DOI:
10.1039/C8RA10165E
(Paper)
RSC Adv., 2019,
9, 3030-3040
Comparison of transition metal (Fe, Co, Ni, Cu, and Zn) containing tri-metal layered double hydroxides (LDHs) prepared by urea hydrolysis†
Received
11th December 2018
, Accepted 16th January 2019
First published on 22nd January 2019
Abstract
This paper details a successful synthesis and comparison of a range of tri-metal hydrotalcite-like layered double hydroxides (LDHs) using urea hydrolysis. Transition-metal-substituted MgMAl-LDHs were synthesized with M = Fe, Co, Ni, Cu or Zn. 5 mol% and 10 mol% substitutions were performed, where Mg was substituted with Co, Ni, Cu and Zn, and Al with Fe. The successful synthesis of crystalline MgMAl-LDHs was confirmed using X-ray powder diffraction (XRD) analysis. Energy-dispersive X-ray (EDX) spectroscopy was used to identify substituted metals and determine changes in composition. Changes in morphology were studied using scanning electron microscopy (SEM). Thermogravimetric analysis was used to determine the effect of Fe-, Co-, Ni-, Cu- or Zn-substitution on the thermal degradation of the MgMAl-LDH phase. The structure, morphology and thermal behavior of the LDHs were shown to be influenced by the substituted transition metals. The observed thermal stability took the order MgNiAl- > MgFeAl- = MgAl- ≥ MgCoAl- > MgCuAl- > MgZnAl-LDH. The urea hydrolysis method was shown to be a simple preparation method for well-defined crystallite structures with large hexagonal platelets and good distribution of transition metal atoms in the substituted LDHs.
Introduction
LDHs – known as hydrotalcite-like materials – are anionic clays with the formula |
[M1−x2+Mx3+(OH)2]x+·[(An−)x/n·yH2O]x−
| (1) |
where M2+, M3+ and An− are divalent metal cations, trivalent metal cations and interlayer anions respectively.1,2 The study of these materials has increased due to their wide range of applications and tunable properties.3 The most studied applications of LDHs include their use as antioxidants,4 stabilizers5,6 and flame retardants for polymers,7 photo-catalytic materials,8 and as UV-Vis absorption materials.9 The possibility to intercalate organic compounds into the layers of the LDHs also make them useful for multifunctional applications, such as usage in dye sensitized solar cells, enhancement of flame retardant properties, and resistance to thermal degradation.10–16 Most recently, the development of novel layered materials attracts great interest in various fields.17–21
There exist many techniques to prepare LDHs, the most common being co-precipitation, urea hydrolysis, ion exchange and hydrothermal synthesis.2,22–24 LDHs can be prepared with a variety of metal combinations that can be tailored to the desired applications and can have different structural, chemical and photoelectric properties dependent on the incorporated metals and preparation methods.25–30 It has been found that the inclusion of a third metal into the layer structure can modify the LDHs optical properties, UV-Vis light absorption range and catalytic properties.31,33
Kang et al. investigated how the catalytic oxidation of SO2 to SO3 can be improved through substitution of Fe and Mn in co-precipitated MgAl-LDHs.32 Jabłońska et al. prepared MgCuFe-LDHs using co-precipitation and characterized the materials for use as catalysts for the selective catalytic oxidation of ammonia to nitrogen and water vapor.34 Basąg et al. synthesized CuMgAl mixed metal oxides derived from LDHs through co-precipitation and studied these as catalysts for the selective oxidation of ammonia to dinitrogen. They concluded that the different molar ratios and calcination temperatures had an effect on the catalytic activity of the LDHs.35 Wang et al. synthesized Zn-doped MgAl-LDHs through modified homogeneous co-precipitation and studied the effect of thermal ageing on PVC. It was found that Zn-based MgAl-LDHs enhance the thermal stability of PVC.36 Chmielarz et al. synthesized MgAl, CuMgAl and FeMgAl mixed metal oxides derived from co-precipitated LDHs and doped the catalysts with noble metals. The catalytic oxidation of ammonia to nitrogen was studied.37 Parida et al. synthesized a ternary series of (Mg/Al + Fe)–CO3 LDHs by co-precipitation and tested them in the photocatalytic hydrogen generation from water.31 Kovanda et al. prepared CuMgAl-LDHs with different molar ratios via co-precipitation and studied their catalytic activity in toluene combustion.38 Chmielarz et al. studied the influence of Cu, Co and Ni cations incorporated into hydrotalcite on the thermal decomposition of the material. The LDHs were synthesized using co-precipitation and the thermal behavior was shown to be influenced by the amount of Cu, Co and Ni substituted.39 Kim et al. prepared ternary ZnAl-LDHs substituted with Co and Cu through co-precipitation and studied their photocatalytic effects in dye degradation.40 Liu et al. studied FeCoAl- and ZnCoAl-LDHs with different metal ratios. Well defined crystals were formed.41 Zheng et al. synthesized ZnMgAl-LDHs using a modified urea hydrolysis method and studied how the concentration of Zn affects the adsorption of methyl orange onto ZnMgAl-LDHs.42 Chagas et al. synthesized MgCoAl- and NiCoAl-LDHs and characterized them using a variety of techniques.43 Sakr et al. synthesized MgZnAl-LDHs using a modified urea hydrolysis method and discussed how different Zn concentrations can affect the capturing of carbon dioxide from methane streams.44 Labuschagné et al. investigated the heat stabilization of flexible PVC with 25 mol% transition metal substituted MgFeAl-, MgCuAl-, and MgZnAl-LDH, synthesized using a hydrothermal method. They found that the inclusion of Zn in the MgAl-LDH improved the color retention and that MgCuAl-LDH acted as an HCl scavenger.45
These investigations are illustrative of the synthesis routes applied to tri-metal LDHs and the applications for which they have been investigated. However, as evident from the overview, and illustrated in Table 1, the synthesis of these materials has been dominated by co-precipitation. In addition to this, the influence of transition metal content on the morphology and thermal degradation of LDHs has not yet been thoroughly investigated – importantly, a comparison between the different transition-metal-substituted MgAl-LDH synthesized at the same conditions is lacking.
