-controlling step of the adsorption process, including mass transport and chemical reactions, the data of the adsorption experiments were analyzed by the pseudo-first order42 and the pseudo-second order43 equations.
The pseudo-first order equation is expressed as follows:
|
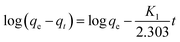 | (2) |
Here,
qt and
qe (mg g
−1) refer to the amounts of Pb
2+ adsorbed on the adsorbent at time
t and at equilibrium, respectively;
K1 (min
−1) refers to the rate constant. The plots of log(
qe −
qt)
versus t are presented in
Fig. 5a; from their slopes, the
K1 values are obtained and listed in
Table 1.
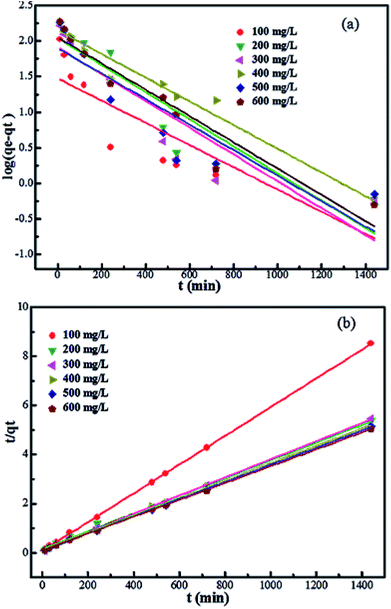 |
| Fig. 5 Pseudo-first order model (a) and pseudo-second order model (b) for the adsorption of Pb2+ on Fe3O4@SiO2@PEI-NTDA at dosage = 0.5 g L−1, pH = 6.0, Pb2+ concentration = 200 mg L−1, contact time = 3 h, and temperature = 298 K. | |
Table 1 Calculated pseudo-first-order and pseudo-second-order parameters for the adsorption of Pb2+
Pb2+ conc. (mg L−1) |
Pseudo-first-order |
Pseudo-second-order |
qe (cal) |
K1 (min−1) |
R2 |
qe (cal) |
K2 (g mg−1 min−1) |
R2 |
100 |
29.92 |
0.0035 |
0.710 |
171.2 |
0.00584 |
0.999 |
200 |
111.2 |
0.0043 |
0.839 |
277.7 |
0.00360 |
0.996 |
300 |
81.8 |
0.0043 |
0.798 |
271.0 |
0.00369 |
0.999 |
400 |
142.1 |
0.0038 |
0.964 |
284.9 |
0.00351 |
0.999 |
500 |
79.58 |
0.0041 |
0.788 |
286.5 |
0.00349 |
0.999 |
600 |
113.1 |
0.0042 |
0.909 |
292.3 |
0.00342 |
0.999 |
The pseudo-second order equation is given as follows:
|
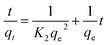 | (3) |
Here,
K2 (g (mg min)
−1) refers to the rate constant and
qt and
qe (mg g
−1) refer to the amounts of Pb
2+ adsorbed on the adsorbent at time
t and at equilibrium, respectively. Similarly,
K2 can be obtained from the plot of
t/
qt versus t (
Fig. 5b), and the results are listed in
Table 1.
From Fig. 5, we can see that the pseudo-second order model fits the experiment data well; this can be confirmed by the correlation coefficient (R2) values beyond 0.996, which are much higher than that of the pseudo-first order model (below 0.964). In addition, the values of the experimental adsorption capacity of the adsorbents are close to the calculated theoretical adsorption capacity (qe,cal) from the pseudo-second order model. These results indicate that chemical adsorption may be the rate-limiting step of the adsorption process of Pb2+ on Fe3O4@SiO2@PEI-NTDA.
For further understanding the adsorption mechanism of Pb2+ on Fe3O4@SiO2@PEI-NTDA NPs, the Langmuir44 and Freundlich45 models were also applied to analyse the experimental adsorption data, which were obtained with an adsorbent dosage of m/V = 0.5 g L−1 and at pH = 6.0 at three different temperatures of 298 K, 303 K, and 308 K.
