DOI:
10.1039/C8RA09419E
(Paper)
RSC Adv., 2019,
9, 1747-1751
Near-infrared emitting lanthanide(III) complexes as prototypes of optical imaging agents with peptide targeting ability: a methodological approach†
Received
15th November 2018
, Accepted 8th January 2019
First published on 14th January 2019
Abstract
A methodological approach to design prototypes of specific near-infrared emitting imaging agents based on a small molecular compound combining a lanthanide(III) ion, the cyclen derivative as a coordinating unit and the azo-dye as a sensitizer with a Arg-Gly-Asp cyclopeptide as a targeting moiety, is presented here.
Optical molecular imaging is in ever-increasing demand for biological research and medical diagnosis as it provides a high detection sensitivity using relatively inexpensive equipment for acquisition and maintenance that can be easily moved to the experiment site. The main requirement to benefit from these advantages is the availability of fluorescent or luminescent imaging agents. Detection in the near-infrared (NIR) range is currently triggering strong enthusiasm for in vitro and in vivo applications as measurements performed in this spectral domain minimize the contribution of the native fluorescence of biological systems (autofluorescence), providing a higher signal-to-noise ratio and correspondingly enhanced detection sensitivity.1 In this respect, the luminescence of lanthanide(III) ions (Ln3+) possesses complementary advantages in respect to organic fluorophores and semi-conductor nanocrystals (quantum dots): sharp emission bands whose wavelengths are not affected by the experimental conditions, large energy differences between excitation and emission bands (pseudo-Stokes shift), and long luminescence lifetimes (μs–ms range).2 However, the forbidden nature of most of the f–f transitions results in the extremely low absorption of free Ln3+, strongly limiting the number of photons emitted. In order to overcome this limitation, Ln3+ need to be located sufficiently close to a chromophoric ligand able to absorb a high number of photons and to transfer the resulting energy to the Ln3+. This sensitization mechanism is called “the antenna effect”.3 In our quest for the creation of NIR-emitting imaging agents based on Ln(III) compounds, we have tested several approaches and systems that have shown their ability to be used under biological conditions as cell imaging probes.4 However, some of these systems have relatively large sizes (i.e. metal–organic frameworks4a and dendrimers4b) with the potential risk of interfering with the biological systems to be studied. Moreover, none of the NIR-emitting Ln(III)-based imaging probes that we have created so far possess the capability of being functionalized with a biological recognition entity in order to target a specific location.4c,d The goal of the present project was therefore to create prototypes of Ln(III)-based NIR-emitting agents based on small molecules using a methodological design approach that allows the attachment of a targeting peptide to a chromophoric unit. In addition, these prototypes have also to include Ln(III) complexes: for Ln(III) complexes to be used as imaging agents, two essential parameters are a high thermodynamic stability and/or kinetic inertness as these properties reflect the absence of dissociation and therefore prevent the loss of the luminescence signal and the release of free Ln3+ that are toxic during experiments. A derivative of the 1,4,7,10-tetraazacyclodecane-1,4,7-triacetic acid (DO3A) macrocycle was chosen to coordinate Ln3+ due to the almost complete absence of dissociation of the corresponding complexes in aqueous media (log
KLnL up to 29).5 Among functional fluorophores, azo-dyes are a well-established class of organic photoactive molecules that possess excellent chemical stability, and have been widely used as photoswitches to follow changes in different biological systems.6 They can tolerate a broad range of organic reactions, an ideal feature to successively install the Ln3+ complex and the targeting moiety. Only few reports described the use of azo-dyes as sensitizers of NIR-emitting Ln3+ ions.6d,e Furthermore, molecules based on azo-dyes can be chemoselectively conjugated to any biomolecule able to target a specific receptor for biological imaging applications. Here, we used this specificity through the copper-catalyzed alkyne/azide cycloaddition7 (CuAAC) conjugation with a cyclopeptide containing an Arg-Gly-Asp (RGD) moiety. This tripeptide motif is a ligand of the αvβ3 integrin overexpression which is associated with the progression of various processes such as osteoclast mediated bone resorption, angiogenesis and pathological neovascularisation, as well as tumour metastasis.8 Among many RGD-containing molecules, the cyclo(Arg-Gly-Asp-D-Phe-Val) peptide (cRGD) initially developed by Kessler and co-workers for cancer therapy9 is a highly potent and selective αvβ3 antagonist. This breakthrough discovery paved the way for the development of cRGD derivatives conjugated with cytotoxic compounds or probes for applications in targeted drug delivery or cancer imaging, respectively.10 This cyclopentapeptide targeting unit is now widely established, and we used it as a benchmark to demonstrate the validity and versatility of our synthetic strategy.
