DOI:
10.1039/C8RA10238D
(Paper)
RSC Adv., 2019,
9, 6152-6162
Preparation of S–N co-doped CoFe2O4@rGO@TiO2 nanoparticles and their superior UV-Vis light photocatalytic activities
Received
13th December 2018
, Accepted 22nd January 2019
First published on 19th February 2019
Abstract
A S–N co-doped CoFe2O4@rGO@TiO2 (CFGT-S/N) nanocomposite was successfully synthesized via a facile vapor-thermal method. XRD, XPS, FT-IR and FETEM results confirmed that N and S were co-doped into the lattice of TiO2. Photocatalytic tests indicated that CFGT-S/N exhibited excellent UV-Vis photocatalytic activity for decompositions of different organic dyes, including methyl orange (MO), rhodamine B (RhB) and methylene blue (MB). Particularly, the photocatalytic degradation rate of MO was about 33% higher than that when using P25 under visible light irradiation. The higher UV-Vis light photocatalytic activity of CFGT-S/N can be attributed to the synergetic effects of the strong absorption of visible light, the narrow band gap, improved separation of photo-generated electron/hole pairs, and the enhancement of the enrichment of pollutant dye molecules by S, N co-doping, CoFe2O4 and rGO. Moreover, this photocatalyst was superparamagnetic, which enables it to be easily recovered by an external magnetic field, and maintained stable photocatalytic efficiency over five cycles. Hence, CFGT-S/N with its highly efficient, recoverable and stable photocatalytic properties shows great potential for environmental treatment.
1 Introduction
Titanium dioxide (TiO2) is a conventional photocatalyst widely used for the degradation of organic pollutants in wastewater, because of its powerful oxidizing power, high benefit-to-cost ratio, nontoxicity, and its extended stability against photo- and chemical corrosion.1 However, TiO2 can be activated only under UV-light irradiation due to its wide bandgap (Eg = 3.2 eV for anatase TiO2), which might hinder its practical application.2 To improve the utilization of solar light, a lot of researchers have shown that doping TiO2 with non-metal elements such as C, N, F, S and B could be an effective strategy.3–7 In order to further achieve photocatalytic activity, co-doping TiO2 with two or three non-metal elements has attracted much attention, such as N–F,5,8 N–B,9 C–N,10 S–N,11,12 S, N and C,3,13 and so on. Density functional theory (DFT) calculations have shown that the doping S, N elements could lower the band gap of TiO2 effectively.14 Our previous work12 also proved that a S–N co-doped anatase nanosized TiO2 photocatalyst exhibited a high activity for the decomposition of methyl orange under both UV-light and Vis-light irradiation comparing to S-doped TiO2 and undoped TiO2 due to the synergetic effects induced by N and S co-doping.
In recent years, graphene has attracted much attention because it is an ideal electron acceptor for nanocomposites during the photocatalytic process, which can slow the recombination of photoexcited electron–hole pairs, increasing the charge transfer rate of electrons.15,16 What is more, the high surface area of graphene based on nanocomposites can provide more active sites to adsorb reactant molecules. Many researchers have indicated that graphene–TiO2 hybrid materials exhibited enhanced photoactivity over bare TiO2 during decomposition of organic dyes in water.17–19
Although the photocatalytic performance of graphene–TiO2 nanocomposites has improved, separation and recycling is still a major bottleneck for their application. To solve the separation of the catalyst, a method to integrate graphene–TiO2 with magnetic nanocomposites, such as Fe3O4,17,20 CoFe2O4,21 ZnFe2O4,22 and so on, was proposed, which enables recycling and removal of the magnetic particles by an external magnetic field. Wherein, cobalt ferrite (CoFe2O4) with a spinel crystallographic structure and a narrow band-gap exhibited a superior magnetic behavior and visible light response.21,23–25 But these TiO2 and magnetic nanoparticles loaded on the surface of graphene sheets may have the defects of detachment and could not effectively prevent photodissolution and recombination of electron–hole pairs, which decreases their photocatalytic activity.26,27 Recently, Yang et al.28 synthesized a ternary nanocomposite with TiO2 nanoparticles anchored on reduced graphene oxide (rGO)-encapsulated Fe3O4 spheres (Fe3O4@rGO@TiO2) by an electrostatic layer-by-layer method. The results showed that the three components of Fe3O4@rGO@TiO2 possessed synergistic effect, and the catalyst exhibited enhancing catalytic activity for the degradation of MB. However, its preparation was more complicated, and visible light activity was high only in the presence of H2O2.
Recently, our group has prepared a magnetic CoFe2O4@rGO core–shell nanocomposite as an efficient adsorbent for removal of organic pollutants, which had excellent adsorption properties and selectivity.29 On this basis, we provided a facile and feasible way to synthesize S and N co-doped CoFe2O4@rGO@TiO2 composites, which possessed excellent photocatalytic activity under both ultraviolet and visible light irradiation. Physical and chemical characterizations of catalysts were conducted, and the photocatalytic activity was evaluated in the photocatalytic degradation of organic dyes. This work may be helpful for preparing novel UV-light and Vis-light photocatalysts with good performance and recoverable ability.
2 Experimental
2.1 Materials and chemicals
Graphite powder, ethylene glycol (EG), polyethylene 2000 (PEG 2000), anhydrous ferric chloride (FeCl3), cobalt(II) chloride hexahydrate (CoCl2·6H2O), sodium acetate (NaAc), tetrabutyltitanate (TBOT), thiourea, methylene blue, methylene orange, rhodamine B and other reagents were purchased from Sinopharm Group Chemical Reagent Co (China). All chemicals used were analytical grade and were used without further purification.
2.2 Preparation of the S, N co-doped CoFe2O4@rGO@TiO2 nanocomposite
Graphene oxide was prepared by a modified Hummers method.30 The preparation of the binary core–shell composites CoFe2O4@rGO (CFG) using a one-step hydrothermal method has been reported by our laboratory.29 The S–N co-doped CoFe2O4@rGO@TiO2 nanocomposite was synthesized via the vapor-thermal method20 with minor modifications. Briefly, 0.8 mL of TBOT and 60 mg of thiourea were added into 10 mL ethanol, and then the beaker was placed in an ultrasonic bath to completely dissolve the resulting mixture. After that, 50 mg of the as-prepared CFG was added into the above clear solution with the help of ultrasound for two hours, and then the suspension was transferred into a 25 mL beaker, which was placed into a 100 mL stainless steel autoclave with a Teflon-liner filled with de-ionized water. The autoclave was heated to 180 °C and kept for 36 h. During the reaction, the de-ionized water vaporized and led to TBOT hydrolysis. At the end of the reaction, the autoclave was cooled to ambient temperature, and the precipitate obtained was repeatedly washed with de-ionized water and ethanol, and then dried at 60 °C in a vacuum oven for 8 h. Furthermore, undoped CoFe2O4@rGO@TiO2 (CFGT) was synthesized as above without thiourea.