The Langmuir model is expressed by the following equation:
|
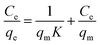 | (4) |
Here,
Ce (mg L
−1) refers to the metal ion concentration in the solution at equilibrium,
qe (mg g
−1) refers to the adsorption capacity on the sorbent at equilibrium,
qm (mg g
−1) refers to the monolayer adsorption capacity, and
K refers to a Langmuir constant related to the sorption energy.
The Freundlich model is applicable to the adsorption that occurs on heterogeneous surfaces, and it is presented as follows:
|
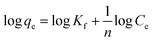 | (5) |
Here,
Kf (mg g
−1) refers to a Freundlich constant related to the adsorption capacity and 1/
n refers to an empirical parameter related to the adsorption intensity.
It can be observed from Fig. 6 that the adsorption isotherm data fit well with the Langmuir model than with the Freundlich model. The constant values and determination coefficients (R2) are presented in Table 2. There is a high correlation regression equation R2 value of 0.999 for the Langmuir model at each of the three experimental temperatures; it is far greater than that of the Freundlich model, which has a value of around 0.80. As we know, the Langmuir model is reasonable for a homogeneous adsorption process that takes place at the adsorbent surface, where there are no intermolecular interactions among the adsorbed molecules and all sites are identical and energetically equivalent for the adsorbate; the Freundlich model is applicable to adsorption that occurs on heterogeneous surfaces. These results reveal that the adsorption process of Pb2+ occurring on Fe3O4@SiO2@PEI-NTDA may be uniform monolayer surface adsorption. The functional groups on the surface of Fe3O4@SiO2@PEI-NTDA provide binding sites for Pb2+ to reach a high adsorption capacity. As shown in Table 3, the prepared Fe3O4@SiO2@PEI-NTDA NPs possess remarkable adsorption capacity for Pb2+ compared to previously reported magnetic adsorbents.
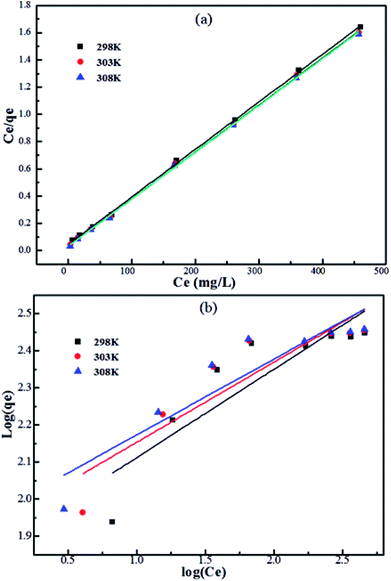 |
| Fig. 6 Langmuir (a) and Freundlich (b) simulations for the adsorption of Pb2+ on Fe3O4@SiO2@PEI-NTDA at dosage = 0.5 g L−1, pH = 6.0, Pb2+ concentration = 200 mg L−1, and contact time = 3 h at three different temperatures of 298 K, 303 K, and 308 K. | |
Table 2 Langmuir and Freundlich isotherm parameters for the adsorption of Pb2+ onto Fe3O4@SiO2@PEI-NTDA at different temperatures
Temp. (K) |
Langmuir |
Freundlich |
R2 |
K |
qm |
R2 |
n |
Kf |
298 |
0.999 |
0.0786 |
286.9 |
0.756 |
4.20 |
74.77 |
303 |
0.999 |
0.0998 |
289.9 |
0.809 |
4.63 |
86.48 |
308 |
0.999 |
0.1128 |
290.7 |
0.836 |
4.89 |
92.99 |
Table 3 Maximum capacities of Pb2+ on various magnetic adsorbents
Type of adsorbent |
Adsorption capacities (mg g−1) |
Ref. |
Pb2+ |
Cd2+ |
Polymer-modified Fe3O4 |
83.3 |
|
46 |
PAA@TSH-modified Fe3O4 |
188.7 |
107.5 |
47 |
Amino-functionalized Fe3O4@SiO2 |
76.6 |
22.5 |
48 |
PEI-functionalized mesoporous magnetic clusters |
216.3 |
|
49 |
PEI-bacterial cellulose bio-adsorbent |
141 |
|
50 |
Magnetic porous Fe3O4–MnO2 |
208.2 |
169.9 |
51 |
Biochar–MnFe2O4 |
154.94 |
127.83 |
52 |
T-β-CD-Fe3O4 |
105.38 |
|
53 |
NTA-silica gel |
76.22 |
53.14 |
54 |
Fe3O4@SiO2@PEI-NTDA |
285.3 |
48.2 |
This work |