Cyclen-based ligands incorporating azo-dyes for the binding and sensitization of NIR-emitting Ln3+ ions were prepared following the procedure presented on Scheme 1. To pursue our objective of developing new nitrogen containing compounds,11 azobenzene compounds 3a–d were synthesized using standard conditions from aniline derivatives 1a–d. The versatility of this synthetic strategy was validated since the reaction was successfully performed using aniline derivatives with different para-substituents including the electron-donating methoxy group that provides solubility or a halogen atom that allows further cross-coupling reactions to be performed.12 With the aim of introducing a biomolecule targeting unit by the CuAAC reaction, derivative 3d was designed with an appended alkyne group. The tri-t-butyl 1,4,7,10-tetraazacyclododecane-1,4,7-triacetate (DO3A-tBu-ester) chelating unit was then anchored to the bromoacetamides 3a–d via a stable amide bond leading to the formation of DO3A ligands 4a–d with high yields. The subsequent deprotection of the t-butyl ester groups of 4a–d using a solution of triisopropylsilane in trifluoroacetic acid (2% v/v) gave the tricarboxylic acids 5a–d in good yields. The reaction of 5a–d with lanthanide triflates in methanol at 50 °C resulted in the formation of the corresponding complexes (5-Ln; Ln = Gd, Nd, Yb). Further, the ability of the water–soluble complexes 5d-Ln to act as substrates in CuAAC reactions was investigated with the azido-containing cRGD derivative 6.13–15 Coupling yielded the new and original conjugates 7-Ln (Ln = Gd, Nd, Yb). It should be mentioned that metalation was performed before the cycloaddition step to avoid a premature and irreversible sequestering of Cu2+ ions by the carboxylic functionality of the DO3A moiety of 5d.16 This feature is particularly attractive as different bioconjugates could thus be potentially envisaged through the CuAAC reaction. Semi-preparative RP-HPLC was then used to purify the 7-Ln complexes. High resolution MS characterization of complexes 7-Nd, 7-Yb, 7-Gd confirmed the formation of the products (see ESI†).
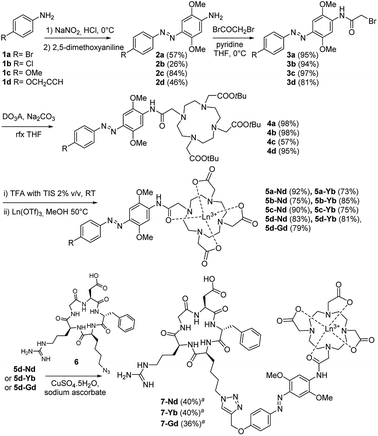 |
| Scheme 1 Synthesis of the 5-Ln and CuAAC reaction between 5d-Ln and azido-containing cRGD derivative 6 (Ln = Nd, Yb, Gd). Percent yields of different reactions are given in parentheses. aIsolated yields after purification by HPLC. | |
An extensive photophysical characterization was carried out on aqueous solutions of the 7-Ln conjugates with Yb3+, Nd3+ and Gd3+ ions. The absorption spectra are depicted in Fig. 1. They reveal that the 7-Ln complexes possess broad absorption bands, almost independent of the nature of the Ln3+ ion, in the UV and visible range up to 550 nm due to π ← π* and n ← π* transitions with features at 250 (ε250 = 7760 M−1 cm−1), 280 (ε280 = 4200–4600 M−1 cm−1), 347 (ε347 = 10
250–10
400 M−1 cm−1), 405 (ε405 = 12
000–12
250 M−1 cm−1) and 457 (8900–9300 M−1 cm−1) nm. Azobenzenes are known to undergo cis–trans photoisomerization upon illumination.6b,c,17 Our experiments performed on the solution of the 7-Nd complex demonstrated no significant changes in the shape and intensities of the absorption bands after 2 h of continuous illumination at 350 nm (Fig. S1, ESI†), thus reflecting an absence of photobleaching and photoactivated processes for the studied complexes. The analysis of the ligand-centred emission of the 7-Gd complex is useful to gather information about the energy of the triplet state which is hypothesized to play a major role in the energy transfer between the antennae and the Ln3+ ion.3 Under excitation in the range 340–450 nm no emission was observed for the 7-Gd complex at room temperature. When the temperature was lowered to 77 K a broad-band emission arising most probably from n → π* transitions and centred at 590 nm was clearly detected under excitation either at 340 or 450 nm (Fig. 2, red and blue traces). Switching from a continuous to a flash source of excitation (λex = 340 nm) and applying a delay of 500 μs led to the generation of the structured broad-band emission centred at 520 nm, that can be assigned to 3π → π* transitions (Fig. 3, black trace). It should be noted that no emission could be detected upon flash excitation at 450 nm. The energy of the 3ππ* triplet state, estimated as 0–0 transition, is thus equal to 20