Table 1 Previously reported tri-metal LDHs using urea hydrolysis and co-precipitation where a range of metal ratios was investigated (subs. M, M = substituted metal)
LDH |
Subs. M |
Variations |
Method |
Characterization methods |
Ref. |
(Mg/Al + Fe)–CO3 |
Fe |
Mg : (Al + Fe) = 2 : 1 |
Co-precipitation |
PXRD, FTIR, TGA, DTA, UV-Vis, BET, TEM, XPS |
31 |
MgAlFe, MnMgAlFe |
Fe, Mn |
0% wt, 1% wt, 5% wt and 10% wt |
Co-precipitation |
XRD, FTIR, TGA, DSC, catalytic test |
32 |
MgCuFe |
Cu |
2 : 1 : 1, 2 : 0.5 : 1 and 2 : 0 : 1 |
Co-precipitation |
XRD, catalytic tests |
34 |
CuMgAl |
Cu |
5 mol% Cu, temperature variations (600 °C, 700 °C and 800 °C) |
Co-precipitation |
XRD, FTIR, EPMA, TGA-DTG-QMS, BET, catalytic tests, DRUV-Vis |
35 |
MgZnAl |
Zn |
2 : 0 : 1, 1.7 : 0.3 : 1.0, 1.5 : 0.5 : 1.0, 1.3 : 0.7 : 1.0, 1.0 : 1.0 : 1.0 |
Homogeneous Co-precipitation |
XRD, FTIR, TEM, SEM, TG-DTA |
36 |
MgAl, CuMgAl, FeMgAl |
Cu, Fe |
71 : 29, 5 : 66 : 29, 5 : 66 : 29 (atomic ratios) |
Co-precipitation |
XRD, UV-vis-DRS, TG-DTA-QMS, STEM, BET, redox properties |
37 |
CuMgAl |
Cu |
0 : 4 : 2, 1 : 3 : 2, 2 : 2 : 2, 3 : 1 : 2, and 4 : 0 : 2 |
Co-precipitation |
XRD, TG, DTA, BET, TPD, catalytic activity |
38 |
MMgAl–CO3–NO3 |
Cu, Co, Ni |
M : Mg (0.10 : 0.61) and M : Al (0.10 : 0.29) |
Co-precipitation |
High temperature XRD, TGA-DTA-MS, TPR |
42 |
ZnAl, ZnCuAl, ZnCoAl |
Cu, Co |
2 : 1, 1.9 : 0.1 : 1, 1.75 : 0.25 : 1, 1.5 : 0.5 : 1, 1 : 1 : 1 |
Co-precipitation |
XRD, FTIR, Raman spectroscopy, UV-Vis, TEM, photocatalytic activity |
40 |
MgCoAl, MgNiAl, MgCuAl |
Co, Cu, Ni |
M(II) : Mg(II) : Al(III) 1 : 6 : 2 |
Co-precipitation |
XRD, XRF, TG MSD, FTIR and Raman spectroscopy |
46 |
MgAl, MgCoAl, MgNiAl, MgFeAl |
Fe, co, Ni |
MgCl2·6H2O = 0.29AlCl3·6H2O = 0.1, CoCl2 = 0.01, FeCl2·4H2O = 0.01, NiCl2·6H2O = 0.01 |
Co-precipitation |
PXRD, EXAFS, XANES, FTIR, SEM-EDX, TGA |
47 |
MgCoAl, NiCoAl |
Mg, Ni |
Molar ratios M2+ : M3+ of 2 (x = 1 : 3) |
Urea hydrolysis |
XRD, NMR, TGA-DTA, FTIR, SEM and N2 adsorption–desorption |
43 |
ZnMgAl |
Zn |
(Zn + Mg) : Al = 2 : 1, Zn/Mg = 0, 0.125, 0.5 and 0.8 |
Modified urea hydrolysis with co-precipitation |
XRD, SEM, FTIR, UV-Vis, AAS |
42 |
MgZnAl |
Mg, Zn |
((Mg + Zn)/Al molar ratio = 5.67), MgZn (25 : 75) Al, MgZn (50 : 50) Al, MgZn (75 : 25) Al |
Modified urea hydrolysis with co-precipitation |
XRD, FTIR, TGA, DTA, elemental analysis, SEM and TEM |
44 |
FeCoAl, ZnCoAl |
Fe, Zn |
M/(M + Co) = 0.8, 0.5, 0.2 |
Urea hydrolysis |
XRD, SEM and UV-Vis |
41 |
MgFeAl, MgCuAl, MgZnAl |
Fe, Cu, Zn |
25 mol% |
Hydrothermal |
XRD, SEM, BET, particle size, ICP-OES, FTIR, TGA, dynamic heat stability, thermal stability |
45 |
Although infrequently used for tri-metal LDH synthesis (as shown in Table 1) urea hydrolysis can provide LDHs with large, thin platelets and narrow particle size distributions. This stands in contrast to the LDHs synthesized by co-precipitation, which typically require extensive ageing to have similar characteristics. LDHs containing the transition metals Fe, Co, Ni, Cu and Zn as substituted metals in MgAl-LDH, have – to our knowledge – not been investigated comprehensively. It is, therefore, the aim of this paper, to provide the reader with a method to synthesize MgMAl-LDHs and compared the properties with M = (Fe, Co, Ni, Cu, Zn) using urea hydrolysis. Furthermore, this paper serves to illustrate the changes in crystallinity and morphological- and thermal properties between the different transition-metal-substituted LDHs as well as with an increase in the transition metal content.
Experimental techniques and characterization
Materials
Chemically pure (CP) or analytical grade (AR) reactants were used for all experiments without further treatment. Mg(NO3)2·6H2O, Al(NO3)3·9H2O, Fe(NO3)3·9H2O, Co(NO3)2·6H2O, Ni(NO3)2·6H2O, Cu(NO3)2·3H2O, Zn(NO3)2·6H2O were purchased from ABCR. Urea was purchased from Sigma Aldrich. Distilled water was used for all experiments.
Synthesis of LDHs
MgMAl-LDHs were synthesized using urea hydrolysis as explained in literature.22 A MII+
:
MIII+ molar ratio of 2
:
1 was used for all LDHs. The transition metals were substituted as follows: M/(Mg + M) = 0.05, 0.1 for Co, Ni, Cu and Zn and M/(Al + M) = 0.05, 0.1 for Fe (all on a molar basis). Salt solutions of Mg(NO3)2·6H2O and Al(NO3)3·9H2O with the required amounts of Fe, Co, Ni, Cu and Zn salts were prepared in distilled water, mixed in a round bottom flask, and heated to 100 °C, at which temperature they were kept for 48 h. After the reaction mixture was cooled down to room temperature, the slurry was filtered and washed with distilled water. The filter cake was dried in an oven at 70 °C for 24 h. The synthesized LDHs will be designated the following naming convention: MgMAl-x with M = Fe, Co, Ni, Cu, Zn and x = 5 (5% molar substitution) and 10 (10% molar substitution) of Mg or Al.