3.3. Stability and cycling properties of Fe3O4@SiO2@PEI-NTDA for heavy metal ions of Pb2+
The material stability and regeneration of Fe3O4@SiO2@PEI-NTDA were also studied to evaluate the repeated availability of the adsorbent in water treatment for economic consideration. It can be seen from the above adsorption results that the adsorption of Pb2+ on Fe3O4@SiO2@PEI-NTDA is pH-dependent and that the optimum adsorption capacity occurs at a pH value of about 6.0. Additionally, in order to recover the adsorbent from the state of adsorption, a desorption process must be carried out by the treatment of the adsorbent with HCl solution. In these two cases, naked Fe3O4 may be subject to leaching from the adsorbents because of the acid media. Therefore, the material stability in acidic media is very important for the modified Fe3O4 adsorbent to evaluate its regeneration properties; this is carried out by soaking certain amounts of Fe3O4@SiO2@PEI-NTDA in HCl solution with various concentrations for certain times. The Fe ion concentration in the solution was tested and the Fe leaching percent was calculated, as shown in Fig. 7a. It can be seen that the Fe leaching percent increases on increasing the soaking time and on increasing the concentration of HCl in the solution and reaches a lower value of 0.65 wt% after 144 h treatment in 2 mol L−1 HCl solution, indicating high material stability of the prepared Fe3O4@SiO2@PEI-NTDA adsorbent in acid media. This can be attributed to the outer SiO2@PEI layer, which functions as an effective protecting shell that enables Fe3O4@SiO2@PEI-NTDA to be readily reused. Hence, adsorption–desorption experiments were carried out, and the adsorption capacity results of the recycling experiment are shown in Fig. 6b. It can be observed that the adsorption capacity decreased on increasing the recycling time and Fe3O4@SiO2@PEI-NTDA remained valid for Pb2+ removal for six times with a high adsorption capacity of 204.3 mg g−1; this indicates that Fe3O4@SiO2@PEI-NTDA is a promising adsorbent that can be applied repeatedly in water treatment for the removal of Pb2+.
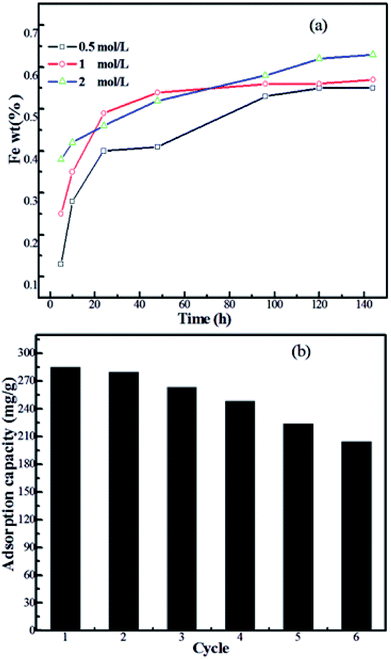 |
| Fig. 7 (a) The leaching percentages of Fe from Fe3O4@SiO2@PEI-NTDA in different HCl solutions; T = 298 K, dose = 0.5 g L−1. (b) Recycling of Fe3O4@SiO2@PEI-NTDA in the removal of Pb2+; T = 298 K, dose = 0.5 g L−1, pH = 6.0. | |
4. Conclusion
A novel magnetically separable adsorbent was successfully constructed with a core–shell structure comprising a magnetic Fe3O4 core, an outer protective SiO2 layer and an outermost hydrophilic layer of PEI-NTDA with abundant functionalized groups including amino groups, hydroxyl and carboxyl groups, which could bind with heavy metal ions. This core–shell structure endowed Fe3O4@SiO2@PEI-NTDA with many good properties: the composites are magnetically separable and sustainable in acid media with a low weight loss; also, Fe3O4@SiO2@PEI-NTDA NPs have a high primary adsorption capacity of Pb2+ and the adsorbents can be recovered by HCl treatment for recycling, maintaining high recycling adsorption capacity of 204.3 mg g−1 for six adsorption–regeneration cycles. These unique properties make Fe3O4@SiO2@PEI-NTDA NPs promising effective adsorbents for the removal of Pb2+ metal ions in water treatment.