2.3 Characterization
X-ray powder diffraction (XRD) was used to characterize the phase structures and particle sizes of the as-prepared samples, using a Rigaku D/max 2500 pc X-ray diffractometer with Cu Kα radiation (λ = 1.54056 Å) at a scan rate of 0.02° s−1. The morphology and structure of the catalysts were examined by field emission transmission electron microscopy (HRTEM, JEM-2100F, Japan). Fourier transform infrared (FT-IR) spectra on KBr pellets of the samples were recorded on a PE-100 FT-IR spectrometer at a resolution of 4 cm−1. Diffuse reflectance spectra (DRS) of samples were obtained using a Varian Cary 5000 spectrometer equipped with a Cary Labsphere DRA-CA-301 DR accessory. Photocurrent density was measured by a CHI electrochemical analyzer (CHI 660e, China) in a standard three-electrode configuration, with the working electrode (an effective area of 2 cm2), a platinum foil as the counter electrode, and a saturated calomel electrode (SCE) as the reference electrode. Na2SO4 (0.01 M) was used as the electrolyte. X-ray photoelectron spectroscopy (XPS) was conducted with an ESCALAB250 (Vacuum Generators) spectrometer using monochromatized Al K X-rays (150 W). The magnetic properties of the samples were determined by a vibrating sample magnetometer (VSM, Lake Shore 7410, USA) at a temperature of 300 K.
2.4 Photocatalytic experiments
The photocatalytic performance of the as-prepared samples was evaluated by measuring the decomposition of a 5 mg L−1 organic dyes aqueous solution. UV-light photocatalytic experiments were carried out in a homemade jacketed reactor with a 25 W low-pressure mercury lamp as the UV-light source, and the light intensity was 80 μW cm−2. 0.18 g of the as-prepared sample was dispersed in 900 mL of the aqueous dye solution. The Vis-light photocatalytic experiments were carried out in a 250 mL jacketed reactor under a 300 W Xe lamp with a filter to remove light of wavelengths below 420 nm as the Vis-light source, and the light intensity is 240 mW cm−2. 0.08 g of the as-prepared sample was dispersed in 100 mL of the aqueous dye solution. Prior to photocatalytic experiments, the catalysts were settled in suspension for 30 min in the dark for the adsorption equilibrium. The concentration of the organic dye solution was quantified by a Shimadzu UV-2550 (Japan) spectrophotometer at the maximum absorption wavelength of different dyes (λmax (MO) = 464 nm, λmax (MB) = 663 nm and λmax (RhB) = 554 nm). To evaluate the photocatalyst stability, CFGT-S/N were magnetically separated, washed, dried, and reused for the next cycle under the same conditions. The used CFGT-S/N after five cycles was named CFGT-S/N-5. The quenching tests of the active species for the photoreaction were carried out by using different scavengers. The photocatalytic reaction rate constants (k) used the pseudo-first-order model as expressed as eqn (1)where C and C0 were the actual and initial concentrations of the MO solution, respectively, and k was the apparent reaction rate constant. To ensure the accuracy of the experiment, each experiment was carried out three times.
3 Results and discussion
3.1 XRD analysis and FT-IR measurements
The XRD patterns of CFG, CFGT, CFGT-S/N and CFGT-S/N-5 are shown in Fig. 1(a). It can be easily found that the diffraction peaks of CFG at 2θ = 30.0°, 35.4°, 43.5°, 57.2° and 62.7° matched the reflections of (220), (311), (400), (511) and (440), respectively, corresponding to the cubic spinel structure of CoFe2O4 (JCPDS #79-1744).31 No apparent diffraction peaks of GO were observed. This was because the crystal growth of CoFe2O4 between the interlayer of GO destroyed the regular layer stacking, leading to the exfoliation of GO and the disappearance of the (001) diffraction peak during the hydrothermal reaction.32 After coating with a TiO2 layer, a new crystalline phase of anatase TiO2 (JCPDS #21-1272) occurred in the CFGT. However, the diffraction peaks of CoFe2O4 were weakened, which suggested that most of the CoFe2O4 NPs were coated with a TiO2 layer. For the co-doped TiO2 NPs (CFGT-S/N), the main diffraction peak at 2θ = 35.4° of CoFe2O4 almost disappeared, suggesting that its saturation magnetization may be decreased. The unit cell volumes of CFGT and CFGT-S/N determined by the Debye–Scherrer equation were 0.1317 and 0.1359 nm3, which indicated that S and N had been successfully co-doped into the TiO2 lattice.8,33 It was noted that the diffraction pattern of CFGT-S/N-5 was almost same as that of CFGT-S/N, which showed that the catalyst possessed a good stability.
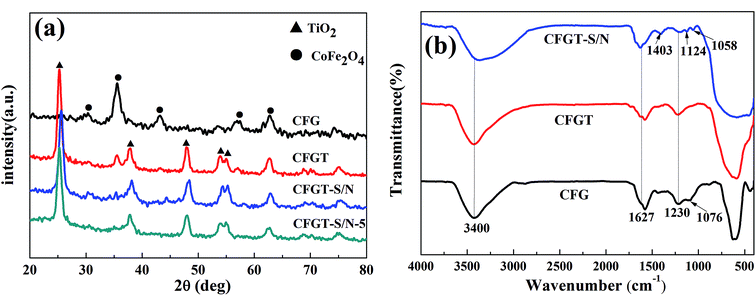 |
| Fig. 1 (a) XRD patterns of CFG, CFGT, CFGT-S/N and CFGT-S/N-5; (b) FT-IR spectra of CFG, CFGT, CFGT-S/N. | |
In addition, the chemical bonding of the sulfur and nitrogen complexes on the surface of TiO2 was examined by FT-IR measurements. Fig. 1(b) presented the FT-IR spectra of the samples ranging from 4000 to 400 cm−1. For the CFG NPs, the band was observed in the range 600–500 cm−1, and was indexed to the intrinsic stretching vibrations of the metal at the octahedral site Co–O and Fe–O in CoFe2O4. The absorption bands at 1230 cm−1 and 1076 cm−1 were associated with the epoxy C–O and alkoxy C–O groups of GO, respectively.16,32 The peak at 1627 cm−1 corresponded to the O–H bending of the adsorbed water molecules, whereas the broad absorption bands at 3400 cm−1 resulted from the O–H stretch region. It can be clearly seen that the main characteristic peaks of the CFG NPs appeared in the spectra of the CFGT NPs and CFGT-S/N NPs, but the GO characteristic peaks were weakened. The absorption bands below 1000 cm−1 can be assigned to the Ti–O–Ti vibration.4 Ammonium ions produced by the dissociation of thiourea were also found in the titania prepared using thiourea as shown by a peak at 1403 cm−1 attributable to the deformation mode of ammonium ions.12 The peak at 1124 cm−1 was assigned to the Ti–S vibration, and the peak at 1058 cm−1 was ascribed to the Ti–O–S vibration.13 The above results confirmed that S and N were successfully implanted in the structure of TiO2.