Characterization methods
X-ray diffraction measurements were performed on a Panalytical X'Pert PRO X-ray diffractometer in θ–θ configuration, equipped with a Fe filtered Co-Kα radiation (1.789 Å) and with an X'Celerator detector and variable divergence- and fixed receiving slits. Samples were prepared according to the standardized Panalytical backloading system, which provides nearly random distribution of the particles. The data was collected in the angular range 5° ≤ 2θ ≤ 80° with a step size 0.008° 2θ and a 13 s scan step time. The phases were identified using X'Pert Highscore plus software. Scanning electron microscopy (SEM) images were taken with a Zeiss Ultra Plus. Energy dispersive X-ray Spectroscopy (EDX) was done with a QUANTAX FlatQUAD from Bruker Nano GmbH. SEM samples were prepared by distributing the LDHs on a stub and sputter coating them with 3 nm of platinum. SEM micrographs were taken at 4.00 kV. For SEM-EDX analysis the samples were deposited on an Au-TEM-Grid with a 2 nm Au-holey-film. The samples were coated with carbon before measurement. EDX studies were performed with an accelerating voltage of 12 keV. Thermogravimetric analysis (TGA) was performed with a heating rate of 10 °C min−1 using a TGA Q5000 from TA instruments in an inert nitrogen atmosphere in the temperature range of 25–1000 °C. TGA measurements were performed three times and the mean value with the corresponding standard deviation is shown in the results.
Results and discussion
X-ray diffraction (XRD)
The XRD patterns of the synthesized LDHs are shown in Fig. 1(a and b). The reflections are narrow and very few to no contaminants or side products could be observed, indicating almost complete substitution of Fe, Co, Ni, Cu, and Zn into the crystalline MgAl-LDH structure.42,43 A high intensity for the reflections corresponding to the (003), (006), (009) and (110) planes of a rhombohedral crystal lattice could be observed (as expected for materials based on a brucite structure), indicating a high orderliness and crystallinity of the samples.5,48 High platelet crystallinity and orderliness is characteristic to the urea hydrolysis method used for synthesis.22
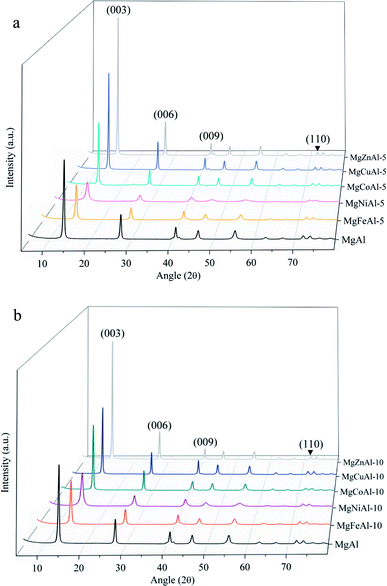 |
| Fig. 1 (a) XRD patterns obtained for the series of M = (Fe, Co, Ni, Cu, Zn) (5 mol%) substituted tri-metal MgMAl-LDHs and MgAl-LDH synthesized using urea hydrolysis. (b) XRD patterns obtained for the series of M = (Fe, Co, Ni, Cu, Zn) (10 mol%) substituted tri-metal MgMAl-LDHs and MgAl-LDH synthesized using urea hydrolysis. | |
Clear changes in reflection intensity can be seen in Fig. 1(a and b). Apart from the Ni-substituted LDHs, the reflection intensity of the samples increased moving right on the periodic table. Because of phenomenon's such as constructive interference and preferred crystallite orientation observable in clays which can alter the sample reflection intensities,49 an increase in reflection intensity cannot directly be related to an increase in sample crystallinity. An example of this would be a comparison of intensities between 5%- and 10% substituted LDHs as produced in this study. It is, however, expected that significantly increased reflection intensities as seen upon moving right on the periodic table upon 5 mol% and 10 mol% transition metal substitution in the LDHs (apart from Ni) can be correlated to increases in the sample crystallinity.
As the substitution increases, an amorphous phase can form, which, dependent on the amount of amorphous material present, can hinder further growth of crystals and in turn reduce the crystallinity of the LDHs.31,39 SEM micrographs showed small amounts of unidentified material on the LDH platelets which we believe to be amorphous material and which will be designated as residue from hereon. The residue material is believed to consist of oxides of the metals used for synthesis. These phases would, due to the low intensity and broadened nature of their diffraction patterns, remain undetected (if their quantities are small enough)31 or be detectable as amorphous halo's on the XRD graphs (if present in large amounts). It was, therefore, reasoned, that these phases existed in very small amounts that did not influence the XRD patterns substantially, and that the samples produced consisted mainly of the desired LDH phase. A schematic representation of the substitution of the transition metals into the LDH structure is shown in Fig. 2.
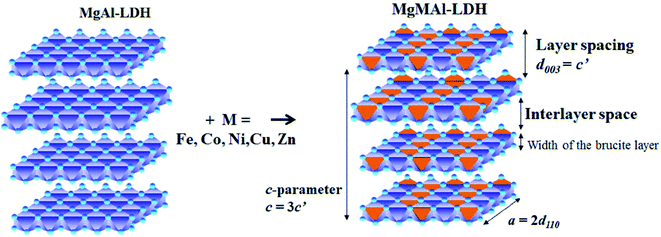 |
| Fig. 2 Schematic representation of di-metal (MgAl) and tri-metal layered double hydroxides (LDHs)(MgMAl) where M = Fe, Co, Ni, Cu, Zn adapted from (ref. 17). | |
The layer spacing (d-spacing) can be modified by changes in the anion or water content in the interlayer or changes in the LDH layer composition. The isomorphic replacement of Mg2+ with M = (Co, Ni, Cu, Zn) and Al3+ with M = (Fe) leads to a change in layer spacing if the ionic radius of the substituted metal is different to that of Mg or Al. This is observed by a shift in reflections on the XRD pattern against those obtained for plain MgAl-LDH and is regarded as an indication of the successful substitution of the transition metals.31,32,50 Dependent on the radius of the substituted ion, the reflections shift to the left when a larger cation is substituted and to the right when a smaller cation is substituted.
The ionic radii used in the following discussion were based on a rhombohedral crystal lattice structure with VI coordination. Changes in layer distance were observed for Fe- and Ni-substitution. The layer distances of the Co-, Cu- and Zn-substituted LDHs remained equal or similar to those obtained for MgAl-LDH. The substitution of Al3+ by Fe3+ increased the d(003) value from 7.547 Å to 7.572 Å, as a possible result of the large difference in ionic radius of Al3+ (0.535 Å) and Fe3+ (0.645 Å).31 The substitution of Mg2+ (0.72 Å) with Ni2+ (0.69 Å) increased the layer distance from 7.547 Å to 7.609 Å even though the Ni cation is slightly smaller than the Mg cation. It is possible that the increase in layer spacing was observed due to an increase in interlayer water. No change in layer distance was observed for Co2+ (0.745 Å), Zn2+ (0.74 Å) and Cu2+ (0.73 Å) substitution for Mg2+ (0.72 Å), which corresponds well to the fact that all four metals have a similar ionic radius. Changes in c and a parameters (where the ionic radius of the substituted metal is similar) can be ascribed to changes in interstitial water content or anion orientation/ordering. Changes in interlayer water content would be visible in the TGA curves of the sample through a peak shift in the differential curve between 150 °C and 200 °C against MgAl-LDH and will be further discussed in the TGA section.