Conflicts of interest
There are no conflicts to declare.
Acknowledgements
This work was supported by grants from the National Natural Science Foundation of China (No. 21401170, 21271160, 91022011, 21371092), National Basic Research Program of China (2010CB923303), Major Projects in Henan Province of China (No. 181100310300) and Research Fund for the Doctoral Program of Zhengzhou University of Light Industry (No. 2013BSJJ021).
References
- F. Fu and Q. Wang, Removal of heavy metal ions from wastewaters: a review, J. Environ. Manage., 2011, 92(3), 407–418 CrossRef CAS PubMed.
- F. E. Peer, N. Bahramifar and H. Younesi, Removal of Cd (II), Pb (II) and Cu (II) ions from aqueous solution by polyamidoamine dendrimer grafted magnetic graphene oxide nanosheets, J. Taiwan Inst. Chem. Eng., 2018, 87, 225–240 CrossRef.
- R. P. Medina, E. T. Nadres, F. C. Ballesteros and D. F. Rodrigues, Incorporation of graphene oxide into a chitosan-poly(acrylic acid) porous polymer nanocomposite for enhanced lead adsorption, Environ. Sci.: Nano, 2016, 3, 638–646 RSC.
- K. Li and X. Wang, Adsorptive removal of Pb(II) by activated carbon prepared from Spartina alterniflora: equilibrium, kinetics and thermodynamics, Bioresour. Technol., 2009, 99(11), 2810–2815 CrossRef PubMed.
- D. Marani, G. Macchi and M. Pagano, Lead precipitaiton in the presence of sulphate and carbonate, testing the thermodynamic predictions, Water Res., 1995, 29(4), 1085–1092 CrossRef CAS.
- L. C. Lin and R. S. Juang, Ion-exchange equilibria of Cu(II) and Zn(II) from aqueous solutions with Chelex 100 and Amberlite IRC 748 resins, Chem. Eng. J., 2005, 112(1), 211–218 CrossRef CAS.
- J. S. George, A. Ramos and H. J. Shipley, Tanning facility wastewater treatment: Analysis of physical–chemical and reverse osmosis methods, J. Environ. Chem. Eng., 2015, 3(2), 969–976 CrossRef CAS.
- D. Sribudda, T. Wannachod and P. Ramakul, Separation of mercury and arsenic from produced water via hollow fiber contactor: Kinetic and mass transfer analysis, Korean J. Chem. Eng., 2016, 33(1), 1–10 CrossRef.
- K. Dutta, S. Mukhopadhyay, S. Bhattacharjee and B. Chaudhuri, Chemical oxidation of methylene blue using a Fenton-like reaction, J. Hazard. Mater., 2001, 84(1), 57–71 CrossRef CAS PubMed.
- L. Luo, Y. Xie, Q. Liu, Z. Tan and X. Li, Isolation of a biological iron sulfide composites-producing strain and its application in emergency treatment of heavy metal wastewater, Chin. J. Appl. Environ. Biol., 2012, 18(01), 115–121 CrossRef CAS.
- V. K. Gupta, I. Ali, T. A. Saleh, A. Nayak and S. Agarwal, Chemical treatment technologies for waste-water recycling—an overview, RSC Adv., 2012, 2, 6380–6388 RSC.
- J. S. Pang, A. H. Deng, L. B. Mao, J. Chen, X. J. Peng and J. Zhu, Adsorption of heavy metal ions with magnetic carbon-coated iron nanoparitcles, Adv. Mater. Res., 2012, 490–495, 3049–3053 CAS.
- G. B. Baur, I. Yuranov and L. Kiwi-Minsker, Activated carbon fibers modified by metal oxide as effective structured adsorbents for acetaldehyde, Catal. Today, 2015, 249, 252–258 CrossRef CAS.
- Q. Meng, H. Chen, Z. Lin and J. Sun, Zeolite A synthesized from alkaline assisted pre-activated halloysite for efficient heavy metal removal in polluted river water and industrial wastewater, J. Environ. Sci., 2017, 56, 254–262 CrossRef PubMed.