3.2 UV-Vis DRS spectra analysis
To further investigate the optical properties of the prepared catalysts, the UV-Vis DRS spectra were recorded (Fig. 2). As shown in Fig. 2(a), P25 mainly responded to the ultraviolet region. However, the absorption of TiO2-SN (T-SN) in the visible light region was significantly increased due to co-doping with N and S elements.14,42,46 CoFe2O4 (CF) had broad and obvious absorption ranging in a wide region from 300 to 800 nm owing to its narrow bandgap.21,23–25,34 CFG NPs showed a stronger light absorption than CF, implying that the introduction of rGO can enhance the visible light absorption.17,25,36 Compared with CFG, the absorption intensity of CFGT decreased slightly, which can be attributed to the mixing effect of the two semiconductors introducing defect levels.35 Furthermore, it was found that CFT showed a relatively lower absorption in the visible light region (550–800 nm) than CFGT. After co-doping, the absorption of CFGT-S/N in the visible region improved, hence enhancing the photocatalytic activity.12,37 The plots of the transformed Kubelka–Munck function versus the energy of light were shown in Fig. 2(b). The band gap values of P25, T-SN, CF, CFG, CFT, CFGT and CFGT-S/N were 3.29, 3.08, 1.55, 1.45, 2.33, 2.21 and 2.15 eV, respectively. The results indicated that S, N co-doping, CoFe2O4 and rGO can enhance the visible light absorption of CFGT-S/N.
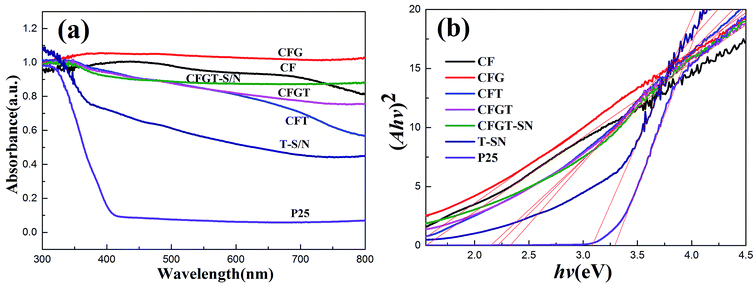 |
| Fig. 2 (a) Diffuse reflectance spectra (DRS); (b) plots of transferred Kubelka–Munk vs. the energy of the light absorbed by the samples. | |
3.3 Morphology and structure analysis
The morphological structure of CFGT-S/N was further verified by FETEM observation. As can be seen in the inset of Fig. 3(a), binary CFG nanoparticles with a core–shell structure can be observed. After TiO2-S/N coating, CFGT-S/N nanocomposites had a spherical morphology and their grain sizes were 160–220 nm in diameter. From the HRTEM image of the CFGT-S/N hybrid (Fig. 3(b)), two different lattices were observed with d spaces of 2.54 and 3.50 Å, corresponding to the (311) plane of CoFe2O4 and the (101) plane of anatase TiO2, respectively, which were quite similar to the literature values.38 In addition, the EDS mapping images clearly showed that Ti, O, C, Fe, Co, S, and N elements were uniformly distributed in the CFGT-S/N sample, further confirming the presence of CoFe2O4, TiO2, rGO, and the elements S and N (Fig. 3(d–j)).
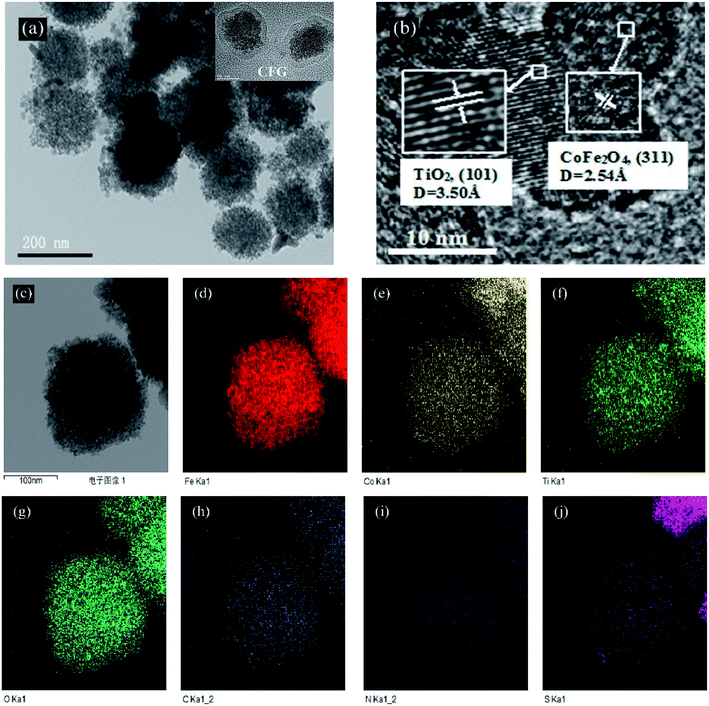 |
| Fig. 3 (a) and (c) FETEM and (b) HRTEM images of CFGT-S/N; EDS elemental mapping images of the corresponding area: (d) Fe; (e) Co; (f) Ti; (g) O; (h) C; (i) N; and (j) S. | |
3.4 XPS analysis
In order to determine the surface chemical compositions, XPS analysis was carried out. As shown in Fig. 4(a), CFGT contained Fe, Co, C, Ti and O, while CFGT-S/N not only contained Fe, Co, C, Ti, and O, but also S and N, which indicated that S and N had been co-doped into the CFGT-S/N hybrids. As shown in Fig. 4(b), the XPS spectrum of Fe 2p depicted two main broad peaks, 710.9 eV for Fe 2p3/2 and 724.0 eV for Fe 2p1/2, and a small peak at 714.3 eV for Fe 2p3/2, which could be due to the contributions from Fe3+ ions. From Fig. 4(c), the peaks of 779.0 eV, 781.0 eV and 794.2 eV were associated with Co2+ ions.21,39 The C 1s high-resolution spectrum was curve-fitted in Fig. 4(d). The peaks at 284.7, 285.8, 286.9 and 288.6 eV were assigned to C
C/C–C, C–OH, C–O and HO–C
O, respectively.25 For the S 2p XPS spectrum in Fig. 4(e), the characteristic peak in the range of 160–163 eV could be ascribed to the formation of Ti–S bond. It can be deduced that the S2− ions replaced the O2− ions in the lattice of TiO2, making it produce a lattice distortion due to a large ionic radius difference between S2− (0.184 nm) and O2− (0.140 nm).12,33 The peak at 168.8 eV was related to the S6+ of SO42− ions, assigned to the SO42− ions adsorbed on the surface of CFGT-S/N.41 The XPS spectra of the N 1s region are shown in Fig. 4(f). The peak at 400.3 eV was attributed to interstitial nitrogen atoms as Ti–O–N structural characteristic.10,13 From Fig. 4(g), the Ti 2p XPS spectrum of CFGT-S/N appeared two peaks at 459.2 and 465.0 eV, assigned to Ti 2p3/2 and Ti 2p1/2, respectively,22,40 a little lower than those of CFGT (459.5 and 465.1 eV), which could be attributed to partial of oxygen in the lattice of TiO2 replaced by low electronegativity S anions, subsequently leading to the increase of the charge density of Ti atoms, and then decreasing binding energy of Ti 2p.42 This consequence further approved the formation of Ti–S bond. In addition, there is a shift of the O 1s peak by 0.1 eV toward low energy in CFGT-S/N (Fig. 4(h)), indicating the existence of concomitant oxygen deficiencies, which resulted in increased outer electron density of O and, consequently, decreased binding energy.43,50 The result of XPS was in good agreement with FT-IR.