Table 2 shows the positions of the basal planes (003), (006), (009) and (110), the corresponding d-spacing and crystal parameters as well as the estimated crystallite size (L). Table 2(b)† shows the crystal parameters for the 10% substituted LDHs and can be found in the ESI. The d-spacings were calculated using Bragg's Law. Crystal parameters c and a were calculated with
and a = 2d110. Crystallite thicknesses (based on the (003) reflection) were estimated using the Scherrer equation
where L is the mean size of crystalline domains parallel to the lattice plane, λ is the wavelength, K is a shape factor of 0.9 and β is the line broadening at half maximum (FWHM) of the diffraction peak. Compared to MgAl-LDH, the crystallite thickness increased for MgMAl-LDH with M = Co, Cu and Zn and decreased for MgMAl-LDH with M = Fe and Ni.
Table 2 Peak positions for the (003), (006) and (009) planes, interlayer distance (d003), crystal lattice parameters (c and a) and Crystallite sizes of the MgMAl-LDHs with M = (Fe, Co, Ni, Cu, Zn) for 5% molar substitutions synthesized using urea hydrolysis
Sample ID |
2θ (003) (°) |
2θ (006) (°) |
2θ (009) (°) |
2θ (110) (°) |
d(003) (Å) |
c (Å) |
a (Å) |
D (nm) |
MgAl |
13.613 |
27.451 |
40.893 |
72.242 |
7.547 |
22.769 |
3.035 |
28.2 |
MgFeAl-5 |
13.569 |
27.385 |
40.870 |
72.155 |
7.572 |
22.815 |
3.038 |
20.8 |
MgCoAl-5 |
13.635 |
27.517 |
40.848 |
72.111 |
7.535 |
22.747 |
3.040 |
33.7 |
MgNiAl-5 |
13.503 |
27.319 |
40.826 |
72.132 |
7.609 |
22.878 |
3.039 |
11.4 |
MgCuAl-5 |
13.635 |
27.495 |
40.827 |
72.088 |
7.535 |
22.757 |
3.040 |
39.7 |
MgZnAl-5 |
13.635 |
27.473 |
40.783 |
72.067 |
7.535 |
22.771 |
3.041 |
39.5 |
Although the crystallite thickness cannot necessarily give a direct correlation to the platelet diameter, the crystallite thicknesses show the same trend as seen for the atomic radii, whereas the calculated d-spacing shows the opposite trend (Fig. 3(b) for divalent transition metal substituted LDHs). It might be possible that this trend could give information regarding the number of layers of the LDH platelet with a change in transition metal – here a potential decrease in the number of layers in the order Zn > Cu > Co > Mg > Ni. It was also interesting to observe that the estimated crystallite thickness corresponded well to the observed platelet sizes in SEM. Correlations have previously been found by Galvão et al.51
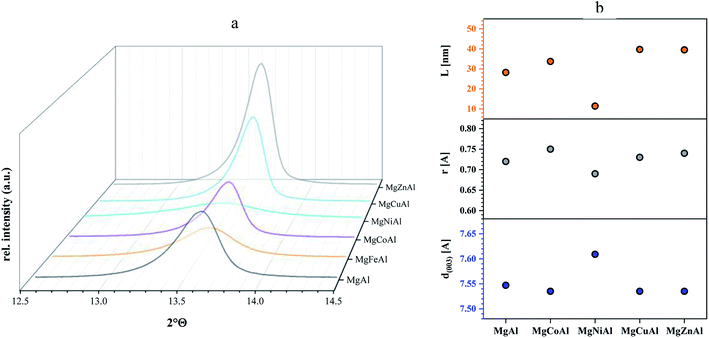 |
| Fig. 3 (a) Detailed XRD pattern of reflex (003) for MgAl-LDH and MgMAl-LDH with M = Fe, Co, Ni, Cu, Zn (5 mol% sub.). (b) Correlation of atomic radii with d-spacing and crystallite size of MgAl-LDH substituted by 5% di-valent transition metals. | |
Scanning electron microscopy (SEM)
Fig. 4 shows the SEM images of the synthesized MgAl- and the MgMAl-LDHs. All samples showed growth of the characteristic hexagonal platelets. The platelets were large and thin (except those obtained for Ni- and Fe-substitution) as expected for LDHs synthesized using the urea hydrolysis method. The large dimensions of the platelets grown, stand in contrast to the small platelet size and low crystallinity of LDHs synthesized using co-precipitation. The large platelet size is a direct result of the time dependency of the hydrolysis of the urea present in the solution, which remains supersaturated to a low degree throughout precipitation.22 The morphologies of the different transition metal substituted LDHs varied. Synthesis condition and nature of transition metal have effect on the morphology of LDHs as discussed previously. As the substitution of transition metal varied the morphology is different of MgAl LDHs.12,26 The best defined platelet structure was obtained for plain MgAl-LDH. The platelet size decreased upon Fe- and Ni-substitution and increased upon Cu-, Co- and Zn-substitution.