- M. N. Sepehr, M. Zarrabi, A. Amrane and M. R. Samarghandi, Removal of Cr (III) from model solutions and a real effluent by Phanerochaete chrysosporium isolated living microorganism: equilibrium and kinetics, Desalin. Water Treat., 2013, 51(28–30), 5627–5637 CrossRef CAS.
- S. Dib, M. Boufatit, S. Chelouaou, F. Sadi-Hassaine, J. Croissant, J. Long, L. Raehm, C. Charnay and J.-O. Durand, Versatile heavy metals removal via magnetic mesoporous nanocontainers, RSC Adv., 2014, 4, 24838–24841 RSC.
- T. S. Munonde, N. W. Maxakato and P. N. Nomngongo, Preparation of magnetic Fe3O4 nanocomposites modified with MnO2, Al2O3, Au and their application for preconcentration of arsenic in river water samples, J. Environ. Chem. Eng., 2018, 6(2), 1673–1681 CrossRef CAS.
- C. Liu, X. Zhu, P. Wang, Y. Zhao and Y. Ma, Defects and interface states related photocatalytic properties
in reduced and subsequently nitridized Fe3O4/TiO2, J. Mater. Sci. Technol., 2018, 34(6), 931–941 CrossRef.
- Q. Wu, M. Li, Z. Huang, Y. Shao, L. Bai and L. Zhou, Well-defined nanostructured core–shell magnetic surface imprinted polymers (Fe3O4@SiO2@MIPs) for effective extraction of trace tetrabromobisphenol A from water, J. Ind. Eng. Chem., 2018, 60, 268–278 CrossRef CAS.
- Q. Yang, S. S. Ren, Q. Zhao, R. Lu, H. Cheng, Z. Chen and H. Zheng, Selective separation of methyl orange from water using magnetic ZIF-67 composites, Chem. Eng. J., 2017, 333, 49–57 CrossRef.
- Y. Ren, H. A. Abbood, F. He, H. Peng and K. Huang, Magnetic EDTA-modified chitosan/SiO2/Fe3O4 adsorbent: Preparation, characterization, and application in heavy metal adsorption, Chem. Eng. J., 2013, 226(12), 300–311 CrossRef CAS.
- S. Zhang, Y. Zhang, J. Liu, Q. Xu, H. Xiao, X. Wang, H. Xu and J. Zhou, Thiol modified Fe3O4@SiO2 as a robust, high effective, and recycling magnetic sorbent for mercury removal, Chem. Eng. J., 2013, 226(24), 30–38 CAS.
- J. H. Wang, S. R. Zheng, Y. Shao, J. Liu, Z. Xu and D. Zhu, Amino-functionalized Fe3O4@SiO2 core-shell magnetic nanomaterial as a novel adsorbent for aqueous heavy metals removal, J. Colloid Interface Sci., 2010, 349(1), 293–299 CrossRef CAS PubMed.
- Y. Yan, G. Yuvaraja, C. Liu, L. Kong, K. Guo, G. M. Reddy and G. V. Zyryanov, Removal of Pb(II) ions from aqueous media using epichlorohydrin crosslinked chitosan schiff's base@Fe3O4 (ECCSB@Fe3O4), Int. J. Biol. Macromol., 2018, 117, 1305–1313 CrossRef CAS PubMed.
- L. Yan, S. Li, H. Yu, R. Shan, B. Du and T. Liu, Facile solvothermal synthesis of Fe3O4/bentonite for efficient removal of heavy metals from aqueous solution, Powder Technol., 2016, 301, 632–640 CrossRef CAS.
- J. Li, Z. Shao, C. Chen and X. Wang, Hierarchical GOs/Fe3O4/PANI magnetic composites as adsorbent for ionic dye pollution treatment, RSC Adv., 2014, 4(72), 38192–38198 RSC.
- Q. Zhang, M. He, B. Chen and B. Hu, Magnetic mesoporous carbons derived from in situ MgO template formation for fast removal of heavy metal ions, ACS Omega, 2018, 3(4), 3752–3759 CrossRef CAS.