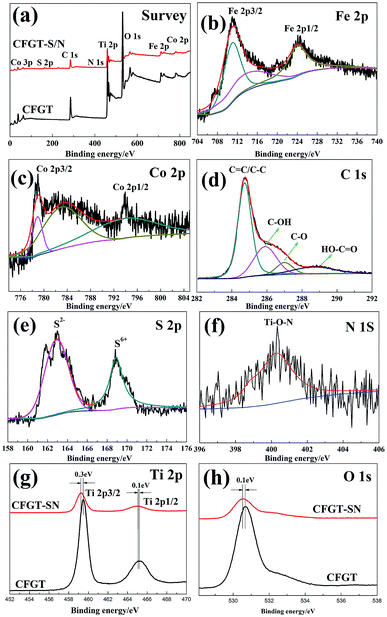 |
| Fig. 4 (a) XPS survey spectra of CFGT and CFGT-S/N; high-resolution spectra of (b) Fe 2p, (c) Co 2p, (d) C 1s, (e) S 2p and (f) N 1s of CFGT-S/N; and high-resolution spectra of (g) Ti 2p and (h) O 1s of CFGT and CFGT-S/N. | |
3.5 Magnetic characterization
To evaluate the magnetic separation capacity, the magnetic hysteresis loops of CFG, CFGT and CFGT-S/N nanoparticles were measured at room temperature, as shown in Fig. 5. The saturation magnetization (Ms) values of the CFG, CFGT and CFGT-S/N were 32.74, 2.95 and 1.73 emu g−1, respectively. It can be found that the non-magnetic coating TiO2 layer affected the magnetic properties of the CoFe2O4 particles.20,23 Fortunately, the maximum saturation magnetization of 1.73 emu g−1 was strong enough to separate CFGT-S/N from solution with the help of an external magnetic force. The magnetic separability was tested by placing a magnet near a glass bottle. The black product was rapidly attracted toward the magnet in a short period (the inset of Fig. 5), demonstrating the high magnetic sensitivity of the CFGT-S/N nanocomposites.
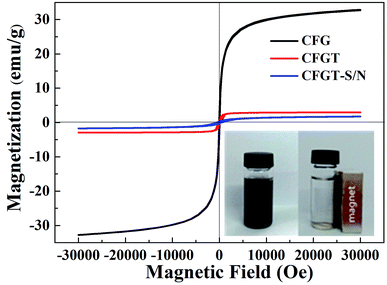 |
| Fig. 5 VSM magnetization curves of CFG, CFGT and CFGT-S/N. The inset shows the separation of CFGT-S/N from aqueous solution under an external magnetic field. | |
3.6 Photocatalytic activities
3.6.1 Photodegradation of MO. The photocatalytic activities of CFT, CFGT, CFGT-S/N, P25, and CoFe2O4 were tested using the degradation of MO under UV- and Vis-light irradiation and the results were displayed in Fig. 6. From Fig. 6(a), it can be seen that CF displayed poor UV-light photocatalytic activity and P25 exhibited the highest photocatalytic activity. For CFT, MO degradation rate was only 74% within 150 min, while for CFGT, 100% of MO was degraded due to the rGO acting as an acceptor of the electrons generated in the TiO2 particles, suppressing the recombination of charges.26 After S, N co-doping, CFGT-S/N exhibited an improved photocatalytic activity compared to CFGT, and it took around 120 min to decompose MO completely. Furthermore, Fig. 6(b) illustrates that the photocatalytic reaction rate constants (k) onto CF, CFT, CFGT, CFGT-S/N and P25, were in turn 0.0009, 0.0081, 0.0133, 0.0192 and 0.1014 min−1. Due to the lower content of TiO2 in CFGT-S/N at the same dosage of catalysts, its rate constant k was lower than that of P25. As shown in Fig. 6(c), almost no degradation of MO over bare CF and CFT was observed under visible light irradiation. Although CFGT exerted facile MO adsorption, it displayed less photocatalytic activity. Notably, CFGT-S/N exhibited better catalytic activity, and the degradation rate of MO reached 100% within 6 h. For P25, only 72% of MO was removed within 6 h. It can be seen from Fig. 6(d), the k of CF, CFT, CFGT, CFGT-S/N and P25 was 0, 0, 0.0003, 0.0052 and 0.0039 min−1, respectively. The k of CFGT-S/N was 33% higher than that of P25 under visible light irradiation. It indicated that the prepared CFGT-S/N displayed an excellent photocatalytic activity no matter which light source (UV or Vis) was used.