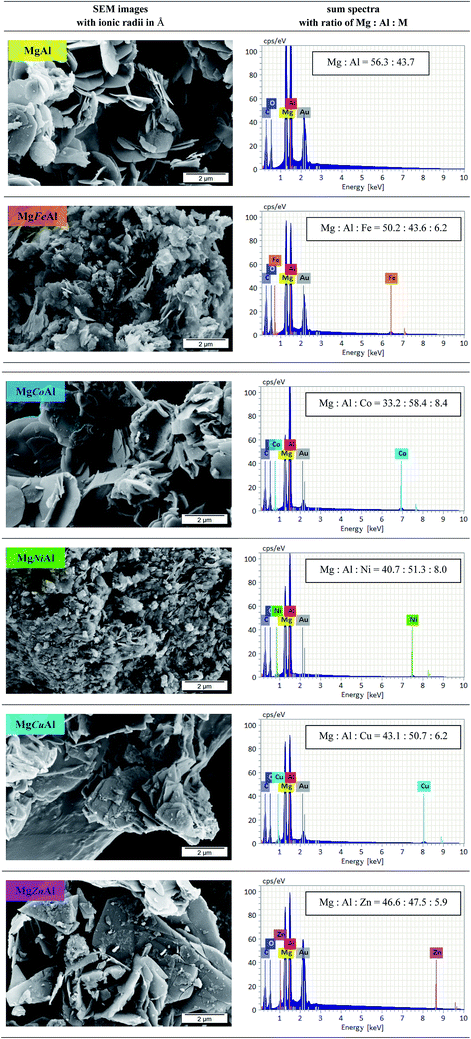 |
| Fig. 4 SEM images of MgAl-LDH and MgMAl-LDH with M = Fe, Co, Ni, Cu, Zn (left) with the corresponding sum spectra and calculated atomic ratios of Mg : Al : M (right). | |
Energy dispersive X-ray spectroscopy (EDX)
The EDX results (sum spectra with calculated atomic ratios of Mg
:
Al
:
M) are also shown in Fig. 4. Using EDX, SEM and XRD analysis, it could be shown that a good distribution of the transition metals in the LDHs layers could be achieved bar small amounts of residue material on the LDHs platelets. Due to the substitution of the transition metals, the ratio of Mg
:
Al was changed. The content of Mg decreased, whereas the amount of Al increased compared to the ratio obtained for MgAl-LDH. For the divalent transition metal substitution, the following order was found to apply for the observed Mg content: MgAl- > MgZnAl- > MgCuAl- > MgNiAl- > MgCoAl-LDH. The opposite order was observed for the Al content of the investigated sample area. The amount of the transition metal successfully incorporated into the LDH layers followed the sequence Co > Ni > Cu > Zn. Furthermore, local variations in Co distribution were observed. We believe that the decrease in Co-substitution occurred due to the formation of a layered α-Co(OH)2 side-species with interstitially substituted Mg. The synthesis and application of α-Co(OH)2 was studied by Rajamathi, Kamath and Seshadri (2000) and Leng et al., who obtained XRD patterns very similar to those of the MgCoAl-LDH phases in this work.52,53
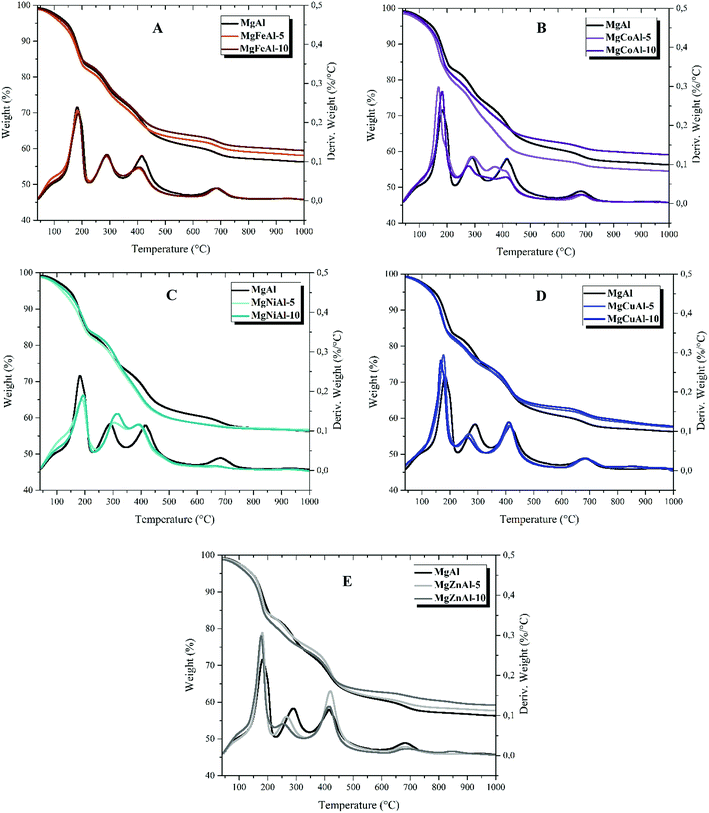 |
| Fig. 5 TGA curves of 5% and 10% substituted tri-metal MgMAl-LDHs for the series of M = (Fe, Co, Ni, Cu, Zn), (A) MgFeAl-LDHs, (B) MgCoAl-LDHs (C), MgNiAl-LDHs, (D) MgCuAl-LDHs and (E) MgZnAl-LDHs synthesized using urea hydrolysis. | |
Thermogravimetric analysis (TGA)
The results of the thermal analysis obtained for the series of M = (Fe, Co, Ni, Cu, Zn) substituted tri-metal MgMAl- synthesized LDHs are shown in Fig. 5 and Table 3 (see ESI†). The series of LDHs decomposed in five stages with a total mass loss of up to 50%. The weight loss as a function of temperature is shown in Fig. 5. The obtained TGA results show a stepwise profile with five temperature regions. The first temperature region lies between 50 °C to 250 °C, the second between 250 °C and 350 °C, the third between 350 °C and 500 °C, the fourth between 500 °C to 700 °C, and the fifth between 700 °C and 1000 °C. For temperatures up to 100 °C, adsorbed water and gases are lost. The mass loss between 170 °C and 250 °C corresponds to the loss of interlayer water molecules. Between 250 °C and 500 °C the major product is CO2 from interlayer CO32− and water vapor formed from the dehydroxylation of OH-groups. At higher temperatures metal oxides of the LDH phase formed.37,39,54,55 The mass loss profile and decomposition stages are summarized in Table 3.† Table 3 shows the residual weight after TGA.
Table 3 Residual weight after TGA for different MgMAl LDHs with M = (Fe, Co, Ni, Cu, Zn) (5 mol% and 10 mol% subs.)
LDHs |
Residual weight left (%) |
5 mol% |
10 mol% |
MgAl |
55.2 ± 2.93 |
55.2 ± 2.93 |
MgFeAl |
58.6 ± 0.43 |
59.9 ± 0.46 |
MgCoAl |
55.0 ± 0.26 |
59.2 ± 0.69 |
MgNiAl |
56.6 ± 0.49 |
57.1 ± 0.15 |
MgCuAl |
58.4 ± 0.60 |
58.8 ± 0.63 |
MgZnAl |
57.9 ± 0.55 |
59.5 ± 0.78 |
The substitution of the different transition metals resulted in differences in the thermal decomposition behavior of the LDHs as can be seen in Fig. 5. Differences in thermal stability could be observed between all LDHs analyzed. It was found that the presence of different transition metals in the layers of MgAl-LDHs reduced the total weight loss. Only the 5 mol% Co-substitution increased the weight loss with respect to MgAl-LDH as can be seen in Fig. 5(B) and in Table 3.† Furthermore, it was observed that an increase in the concentration of the transition metal from 5 mol% to 10 mol% led to a decrease in total weight loss in all tested LDHs apart from MgNiAl-LDH (Fig. 5(C)). Differences in total weight loss can be explained by differences in atomic weight and concentration effects as well as changes in intermolecular forces. Finally, it could be shown that the decomposition of the LDH structure – based on the removal of interlayer carbonates (peak 2 in the DTA profile) – decreased according to MgNiAl- > MgFeAl- = MgAl- ≥ MgCoAl- > MgCuAl- > MgZnAl-LDH. An increase in transition metal content led to an increase in stability for Ni-substitution and a decrease in stability for Cu- and Zn-substitution with respect to the lower-substituted LDHs.