- S. Zhang, Z. Wang, H. Chen, C. Kai, M. Jiang, Q. Wang and Z. Zhou, Polyethylenimine functionalized Fe3O4/steam-exploded rice straw composite as an efficient adsorbent for Cr(VI) removal, Appl. Surf. Sci., 2018, 440, 1277–1285 CrossRef CAS.
- G. Bayramoglu and M. Y. Arica, Adsorption of Cr(VI) onto PEI immobilized acrylate-based magnetic beads: Isotherms, kinetics and thermodynamics study, Chem. Eng. J., 2008, 139(1), 20–28 CrossRef CAS.
- C. Xiao, X. Liu, S. Mao, L. Zhang and J. Lu, Sub-micron-sized polyethylenimine-modified polystyrene/Fe3O4/chitosan magnetic composites for the efficient and recyclable adsorption of Cu(II) ions, Appl. Surf. Sci., 2017, 394, 378–385 CrossRef CAS.
- T. Zhang, X. Zhang, X. Yan, L. Kong, G. Zhang, H. Liu, J. Qiu and K. L. Yeung, Synthesis of Fe3O4@ZIF-8 magnetic core–shell microspheres and their potential application in a capillary microreactor, Chem. Eng. J., 2013, 228(28), 398–404 CrossRef CAS.
- C. Hui, C. Shen, J. Tian, L. Bao, H. Ding, C. Li, Y. Tian, X. Shi and H. Gao, Core-shell Fe3O4@SiO2 nanoparticles synthesized with well-dispersed hydrophilic Fe3O4 seeds, Nanoscale, 2011, 3(2), 701–705 RSC.
- L. Wang, D. Hu, X. Kong, X. Li, K. Zhou, H. Zhao and C. Zhou, Anionic polypeptide poly(γ-glutamic acid)-functionalized magnetic Fe3O4-GO-(o-MWCNTs) hybrid nanocomposite for high-efficiency removal of Cd(II), Cu(II) and Ni(II) Heavy metal ions, Chem. Eng. J., 2018, 346, 38–49 CrossRef CAS.
- Y. Zhang, W. Liu, Z. Li, M. Wang and M. Zhao, Application of bifunctional saccharomyces cerevisiae to remove lead(II) and cadmium(II) in aqueous solution, Appl. Surf. Sci., 2011, 257(23), 9809–9816 CrossRef CAS.
- N. Mir, A. Heidari, H. Beyzaei, S. Mirkazehi-Rigi and P. Karimi, Detection of Hg2+ in aqueous solution by pyrazole derivative-functionalized Fe3O4@SiO2 fluorescent probe, Chem. Eng. J., 2017, 327, 648–655 CrossRef CAS.
- L. Cao, Q. Q. Sun, Y. H. Gao, L. T. Liu and H. F. Shi, Novel acid-base hybrid membrane based on amine-functionalized reduced graphene oxide and sulfonated polyimide for vanadium redox flow battery, Electrochim. Acta, 2015, 18, 24–34 CrossRef.
- J. Kim, H. S. Kim, N. Lee, T. Kim, H. Kim, T. Yu, I. C. Song, W. K. Moon and T. yeon, Multifunctional uniform nanoparticles composed of a magnetite nanocrystal core and a mesoporous silica shell for magnetic resonance and fluorescence imaging and for drug delivery, Angew. Chem., 2008, 47(44), 8438–8441 CrossRef CAS PubMed.
- W. S. W. Ngah, C. S. Endud and R. Mayanar, Removal of copper(II) ions from aqueous solution onto chitosan and cross-linked chitosan beads, React. Funct. Polym., 2002, 50(2), 181–190 CrossRef CAS.
- R. Katal, E. Hasani, M. Farnam, M. S. Baei and M. A. Ghayyem, Charcoal ash as an adsorbent for Ni(II) adsorption and Its application for wastewater treatment, J. Chem. Eng. Data, 2012, 57(2), 374–383 CrossRef CAS.
- W. C. Kao, J. Y. Wu, C. C. Chang and J. Chang, Cadmium biosorption by polyvinyl alcohol immobilized recombinant escherichia coli, J. Hazard. Mater., 2009, 169(1–3), 651–658 CrossRef CAS PubMed.