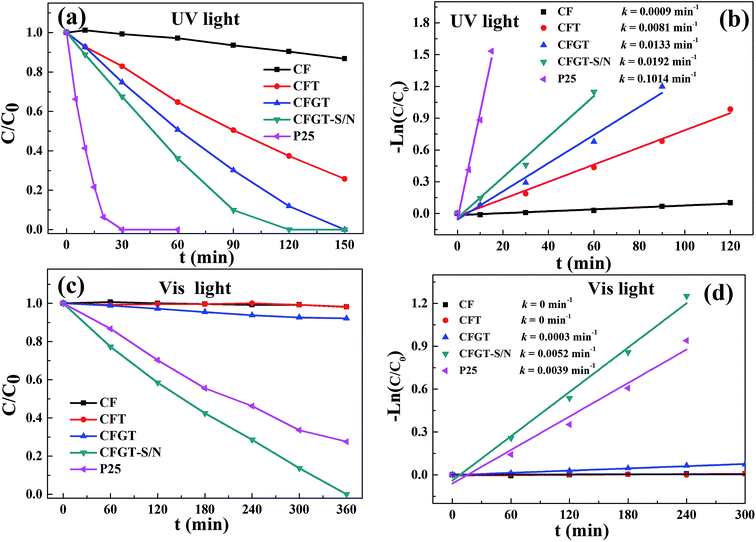 |
| Fig. 6 Photodegradation of MO on CF, P25, CFT, CFGT and CFGT-S/N under (a) UV- and (c) Vis-light irradiation; (b) and (d) first-order kinetics rate curves. | |
3.6.2 Photodegradation of various dyes. The photodegradation of the different organic dyes on CFGT-S/N was further studied under UV- and Vis-light irradiation. Two kinds of anionic dyes (RhB and MB) were selected as other pollutants. To confirm whether dyes were not photodegraded by itself, a blank test was also carried out. As shown in Fig. 7(a), CFGT-S/N exhibited excellent UV-light photocatalytic activity, and it only took 120 min to decompose various pollutants molecules completely. Under Vis-light illumination, the photocatalytic degradation rates of pollutants were still lower than those under UV-light illumination as shown in Fig. 7(b). Overall, the CFGT-S/N showed no selectivity to photocatalytic degradation for most organic pollutants, which was of great importance to practical applications.
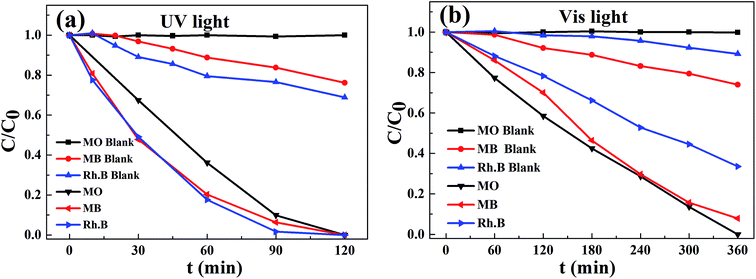 |
| Fig. 7 Photodegradation of various dyes in the presence of CFGT-S/N under (a) UV- and (b) Vis-light irradiation. | |
3.6.3 Cycling photodegradation of MO. As a recyclable photocatalyst, high stability and facile separation of catalysts were also important for the photocatalytic nanomaterial in water remediation.4 Recycling photocatalytic experiments of CFGT-S/N were conducted, and the results are represented in Fig. 8(a and b). After five cycles, the degradation rates of MO were 96% and 92% of that for the first cycle under UV- and Vis-light illumination, respectively. This result implied that the as-prepared catalysts had an excellent chemical stability and could be recycled in the organic dyestuff wastewater purification.
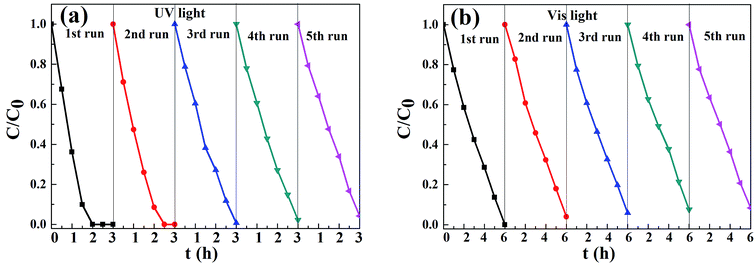 |
| Fig. 8 Cycling photodegradation of MO on CFGT-S/N under (a) UV- and (b) Vis-light irradiation. | |
3.7 Photocatalytic mechanism
To better understand the separation efficiency of the photo-generated electrons and holes of CFGT-S/N, we investigated its photoinduced charge transfer properties.5 Fig. 9(a) shows the photocurrent density variations of the P25, CFG, CFT, CFGT and CFGT-S/N electrodes with measured potential in Na2SO4 solution. The dark current densities in all cases can be negligible. Upon irradiation, a significant increase in the photocurrent density was observed throughout the potential window at the CFGT-S/N electrode. The saturated photocurrent density of the CFGT-S/N electrode was about 0.71 μA cm−2, higher than those of the CFGT (0.08 μA cm−2), CFT (0.05 μA cm−2), CFG (0.05 μA cm−2) and P25 (0.06 μA cm−2) electrodes by a factor of 11.8, 14.2, 14.2 and 8.9, respectively. This demonstrated that the separation rate of photo-generated electrons and holes increased because of the co-doping of S and N in the TiO2 network.
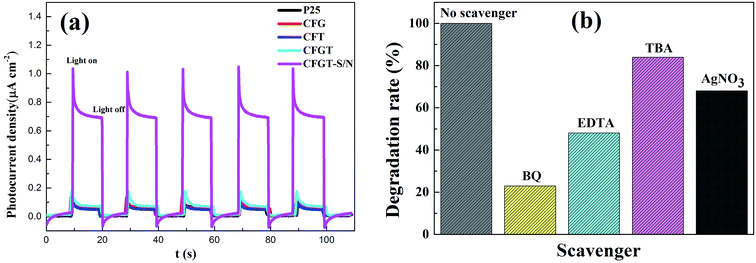 |
| Fig. 9 (a) Photocurrent density of P25, CFG, CFT, CFGT and CFGT-S/N; and (b) photocatalytic activities of CFGT-S/N hybrid in the presence of different scavengers under UV-Vis light irradiation (reaction conditions: [MO] = 5 mg L−1, [catalyst] = 0.20 g L−1). | |
Furthermore, to verify the role of the reactive species under UV-Vis light irradiation over CFGT-S/N, different scavengers were employed in this study. They were benzoquinone (0.1 mM BQ) for the superoxide radical (O2˙−), tert-butyl alcohol (0.1 mM TBA) for the hydroxyl radical (·OH), AgNO3 (0.01 mM) for photogenerated electrons (e−) and disodium ethylenediamine-tetraacetate (0.1 mM EDTA) for photo-generated holes (h+).3,4 As shown in Fig. 9(b), upon the addition of BQ and EDTA, the degradation rates were greatly suppressed to 23% and 48%, respectively. After the addition of AgNO3, the photodegradation rate of MO decreased from 100% to 68%, indicating that e− was definitely produced and transferred from TiO2-SN to rGO in the photocatalytic reaction, which was consistent with the literature reports.47,50 Besides, a significant change was observed in the presence of TBA, displaying that ·OH as an oxidation species was also indeed photogenerated on catalyst surfaces. The results suggested that O2˙−, h+, e− and ·OH were the main active species in the MO photocatalytic oxidation process.