MgFeAl-LDH – Fig. 5(A). For Fe-substitution, the total weight loss decreased from 43.1% (MgAl-LDH) to 40.65/39.7% (MgFeAl-5/MgFeAl-10). First (up to 184/186 °C (MgFeAl-5/MgFeAl-10)), adsorbed water and gases as well as interlayer water were lost. Interlayer carbonates were decomposed up to 288/290 °C (MgFeAl-5/MgFeAl-10), and the LDH structure dehydroxylated up to 415/405 °C (MgFeAl-5/MgFeAl-10). Finally, the LDH phase was transformed to mixed oxide phases, as the hydrotalcite phase does not exist above 500 °C. Weight loss observed at higher temperatures (around 700 °C) could also have occurred as a result of the formation of Fe2O3.31,37,47
MgCoAl-LDH – Fig. 5(B). The Co-substituted LDHs provided different results to those obtained for the other LDHs. Here the weight loss of the 5 mol% substituted LDH (MgCoAl-5) was 44.0%, higher than that of MgAl-LDH with 43.1%. A decrease in total weight loss with respect to MgAl-LDH to 39.9% was observed for MgCoAl-10. Up to 169/183 °C, adsorbed water and gases as well as interlayer water was lost. Interlayer carbonates were decomposed up to 291/277 °C and the LDH structure dehydroxylated up to 372-410/410 °C. Further weight loss at higher temperatures again occurred due to the formation of mixed metal oxides from the LDH phase.39 Similar results were obtained in the study of the influence of Co-substitution in MgAl-LDHs.39,43,47
MgNiAl-LDH – Fig. 5(C). For Ni-substitution, the total weight loss decreased to 41.9/42.4% from 43.1%. MgNiAl-LDH showed a sharp increase in weight loss between 200 °C and 400 °C. Interlayer water and adsorbed gases were lost until 190/195 °C. Interlayer carbonates were decomposed up to 304/315 °C and the LDH structure dehydroxylated up to 390/392 °C. Decomposition of hydroxyl groups and carbonate anions occurred until 668/658 °C. Further weight loss at higher temperatures around 700 °C in Fig. 5(C) occurred due to the formation of transition metal oxides. Similar thermal decomposition behavior was observed by Chmielarz et al. and Curtius et al.39,47
MgCuAl-LDH – Fig. 5(D). The total weight loss for the Cu-substituted LDHs was the same for both substitution percentages (41.7%) and less than that of MgAl-LDH (43.1%). In Fig. 5(D), it can be seen that the interlayer water was lost at lower temperatures compared to MgAl-LDH. Adsorbed water and gases as well as interlayer water were lost until 177/168 °C. Interlayer carbonates were decomposed up to 262/268 °C and the LDH structure dehydroxylated up to 413/410 °C. The peak at 849 °C in the case of 5 mol% Cu-substituted and 858 °C in the case of the 10 mol% Cu-substituted LDHs could have occurred due to the thermal reduction of Cu. Similar behavior was observed at 860 °C in a previous study by Chmielarz et al., who synthesized CuMgAl-LDH using co-precipitation.39 It seems that Cu is responsible for stabilization of MgAl-LDHs.
MgZnAl-LDH – Fig. 5(E). The total weight loss for the Zn-substituted LDHs decreased to 41.5/39.5% from 43.1% for MgAl-LDH. The substituted LDHs decomposed at lower temperatures than MgAl-LDH. Adsorbed water and gases as well as interlayer water were lost up to 181/178 °C. Interlayer carbonates were decomposed up to 267/254 °C and the LDH structure dehydroxylated up to 420/415 °C. Further weight loss at higher temperatures around 700 °C occurred due to the formation of a mixed oxide phase. Wang et al. and Sakr et al. studied the synthesis of ZnMgAl-LDHs but did not discuss the effect of the substitution on their thermal decomposition.36,44As a comparison it can be seen that substitution of different transition metals in MgAl-LDHs have effect on thermal stability of MgAl LDHs. Different morphology and structure can also be observed from the XRD and SEM analysis for different substitution of transition metal in MgAl LDHs.
Comparison of theoretical residual weight and measured residual weight of different LDHs
The residual weight after complete calcination of LDHs (into a mixed oxide) can be calculated from eqn (2) as previously given by Forano et al.56 The decomposition follows approximately through this equation, although other decomposition phases are possible dependent on the composition of LDHs. For the carbonate intercalated LDHs investigated in this article the anion decomposition product is expected to be CO2. |
 | (2) |
It is assumed that eqn (2) can also be used when calculating the residual weights of the synthesized tri-metal LDHs – if similar decomposition products are formed. A comparison between theoretical residual weights calculated under this assumption and the measured residual weights obtained through TGA is presented in Table 4. No large deviations in residual weight from the theoretical values were observed in any of the samples.
Table 4 Thermogravimetric analysis data of MgMAl LDHs with M = (Fe, Co, Ni, Cu, Zn) synthesized using urea hydrolysis showing measured residue value and theoretically calculated reside value
LDHs |
Theoretical residue structure (mix oxides) |
Theoretical residue (%) |
Measured residue (%) |
Variance from theoretical residue (%) |
MgAl |
4MgO·Al2O3 |
57.1 |
56.9 |
0.2 |
MgFeAl-5 |
4MgO·(Al0.95Fe0.05)2O3 |
57.6 |
59.3 |
−1.7 |
MgFeAl-10 |
4MgO·(Al0.9Fe0.1)2O3 |
58.1 |
60.3 |
−2.2 |
MgCoAl-5 |
4(Mg0.95Co0.05)O·Al2O3 |
60.7 |
56.0 |
4.7 |
MgCoAl-10 |
4(Mg0.9Co0.1)O·Al2O3 |
61.3 |
61.1 |
0.2 |
MgNiAl-5 |
4(Mg0.95Ni0.05)O·Al2O3 |
60.7 |
58.1 |
2.6 |
MgNiAl-10 |
4(Mg0.9Ni0.1)O·Al2O3 |
61.3 |
57.6 |
3.7 |
MgCuAl-5 |
4(Mg0.95Cu0.05)O·Al2O3 |
60.8 |
58.3 |
2.5 |
MgCuAl-10 |
4(Mg0.9Cu0.1)O·Al2O3 |
61.5 |
58.3 |
3.5 |
MgZnAl-5 |
4(Mg0.95Zn0.05)O·Al2O3 |
60.8 |
58.5 |
2.3 |
MgZnAl-10 |
4(Mg0.9Zn0.1)O·Al2O3 |
61.5 |
60.5 |
1.0 |
No clear correlation between the residual weights and other analyses results were observed. However, there appears to be a correlation between the variance of the theoretical residual weight and measured residual weight (indicated as percentage variance from theoretical residue in Table 4) when compared to the incomplete substitution as observed through EDX analysis. From the results it appears that the lower the variance from the theoretical residual weight, the higher the substitution efficiency. If the atomic substitution did not occur as expected, it is likely that some of the expected LDH phase would not have formed (in favor of other metal complexes), thus leading to a decrease in the measured residual weight. In the case of trivalent metal substitution (partially replacing the Al with Fe), the measured residue was more than the postulated theoretical value. This may be attributed to the higher Mg content of the LDH phase or an increase in interstitial water content.