- Z. F. Liu, G. M. Zeng, H. Zhong, X. Yuan, L. Jiang, H. Fu, X. Ma and J. Zhang, Effect of saponins on cell surface properties of penicillium simplicissimum: performance on adsorption of cadmium(II), Colloids Surf., B, 2011, 86(2), 364–369 CrossRef CAS PubMed.
- C. Tamerler, E. E. Oren, M. Duman, E. Venkatasubramanian and M. Sarikaya, Adsorption kinetics of an engineered gold binding peptide by surface plasmon resonance spectroscopy and a quartz crystal microbalance, Langmuir, 2006, 22(18), 7712–7718 CrossRef CAS PubMed.
- J. Ethève and D. Philippe, Adsorption kinetics of lysozyme on silica at pH 7.4:
correlation between streaming potential and adsorbed amount, Langmuir, 2002, 18(5), 1777–1785 CrossRef. - L. G. Yan, K. Yang, R. R. Shan, T. Yan, J. Wei, S. Yu, H. Yu and B. Du, Kinetic, isotherm and thermodynamic investigations of phosphate adsorption onto core-shell Fe3O4@LDHs composites with easy magnetic separation assistance, J. Colloid Interface Sci., 2015, 448, 508–516 CrossRef CAS PubMed.
- L. A. Rodrigues and M. L. C. P. D. Silva, Thermodynamic and kinetic investigations of phosphate adsorption onto hydrous niobium oxide prepared by homogeneous solution method, Desalination, 2010, 263(1), 29–35 CrossRef CAS.
- S. Venkateswarlu and M. Yoon, Core-shell ferromagnetic nanorod based on amine polymer composite (Fe3O4@DAPF) for fast removal of Pb(II) from aqueous solutions, ACS Appl. Mater. Interfaces, 2015, 7(45), 25362–25372 CrossRef CAS PubMed.
- K. Zargoosh, H. Abedini, A. Abdolmaleki and M. R. Molavian, Effective removal of heavy metal ions from industrial wastes using thiosalicylhydrazide-modified magnetic nanoparticles, Ind. Eng. Chem. Res., 2013, 52(42), 14944–14954 CrossRef CAS.
- J. Wang, S. Zheng, Y. Shao, J. Liu, Z. Xu and D. Zhu, Amino-functionalized Fe3O4@SiO2 core-shell magnetic nanomaterial as a novel adsorbent for aqueous heavy metals removal, J. Colloid Interface Sci., 2010, 349(1), 293–299 CrossRef CAS PubMed.
- M. Y. Lee, J. H. Lee, J. W. Chung and S. Kwak, Hydrophilic and positively charged polyethylenimine-functionalized mesoporous magnetic clusters for highly efficient removal of Pb(II) and Cr(VI) from wastewater, J. Environ. Manage., 2017, 206, 740–748 CrossRef PubMed.
- X. Jin, Z. Xiang, Q. Liu, Y. Chen and F. Lu, Polyethyleneimine-bacterial cellulose bioadsorbent for effective removal of copper and lead ions from aqueous solution, Bioresour. Technol., 2017, 244(1), 844–849 CrossRef CAS PubMed.
- J. Zhao, J. Liu, N. Li, W. Wang, J. Nan, Z. Zhao and F. Cui, Highly efficient removal of bivalent heavy metals from aqueous systems by magnetic porous Fe3O4-MnO2: Adsorption behavior and process study, Chem. Eng. J., 2016, 304, 737–746 CrossRef CAS.
- L. Zhang, J. Guo, X. Huang, W. Wang, P. Sun, Y. Li and J. Han, Functionalized biochar-supported magnetic MnFe2O4 nanocomposite for the removal of Pb(II) and Cd(II), RSC Adv., 2019, 9, 365–376 RSC.
- A. Abdolmaleki, S. Mallakpour and S. Borandeh, Efficient heavy metal ion removal by triazinyl-β-cyclodextrin functionalized iron nanoparticles, RSC Adv., 2015, 5, 90602–90608 RSC.
- Y. Li, J. He, K. Zhang, T. Liu, Y. Hu, X. Chen, C. Wang, X. Huang, L. Kong and J. Liu, Super rapid removal of copper, cadmium and lead ions from water by NTA-silica gel, RSC Adv., 2019, 9, 397–407 RSC.
|
This journal is © The Royal Society of Chemistry 2019 |