On the basis of the above experimental results, a possible photocatalytic mechanism for CFGT-S/N under UV-Vis irradiation was proposed and is illustrated in Scheme 1. Upon UV-Vis irradiation, CoFe2O4 (1.55 eV) with a narrow bandgap energy could be easily excited from the valence band (VB) to the conduction band (CB), inducing the generation of electron–hole pairs (eqn (2)).24,25,44,45 Notably, the introduction of CoFe2O4 with superparamagnetic behavior was conducive to catalyst recycling.23,25 For TiO2, the co-doping of nitrogen and sulfur into the TiO2 lattice led to the formation of mid-gap energy levels such as N 2p and S 2p between the valence band (VB) of O 2p and the conduction band (CB) of Ti 3d and narrowed the bandgap energy to 3.08 eV, thereby TiO2-S/N can be excited easily by the photons (eqn (3)).42,46,52,53 The photoinduced electrons (e−) from TiO2-S/N can be quickly transferred to the huge π–π network of rGO due to their excellent electron accepting and transporting properties,36,47 and then rGO (e−) reacted with dissolved O2 to generate O2˙− radicals (eqn (4) and (5)). This process can restrain effectively the electron–hole recombination on TiO2-S/N.48,49 The photoinduced holes (h+) remaining in the VB of TiO2-SN can directly degrade MO, and can also be captured by H2O to form ·OH radicals (eqn (6)).45,46,50 Moreover, the h+ on the VB of TiO2-S/N can be transferred to the VB of CoFe2O4 due to the lower potential energy of the VB of CoFe2O4, which can facilitate efficiently interface charge separation and hamper carrier recombination.44,51 As a result, these highly reactive oxygen species (ROS, such as O2˙−, h+ and ·OH) can effectively degrade MO adsorbed on CFGT-SN (eqn (7)). According to the above analysis, the relevant reactions on the surface of nanocomposite can be expressed as follows:
|
CoFe2O4 + hv → CoFe2O4 (h+ + e−)
| (2) |
|
TiO2-S/N + hv → TiO2-S/N (h+ + e−)
| (3) |
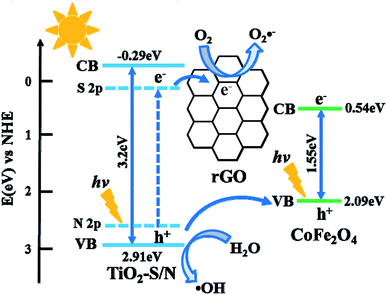 |
| Scheme 1 The possible photocatalytic mechanism on the CFGT-S/N nanocomposite. | |
4 Conclusions
In summary, we have presented a simple and controllable way to synthesise magnetically separable S, N co-doped CoFe2O4@rGO@TiO2 composites, which has not been reported before. This method can efficiently dope sulfur and nitrogen elements into the lattice of TiO2. The results showed that CFGT-S/N exhibited excellent photocatalytic activity no matter which light source (UV or Vis) was used. The high activity of CFGT-S/N was ascribed to a synergetic consequence of several beneficial effects, such as the efficient separation of photoinduced electron–hole pairs and the improvement in UV-Vis absorption. Furthermore, the presence of CoFe2O4 in the hybrid helped the catalyst particles to acquire ferromagnetic properties, and they were easily separated from the catalytic solution. Therefore, the synthesized CFGT-S/N can be used as a composite photocatalyst for the elimination of organic pollutants from waste water.
Conflicts of interest
There are no conflicts to declare.
Acknowledgements
The authors gratefully acknowledge financial support from the National Natural Science Foundation of China (No. 51372062).
References
- J. Schneider, M. Matsuoka, M. Takeuchi, J. L. Zhang, Y. Horiuchi, M. Anpo and D. W. Bahnemann, Understanding TiO2 photocatalysis: mechanisms and materials, Chem. Rev., 2014, 114(19), 9919–9986 CrossRef CAS PubMed.
- M. Liu, R. Inde, M. Nishikawa, X. Q. Qiu, D. Atarashi, E. Sakai, Y. Nosaka, K. Hashimoto and M. Miyauchi, Enhanced photoactivity with nanocluster-grafted titanium dioxide photocatalysts, ACS Nano, 2014, 8(7), 7229–7238 CrossRef CAS PubMed.
- C. Burda and X. Chen, The electronic origin of the visible-light absorption properties of C-, N- and S-doped TiO2 nanomaterials, J. Am. Chem. Soc., 2008, 130(15), 5018–5019 CrossRef PubMed.
- Y. J. Yao, J. C. Qin, H. Chen, F. Y. Wei, X. T. Liu, J. L. Wang and S. B. Wang, One-pot approach for synthesis of N-doped TiO2/ZnFe2O4 hybrid as an efficient photocatalyst for degradation of aqueous organic pollutants, J. Hazard. Mater., 2015, 291, 28–37 CrossRef CAS PubMed.
- F. Y. Wei, T. Liu, F. Z. Zhou, W. Ran, Y. J. Yao, H. Wang and P. H. Wang, Magnetic recoverable F-N Co-doped ZnFe2O4/C/TiO2 nanocomposites with UV-Vis light photocatalytic activity, Environ. Eng. Sci., 2018, 35, 37–45 CrossRef CAS.
- T. Ohno, M. Akiyoshi, T. Umebayashi, K. Asai, T. Mitsui and M. Matsumura, Preparation of S-doped TiO2 photocatalysts and their photocatalytic activities under visible light, Appl. Catal., A, 2004, 265(1), 115–121 CrossRef CAS.
- Y. M. Wu, M. Y. Xing, J. L. Zhang and F. Chen, Effective visible light-active boron and carbon modified TiO2 photocatalyst for degradation of organic pollutant, Appl. Catal., B, 2010, 97, 182–189 CrossRef CAS.
- M. Behpour, R. Foulady-Dehaghi and N. Mir, Considering photocatalytic activity of N/F/S-doped TiO2 thin films in degradation of textile waste under visible and sunlight irradiation, Sol. Energy, 2017, 158, 636–643 CrossRef CAS.
- S. In, A. Orlov, R. Berg, F. García, S. Pedrosa-Jimenez, M. S. Tikhov, D. S. Wright and R. M. Lambert, Effective visible light-activated B-doped and B,N-codoped TiO2 photocatalysts, J. Am. Chem. Soc., 2007, 129(45), 13790–13791 CrossRef CAS PubMed.
- X. P. Wang and T. Lim, Solvothermal synthesis of C-N codoped TiO2 and photocatalytic evaluation for bisphenol a degradation using a visible-light irradiated LED photoreactor, Appl. Catal., B, 2010, 100, 355–364 CrossRef CAS.
- N. C. Birben, C. S. Uyguner-Demirel, S. Sen-Kavurmaci, Y. Y. Gurkan, N. Turkten, Z. Cinar and M. Bekbolet, Comparative evaluation of anion doped photocatalysts on the mineralization and decolorization of natural organic matter, Catal. Today, 2015, 240, 125–131 CrossRef CAS.