Conclusion
A comprehensive understanding of LDHs is important for their future applications as catalyst and flame retardants. Co-precipitation, hydrothermal and modified urea hydrolysis methods as well as a variety of metal combinations have been studied previously. In this study, the urea hydrolysis method was (for the first time comprehensively) used for the preparation of Fe-, Co-, Ni-, Cu- and Zn-substituted MgAl-LDH at the same synthesis conditions, allowing true comparison between the LDH properties with respect to morphology and changes in thermal decomposition behavior. The LDHs were studied using XRD, SEM, SEM-EDX and TGA.
Well defined crystals with hexagonal platelet structure and large platelet size were obtained. Good distribution of the transition metal in the MgAl-LDH layers could be achieved at 5 mol% substitution. The different substituted transition metals had a notable effect on the morphology and platelet size. The substitution percentage had a notable effect on thermal behavior. It might be valuable to further investigate the effect of an increasing substitution percentage in future. In conclusion, the urea hydrolysis method provides a simple one pot synthesis method for tri-metal LDHs that could find applications in different research areas. It is envisaged that other tri-metal combinations of LDHs could be synthesized using this method and their properties further investigated.
Conflicts of interest
There are no conflicts to declare.
Acknowledgements
We acknowledge the Leibniz Institut für Polymerforschung Dresden e. V., Germany for providing the facilities to do this research. Sajid Naseem is also thankful to the Higher Education Commission (HEC), Pakistan and German Academic Exchange Service (DAAD) for providing his scholarship. The authors would also like to thank Techsparks (Pty) Ltd and the Technology and Human Resources for Industry Programme (THRIP) administered by the Department of Trade and Industry, South Africa, (grant number THRIP/133/31/03/2016) for their contribution in terms of funding for powder X-ray diffraction analysis performed at the University of Pretoria, South Africa and a travel allowance for Bianca Gevers to visit the Institut für Polymerforschung Dresden e. V to work on this project. Bianca Gevers is also thankful to the Institut für Polymerforschung Dresden e. V. for providing her guest student stipend for the duration of her stay.
References
- Y. Kuang, L. Zhao, S. Zhang, F. Zhang, M. Dong and S. Xu, Materials, 2010, 3, 5220–5235 CrossRef CAS PubMed
. - In Modern Inorganic Synthetic Chemistry, ed. R. Xu and Y. Xu, Elsevier, Amsterdam, 2nd edn, 2017, pp. 493–543, DOI:10.1016/B978-0-444-63591-4.00018-5
. - J. Yu, Q. Wang, D. O'Hare and L. Sun, Chem. Soc. Rev., 2017, 46, 5950–5974 RSC
. - S. P. Lonkar, A. Leuteritz and G. Heinrich, RSC Adv., 2013, 3, 1495–1501 RSC
. - B. Kutlu, A. Leuteritz, L. Häußler, U. Oertel and G. Heinrich, Polym. Degrad. Stab., 2014, 102, 9–14 CrossRef CAS
. - S. Naseem, S. P. Lonkar, A. Leuteritz and F. J. W. J. Labuschagné, RSC Adv., 2018, 8, 29789–29796 RSC
. - Y. Gao, J. Wu, Q. Wang, C. A. Wilkie and D. O'Hare, J. Mater. Chem. A, 2014, 2, 10996–11016 RSC
. - S.-J. Xia, F.-X. Liu, Z.-M. Ni, W. Shi, J.-L. Xue and P.-P. Qian, Appl. Catal., B, 2014, 144, 570–579 CrossRef CAS
. - J. J. Reinosa, P. Leret, C. M. Álvarez-Docio, A. del Campo and J. F. Fernández, Bol. Soc. Esp. Ceram. Vidrio, 2016, 55, 55–62 CrossRef
. - F. L. Theiss, G. A. Ayoko and R. L. Frost, Appl. Surf. Sci., 2016, 383, 200–213 CrossRef CAS
. - R. Quispe-Dominguez, S. Naseem, A. Leuteritz and I. Kuehnert, RSC Adv., 2019, 9, 658–667 RSC
. - U. Costantino, F. Marmottini, M. Nocchetti and R. Vivani, Eur. J. Inorg. Chem., 1998, 1998, 1439–1446 CrossRef
. - E. N. Kalali, X. Wang and D.-Y. Wang, J. Mater. Chem. A, 2016, 4, 2147–2157 RSC
. - D. Basu, A. Das, D.-Y. Wang, J. J. George, K. W. Stockelhuber, R. Boldt, A. Leuteritz and G. Heinrich, RSC Adv., 2016, 6, 26425–26436 RSC
. - Y. Peng, W. Wang, J. Cao and Y. Huang, J. Appl. Polym. Sci., 2017, 134, 44597 Search PubMed
. - B. Nagendra, C. V. S. Rosely, A. Leuteritz, U. Reuter and E. B. Gowd, ACS Omega, 2017, 2, 20–31 CrossRef CAS
. - Y. Zhu, R. Zhu, G. Zhu, M. Wang, Y. Chen, J. Zhu, Y. Xi and H. He, Appl. Surf. Sci., 2018, 433, 458–467 CrossRef CAS
. - Y. Cao, P. Maitarad, M. Gao, T. Taketsugu, H. Li, T. Yan, L. Shi and D. Zhang, Appl. Catal., B, 2018, 238, 51–60 CrossRef CAS
. - J. An, L. Shi, G. Chen, M. Li, H. Liu, S. Yuan, S. Chen and D. Zhang, J. Mater. Chem. A, 2017, 5, 19738–19744 RSC