620 cm−1 (485 nm). The position of the 3ππ* triplet state is higher than the accepting levels of Nd3+ (4F3/2) and Yb3+ (2F5/2) so can potentially act as a feeding level.
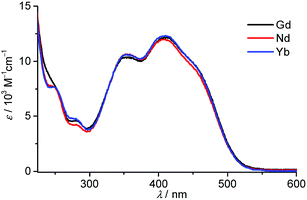 |
| Fig. 1 Absorption spectra of 7-Ln complexes (Ln = Nd, Gd, Yb) at room temperature (H2O, 200 μg mL−1). | |
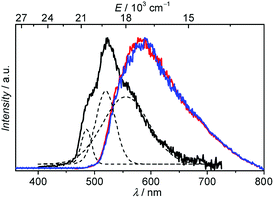 |
| Fig. 2 Emission spectra of the 7-Gd complex recorded at room temperature under continuous excitation at 340 nm (blue trace) or 450 nm (red trace), and at 77 K under excitation at 340 nm upon applying a 500 μs delay after the flash (black trace) (H2O/glycerol = 9/1, 200 μg mL−1). The dashed lines represent the Gaussian deconvolution of the phosphorescence spectrum. | |
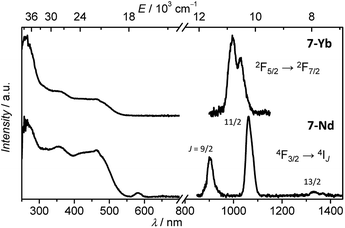 |
| Fig. 3 (Left) Excitation upon monitoring either the Yb3+ emission signal at 980 nm or Nd3+ at 1064 nm, and (right) emission spectra upon excitation at 450 nm of 7-Nd and 7-Yb complexes at room temperature (H2O, 1 mg mL−1). | |
Under excitation in the range of the antenna absorption, sharp emission bands with maxima at 903, 1062 and 1332 nm that are typical of Nd3+-centred electronic transitions between 4F3/2 and 4IJ (J = 9/2, 11/2, 13/2) levels as well as a Yb3+-centred band with the maximum at 996 nm arising from the 2F5/2 → 2F7/2 transition were observed for the 7-Nd and 7-Yb complexes, respectively (Fig. 3). Upon monitoring the emission bands of either Nd3+ (at 1064 nm) or Yb3+ (at 980 nm), the excitation spectra of the 7-Nd and 7-Yb complexes exhibited broad bands in the range 250–550 nm which are fairly similar to the absorption spectra of the corresponding complexes. These observations indicate that the sensitization of the NIR-emitting Ln3+ ion occurs through the electronic levels of the chromophoric ligand, validating an antenna effect. We can notice the presence of an additional band with a maximum at 582 nm on the excitation spectrum of 7-Nd. This band can be assigned to 4G5/2,2G7/2 ← 4I9/2 transitions of Nd3+ and reflects the possibility of direct excitation of the characteristic emission of this cation through f–f transitions. Luminescence lifetimes (τobs) of Yb3+- and Nd3+-centred transitions were determined in order to monitor changes in the environment around the Ln3+ ion (Table 1). Luminescence decays were collected in H2O and D2O solutions as the treatment of these data using empirical equations18 allows the determination of the number of water molecules coordinated onto Ln3+ (q values, Table 1). In order to establish whether the presence of the cRGD peptide significantly affected the environment and the protection of the Ln3+ ions, τobs were estimated not only for the 7-Nd, 7-Yb but also for the 5d-Nd, 5d-Yb (Table S1, ESI†) complexes. Analysis of the experimental luminescence decays obtained upon excitation in the electronic levels of the organic ligand at 355 nm and monitoring emissions at 1064 or 980 nm revealed that they are best fitted with single-exponential functions. The calculated q values for all four complexes are consistent with the presence of one molecule of water coordinated onto Ln3+. In addition, the similarity of the τobs values for Yb3+ and Nd3+ complexes formed with the ligands 5d and 7 demonstrated that the presence of the cRGD peptide had no or a very limited impact on the local environment of Ln3+ ions.