- F. Y. Wei, L. S. Ni and P. Cui, Preparation and characterization of N-S-codoped TiO2 photocatalyst and its photocatalytic activity, J. Hazard. Mater., 2008, 156, 135–140 CrossRef CAS PubMed.
- G. Zhang, C. Z. Yong, M. Nadagouda, C. Han, K. O'Shea, S. M. El-Sheikh, A. A. Ismail and D. D. Dionysiou, Visible light-sensitized S, N and C co-doped polymorphic TiO2 for photocatalytic destruction of microcystin-LR, Appl. Catal., B, 2014, 144(1), 614–621 CrossRef CAS.
- W. W. Zhao, F. H. Tian, X. B. Wang, L. H. Zhao, L. H. Xia and C. B. Liu, Theoretical study on S-, N-doped anatase TiO2: the visible-light-driven photocatalysts, Journal of Computational Science & Engineering, 2013, 4, 170–177 Search PubMed.
- Q. Y. He, H. G. Sudibya, Z. Y. Yin, S. X. Wu, H. Li, F. Boey, W. Huang, P. Chen and H. Huang, Centimeter-long and large-scale micropatterns of reduced graphene oxide films: fabrication and sensing applications, ACS Nano, 2010, 4, 3201–3208 CrossRef CAS PubMed.
- D. Y. Liang, C. Cui, H. H. Hu, Y. P. Wang, S. Xu, B. L. Ying, P. G. Li, B. Q. Lu and H. L. Shen, One-step hydrothermal synthesis of anatase TiO2/reduced graphene oxide nanocomposites with enhanced photocatalytic activity, J. Alloys Compd., 2014, 582, 236–240 CrossRef CAS.
- Z. Q. Li, H. L. Wang, L. Y. Zi, J. J. Zhang and Y. S. Zhang, Preparation and photocatalytic performance of magnetic TiO2–Fe3O4/graphene (RGO) composites under Vis-light irradiation, Ceram. Int., 2015, 41, 10634–10643 CrossRef CAS.
- H. Liu, X. N. Dong, X. C. Wang, C. C. Sun, J. Q. Li and Z. F. Zhu, A green and direct synthesis of graphene oxide encapsulated TiO2 core/shell structures with enhanced photoactivity, Chem. Eng. J., 2013, 230, 279–285 CrossRef CAS.
- H. Kim, G. Moon and M. Damian, Solar photoconversion using Graphene/TiO2 composites: nanographene shell on TiO2 core versus TiO2 nanoparticles on graphene sheet, J. Phys. Chem. C, 2012, 116, 1535–1543 CrossRef CAS.
- P. C. Ma, W. Jiang, F. H. Wang, F. S. Li, P. Shen, M. D. Chen, Y. J. Wang, J. Liu and P. Y. Li, Synthesis and photocatalytic property of Fe3O4@TiO2 core/shell nanoparticles supported by reduced graphene oxide sheets, J. Alloys Compd., 2013, 578, 501–506 CrossRef CAS.
- V. K. Gupta, T. Eren, N. Atar, M. L. Yole, C. Parlak and H. Karimi-Maleh, CoFe2O4@TiO2 decorated reduced graphene oxide nanocomposite for photocatalytic degradation of chlorpyrifos, J. Mol. Liq., 2015, 208, 122–129 CrossRef.
- J. T. Feng, Y. C. Wang, Y. H. Hou and L. C. Li, Tunable design of yolk–shell ZnFe2O4@RGO@TiO2 microspheres for enhanced high-frequency microwave absorption, Inorg. Chem. Front., 2017, 4, 935–945 RSC.
- S. M. Moosavi, P. Molla-Abbasi and Z. Haji-Aghajani, Photo-catalyst CoFe2O4–TiO2: application in photo-degradation of organic dyes and magnetic nanocomposite preparation, J. Mater. Sci.: Mater. Electron., 2016, 27, 4879–4886 CrossRef CAS.
- P. Sathishkumar, R. V. Mangalaraja, S. Anandan and M. Ashokkumar, CoFe2O4/TiO2, nanocatalysts for the photocatalytic degradation of reactive red 120 in aqueous solutions in the presence and absence of electron acceptors, Chem. Eng. J., 2013, 220, 302–310 CrossRef CAS.
- J. W. Sun, Y. S. Fu, P. Xiong, X. Q. Sun, B. H. Xu and X. Wang, A magnetically separable P25/CoFe2O4/graphene catalyst with enhanced adsorption capacity and visible-light-driven photocatalytic activity, RSC Adv., 2013, 3, 22490–22497 RSC.
- Y. Lin, Z. G. Geng, H. B. Cai, L. Ma, J. Chen, J. Zeng, N. Pan and X. P. Wang, Ternary graphene–TiO2–Fe3O4 nanocomposite as a recollectable photocatalyst with enhanced durability, Eur. J. Inorg. Chem., 2012, 28, 4439–4444 CrossRef.
- S. M. Li, B. Wang, J. H. Liu and M. Yu, In situ one-step synthesis of CoFe2O4/graphene nanocomposites as high performance anode for lithium-ion batteries, Electrochim. Acta, 2014, 129, 33–39 CrossRef CAS.
- X. L. Yang, W. Chen, J. F. Huang, Y. Zhou, Y. H. Zhu and C. Z. Li, Rapid degradation of methylene blue in a novel heterogeneous Fe3O4@rGO@TiO2-catalyzed photo-fenton system, Sci. Rep., 2015, 5, 10632–10642 CrossRef PubMed.
- Z. J. Song, W. Ran and F. Y. Wei, One-step approach for the synthesis of CoFe2O4@rGO core-shell nanocomposites as efficient absorbent for removal of organic pollutants, Water Sci. Technol., 2017, 75, 397–405 CrossRef CAS PubMed.
- D. C. Marcano, D. V. Kosynkin, J. M. Berlin, A. Sinitskii, Z. Z. Sun, A. Slesarev, L. B. Alemany, W. Lu and J. M. Tour, Improved synthesis of graphene oxide, ACS Nano, 2010, 4, 4806–4814 CrossRef CAS PubMed.
- Y. J. Yao, Z. H. Yang, D. W. Zhang, W. H. Peng, H. Q. Sun and S. Wang, Magnetic CoFe2O4-graphene hybrids: facile synthesis, characterization, and catalytic properties, Ind. Eng. Chem. Res., 2012, 51, 6044–6051 CrossRef CAS.
- L. Gan, S. M. Shang, C. W. M. Yuen, S. X. Jiang and E. L. Hu, Hydrothermal synthesis of magnetic CoFe2O4/graphene nanocomposites with improved photocatalytic activity, Appl. Surf. Sci., 2015, 351, 140–147 CrossRef CAS.