. - Z. Chen, Z. Tao, S. Cong, J. Hou, D. Zhang, F. Geng and Z. Zhao, Chem. Commun., 2016, 52, 11442–11445 RSC
. - G. Chen, J. An, Y. Meng, C. Yuan, B. Matthews, F. Dou, L. Shi, Y. Zhou, P. Song, G. Wu and D. Zhang, Nano Energy, 2019, 57, 157–165 CrossRef CAS
. - J. He, M. Wei, B. Li, Y. Kang, D. G. Evans and X. Duan, in Layered Double Hydroxides, ed. X. Duan and D. G. Evans, Springer Berlin Heidelberg, Berlin, Heidelberg, 2006, pp. 89–119, DOI:10.1007/430_006
. - J. S. Valente, M. S. Cantu and F. Figueras, Chem. Mater., 2008, 20, 1230–1232 CrossRef CAS
. - J. S. Valente, M. Sánchez-Cantú, E. Lima and F. Figueras, Chem. Mater., 2009, 21, 5809–5818 CrossRef CAS
. - D.-K. Cho, C.-W. Jeon and I.-K. Park, J. Alloys Compd., 2018, 737, 725–730 CrossRef CAS
. - J. J. Bravo-Suárez, E. A. Páez-Mozo and S. T. Oyama, Quim. Nova, 2004, 27, 601–614 Search PubMed
. - C. Forano, U. Costantino, V. Prévot and C. T. Gueho, in Developments in Clay Science, ed. F. Bergaya and G. Lagaly, Elsevier, 2013, vol. 5, pp. 745–782 Search PubMed
. - F. Cavani, F. Trifirò and A. Vaccari, Catal. Today, 1991, 11, 173–301 CrossRef CAS
. - S. MIYATA, Clays Clay Miner., 1980, 28, 50–56 CrossRef CAS
. - M. Pavlovic, P. Rouster, T. Oncsik and I. Szilagyi, ChemPlusChem, 2017, 82, 121–131 CrossRef CAS
. - K. Parida, M. Satpathy and L. Mohapatra, J. Mater. Chem., 2012, 22, 7350–7357 RSC
. - H. T. Kang, K. Lv and S. L. Yuan, Appl. Clay Sci., 2013, 72, 184–190 CrossRef CAS
. - J. Wang, T. Zhang, M. Li, Y. Yang, P. Lu, P. Ning and Q. Wang, RSC Adv., 2018, 8, 22694–22709 RSC
. - M. Jabłońska, L. Chmielarz, A. Węgrzyn and S. WITKOWSKI, Chemik, 2012, 66, 750–757 Search PubMed
. - S. Basąg, Z. Piwowarska, A. Kowalczyk, A. Węgrzyn, R. Baran, B. Gil, M. Michalik and L. Chmielarz, Appl. Clay Sci., 2016, 129, 122–130 CrossRef
. - G. Wang, M. Yang, Z. Li, K. Lin, Q. Jin, C. Xing, Z. Hu and D. Wang, J. Nanopart. Res., 2013, 15, 1882 CrossRef
. - L. Chmielarz, M. Jabłońska, A. Strumiński, Z. Piwowarska, A. Węgrzyn, S. Witkowski and M. Michalik, Appl. Catal., B, 2013, 130–131, 152–162 CrossRef CAS
. - F. Kovanda, K. Jirátová, J. Rymeš and D. Koloušek, Appl. Clay Sci., 2001, 18, 71–80 CrossRef
. - L. Chmielarz, P. Kuśtrowski, A. Rafalska-Łasocha and R. Dziembaj, Thermochim. Acta, 2002, 395, 225–236 CrossRef
. - S. Kim, J. Fahel, P. Durand, E. André and C. Carteret, Eur. J. Inorg. Chem., 2017, 2017, 669–678 CrossRef CAS
. - Z. Liu, R. Ma, Y. Ebina, N. Iyi, K. Takada and T. Sasaki, Langmuir, 2007, 23, 861–867 CrossRef CAS PubMed
. - Y.-M. Zheng, N. Li and W.-D. Zhang, Colloids Surf., A, 2012, 415, 195–201 CrossRef CAS
. - L. H. Chagas, G. S. G. De Carvalho, W. R. Do Carmo, R. A. S. San Gil, S. S. X. Chiaro, A. A. Leitão, R. Diniz, L. A. De Sena and C. A. Achete, Mater. Res. Bull., 2015, 64, 207–215 CrossRef CAS
. - A. A. E. Sakr, T. Zaki, O. Elgabry, M. A. Ebiad, S. M. El-Sabagh and M. M. Emara, Appl. Clay Sci., 2018, 160, 263–269 CrossRef CAS
. - F. J. W. J. Labuschagne, D. M. Molefe, W. W. Focke, I. van der Westhuizen, H. C. Wright and M. D. Royeppen, Polym. Degrad. Stab., 2015, 113, 46–54 CrossRef CAS
. - A. Tsyganok and A. Sayari, J. Solid State Chem., 2006, 179, 1830–1841 CrossRef CAS
. - H. Curtius, G. Kaiser, K. Rozov, A. Neumann and D. Kathy Dardenne, Clays Clay Miner., 2013, 61, 424–439 CrossRef CAS
. - N. Iyi and T. Sasaki, J. Colloid Interface Sci., 2008, 322, 237–245 CrossRef CAS PubMed
. - R. Dohrmann, K. B. Rüping, M. Kleber, K. Ufer and R. Jahn, Clays Clay Miner., 2009, 57, 686–694 CrossRef CAS
. - Database of Ionic Radii, http://abulafia.mt.ic.ac.uk/shannon/radius.php, accessed 23/07/2018.
- T. L. P. Galvão, C. S. Neves, A. P. F. Caetano, F. Maia, D. Mata, E. Malheiro, M. J. Ferreira, A. C. Bastos, A. N. Salak, J. R. B. Gomes, J. Tedim and M. G. S. Ferreira, J. Colloid Interface Sci., 2016, 468, 86–94 CrossRef PubMed
. - M. Rajamathi, P. V. Kamath and R. Seshadri, Mater. Res. Bull., 2000, 35, 271–278 CrossRef CAS
. - X. Leng, K.-H. Wu, Q. Zeng, I. R. Gentle and D.-W. Wang, Asia-Pac. J. Chem. Eng., 2016, 11, 415–423 CrossRef CAS
. - R. Chitrakar, S. Tezuka, A. Sonoda, K. Sakane and T. Hirotsu, Ind. Eng. Chem. Res., 2008, 47, 4905–4908 CrossRef CAS
. - V. R. Choudhary, R. Jha and P. A. Choudhari, J. Chem. Sci., 2005, 117, 635–639 CrossRef CAS
. - C. Forano, T. Hibino, F. Leroux and C. Taviot-Guého, in Developments in Clay Science, ed. F. Bergaya, B. K. G. Theng and G. Lagaly, Elsevier, 2006, vol. 1, pp. 1021–1095 Search PubMed
.
Footnote |
† Electronic supplementary information (ESI) available. See DOI: 10.1039/c8ra10165e |
|
This journal is © The Royal Society of Chemistry 2019 |