Table 1 Observed luminescence lifetimes (τobs) and absolute quantum yields (QLLn) of 7-Nd and 7-Yb at room temperature (H2O or D2O, 1 mg mL−1)a
Complex |
τobsb [μs] |
qc |
QLLnd [%] |
H2O |
D2O |
2σ values in parentheses. Experimental errors: τ, ±2%, QLLn, ±10%. Under excitation at 355 nm upon monitoring the emissions of Nd3+ at 1064 nm and Yb3+ at 980 nm. Calculated according to phenomenological equations reported in ref. 17. Under excitation at 450 nm. |
7-Nd |
0.069(1) |
0.238(0) |
1.0 |
2.4(1) × 10−4 |
7-Yb |
0.793(1) |
5.843(3) |
0.9 |
8.4(1) × 10−5 |
The Ln3+-centred luminescence quantum yield under ligand excitation (QLLn) is a quantitative parameter that reflects the efficiency of the antenna to sensitize the NIR-emitting Ln3+ combined with the degree of protection of this cation against sources of non-radiative deactivations. The values of QLYb and QLNd can be considered as modest compared with corresponding Yb3+ and Nd3+ DO3A complexes, with one molecule of water coordinated to the Ln3+ ion, while observed lifetime values are similar.19 Such results indicate a moderate ability of the studied ligand incorporating an azobenzene chromophore to sensitize Yb3+ and Nd3+.
In summary, we have described the construction of an original system that combines azobenzene-based chromophores with the cyclen coordinating unit and the targeting cRGD peptide moiety in five easy-to-perform and versatile steps. This strategy could find widespread application by anchoring Ln(III)-based luminescent probes to diverse biomolecules or targeting units.20 Photophysical studies demonstrated that this system allows the sensitization of both NIR-emitting Nd3+ and Yb3+ ions in aqueous solutions although with moderate efficiency. The analysis of the luminescence lifetimes confirmed the presence of a water molecule coordinated onto Ln3+ which causes non-radiative deactivation and partially explains the relatively modest values of NIR quantum yields. Nevertheless, the versatility of the described approach that allows: (i) a variation of substituents on the chromophoric unit in order to enhance the sensitization efficiency of NIR-emitting Ln3+ ion or to replace a water molecule from the Ln3+ coordination sphere, (ii) an exchange of the cyclen coordination unit to the one with higher denticity, would address current limitations and open promising possibilities for practical applications of these molecules.
Conflicts of interest
There are no conflicts to declare.
Acknowledgements
M. C. B. thanks the Association pour la Recherche sur le Cancer (ARC), the Region Centre-Val de Loire (APR-IR 2011 Multiplex) and the LABEX SynOrg (ANR-11-LABX-0029) for financial support. S. V. E. was supported through a grant from the European Community's Seventh Framework Programme (FP7 IEF Dendrimage – project number 316906). S. P. acknowledges support from the Institut National de la Santé et de la Recherche Médicale (INSERM). Dr M. Galibert is greatly acknowledged for his help with the peptide conjugation reactions. We acknowledge Dr G. Gabant and the mass spectrometry platform of CBM for the MS analyses of peptides and peptide conjugates.