- D. Ma, Y. J. Xin, M. C. Gao and J. Wu, Fabrication and photocatalytic properties of cationic and anionic S-doped TiO2 nanofibers by electrospinning, Appl. Catal., B, 2014, 147, 49–57 CrossRef CAS.
- M. H. Habibi and J. Parhizkar, Cobalt ferrite nano-composite coated on glass by doctor blade method for photo-catalytic degradation of an azo textile dye reactive red 4: XRD, FESEM and DRS investigations, Spectrochim. Acta, Part A, 2015, 150, 879–885 CrossRef CAS PubMed.
- P. P. Hankare, R. P. Patil, A. V. Jadhav, K. M. Garadkar and R. Sasikala, Enhanced photocatalytic degradation of methyl red and thymol blue using titania–alumina–zinc ferrite nanocomposite, Appl. Catal., B, 2011, 107, 333–339 CrossRef CAS.
- G. Y. He, J. J. Ding, J. G. Zhang, Q. L. Hao and H. Q. Chen, One-step ball-milling preparation of highly photocatalytic active CoFe2O4-reduced graphene oxide heterojunctions for organic dye removal, Ind. Eng. Chem. Res., 2015, 54, 2862–2867 CrossRef CAS.
- M. Behpour and V. Atouf, Study of the photocatalytic activity of nanocrystalline S, N-codoped TiO2 thin films and powders under visible and sun light irradiation, Appl. Surf. Sci., 2012, 258(17), 6595–6601 CrossRef CAS.
- W. Li, J. P. Yang, Z. X. Wu, J. X. Wang, B. Li, S. S. Feng, Y. H. Deng, F. Zhang and D. Y. Zhao, A versatile kinetics-controlled coating method to construct uniform porous TiO2 shells for multifunctional core-shell structures, J. Am. Chem. Soc., 2012, 134, 11864–11867 CrossRef CAS PubMed.
- Z. P. Zhou, Y. Zhang, Z. Y. Wang, W. Wei, W. F. Tang, J. Shi and R. Xiong, Electronic structure studies of the spinel CoFe2O4 by X-ray photoelectron spectroscopy, Appl. Surf. Sci., 2008, 254, 6972–6975 CrossRef CAS.
- Y. Min, G. Q. He, R. B. Li, W. Zhao, Y. C. Chen and Y. G. Zhang, Doping nitrogen anion enhanced photocatalytic activity on TiO2 hybridized with graphene composite under solar light, Sep. Purif. Technol., 2013, 106, 97–104 CrossRef CAS.
- C. Han, M. Pelaez, V. Likodimos, A. G. Kontos, P. Falaras, K. Shea and D. D. Dionysiou, Innovative visible light-activated sulfur doped TiO2 films for water treatment, Appl. Catal., B, 2011, 107, 77–87 CrossRef CAS.
- D. Li, Z. P. Xing, X. J. Yu and X. W. Cheng, One-step hydrothermal synthesis of C-N-S-tridoped TiO2-based nanosheets photoelectrode for enhanced photoelectrocatalytic performance and mechanism, Electrochim. Acta, 2015, 170, 182–190 CrossRef CAS.
- R. Huo, J. Y. Yang, Y. Q. Liu, H. F. Liu, X. Li and Y. H. Xu, Preparation of W and N, S co-doped titanium dioxide with enhanced photocatalytic activity under visible light irradiation, Mater. Res. Bull., 2016, 76, 72–78 CrossRef CAS.
- C. Haw, W. Chiu, S. A. Rahman, P. Khiew, S. Radiman, R. A. Shukor, M. A. A. Hamid and N. Ghazali, The design of new magnetic-photocatalyst nanocomposites (CoFe2O4-TiO2) as smart nanomaterials for recyclable-photocatalysis applications, New J. Chem., 2016, 40, 1124–1136 RSC.
- P. Xiong, L. J. Wang, X. Q. Sun, B. H. Xu and X. Wang, Ternary titania-cobalt ferrite-polyaniline nanocomposite: a magnetically recyclable hybrid for adsorption and photodegradation of dyes under visible light, Ind. Eng. Chem. Res., 2013, 52, 10105–10113 CrossRef CAS.
- A. Brindha and T. Sivakumar, Visible active N, S co-doped TiO2/graphene photocatalysts for the degradation of hazardous dyes, J. Photochem. Photobiol., A, 2017, 340, 146–156 CrossRef CAS.
- Q. W. Huang, S. Q. Tian, D. W. Zeng, X. X. Wang, W. L. Song, Y. Y. Li, W. Xiao and C. S. Xie, Enhanced photocatalytic activity of chemically bonded TiO2/graphene composites based on the effective interfacial charge transfer through the C–Ti bond, ACS Catal., 2013, 3(7), 1477–1485 CrossRef CAS.
- P. Wang, J. Wang, T. S. Ming, X. F. Wang, H. G. Yu, J. G. Yu, Y. G. Wang and M. Lei, Dye-sensitization-induced visible-light reduction of graphene oxide for the enhanced TiO2 photocatalytic performance, ACS Appl. Mater. Interfaces, 2013, 5(8), 2924 CrossRef CAS PubMed.
- H. Zhang, X. J. Lv, Y. M. Li, Y. Wang and J. H. Li, P25-graphene composite as a high performance photocatalyst, ACS Nano, 2010, 4(1), 380–386 CrossRef CAS PubMed.
- W. Qian, P. A. Greaney, S. Fowler, S. K. Chiu, A. M. Gafoth and J. Jiao, Low-temperature titrogen doping in ammonia solution for production of N-doped TiO2-hybridized graphene as a highly efficient photocatalyst for water treatment, ACS Sustainable Chem. Eng., 2014, 2(7), 1802–1810 CrossRef CAS.
- C. J. Leng, J. H. Wei, Z. Y. Liu, R. Xiong, C. X. Pan and J. Shi, Facile synthesis of PANI-modified CoFe2O4-TiO2, hierarchical flower-like nanoarchitectures with high photocatalytic activity, J. Nanopart. Res., 2013, 15(5), 1–11 CrossRef.
- C. Di Valentin, G. Pacchioni, A. Selloni, S. Livraghi and E. Giamello, Characterization of paramagnetic species in N-doped TiO2 powders by EPR spectroscopy and DFT calculations, J. Phys. Chem. B, 2005, 109(23), 11414–11419 CrossRef CAS PubMed.
- S. Pany, B. Naik, S. Martha and K. Parida, Plasmon induced nano Au particle decorated over S,N-modified TiO2 for exceptional photocatalytic hydrogen evolution under visible light, ACS Appl. Mater. Interfaces, 2014, 6(2), 839–846 CrossRef CAS PubMed.
|
This journal is © The Royal Society of Chemistry 2019 |