Notes and references
-
(a) R. Weissleder and V. Ntziachristos, Nat. Med., 2003, 9, 123 CrossRef CAS PubMed;
(b) J. V. Frangioni, Curr. Opin. Chem. Biol., 2003, 7, 626 CrossRef CAS PubMed;
(c) I. Martinić, S. V. Eliseeva and S. Petoud, J. Lumin., 2017, 189, 19 CrossRef.
-
(a) S. V. Eliseeva and J.-C. G. Bünzli, Chem. Soc. Rev., 2010, 39, 189 RSC;
(b) J.-C. G. Bünzli, Chem. Rev., 2010, 110, 2729 CrossRef PubMed.
-
(a) H. Uh and S. Petoud, C. R. Chim., 2010, 13, 668 CrossRef CAS;
(b) J.-C. G. Bünzli and S. V. Eliseeva, Photophysics of Lanthanoide Coordination Compounds, in Comprehensive Inorganic Chemistry II, ed. J. Reedijk, K. Poeppelmeier Elsevier B.V., Amsterdam, 2013, vol. 8, ch. 8.08, pp. 339–398 Search PubMed.
-
(a) A. Foucault-Collet, K. A. Gogick, K. A. White, S. Villette, A. Pallier, G. Collet, C. Kieda, T. Li, S. J. Geib, N. L. Rosi and S. Petoud, Proc. Natl. Acad. Sci. U. S. A., 2013, 110, 17199 CrossRef CAS PubMed;
(b) A. Foucault-Collet, C. M. Shade, I. Nazarenko, S. Petoud and S. V. Eliseeva, Angew. Chem., Int. Ed., 2014, 53, 2927 CrossRef CAS PubMed;
(c) I. Martinić, S. V. Eliseeva, T. N. Nguyen, V. L. Pecoraro and S. Petoud, J. Am. Chem. Soc., 2017, 139, 8388 CrossRef PubMed;
(d) I. Martinić, S. V. Eliseeva, T. N. Nguyen, V. L. Pecoraro and S. Petoud, Chem. Sci., 2017, 8, 6042 RSC.
- S. L. Wu and W. D. Horrocks, J. Chem. Soc., Dalton Trans., 1997, 1497 RSC.
-
(a) F. Hamon, F. Djedaini-Pilard, F. Barbot and C. Len, Tetrahedron, 2009, 65, 10105 CrossRef CAS;
(b) J. Griffiths, Chem. Soc. Rev., 1972, 1, 481 RSC;
(c) A. A. Beharry and G. A. Woolley, Chem. Soc. Rev., 2011, 40, 4422 RSC;
(d) M. P. Placidi, A. J. L. Villaraza, L. S. Natrajan, D. Sykes, A. M. Kenwright and S. Faulkner, J. Am. Chem. Soc., 2009, 131, 9916 CrossRef CAS PubMed;
(e) K. Aita, T. Temma, Y. Kuge, K. Seki and H. Saji, Luminescence, 2010, 25, 19 CrossRef CAS PubMed.
-
(a) C. W. Tornøe, C. Christensen and M. Meldal, J. Org. Chem., 2002, 67, 3057 CrossRef;
(b) V. V. Rostovtsev, L. G. Green, V. V. Fokin and K. B. Sharpless, Angew. Chem., Int. Ed., 2002, 41, 2596 CrossRef CAS;
(c) F. de Moliner, N. Kielland, R. Lavilla and M. Vendrell, Angew. Chem., Int. Ed., 2017, 56, 3758 CrossRef CAS PubMed.
-
(a) R. O. Hynes, Cell, 1992, 69, 11 CrossRef CAS PubMed;
(b) G. J. Mizejewski, Proc. Soc. Exp. Biol. Med., 1999, 222, 124 CrossRef CAS PubMed;
(c) H. Jin and J. Varner, Br. J. Cancer, 2004, 90, 561 CrossRef CAS PubMed.
-
(a) M. Gurrath, G. Müller, H. Kessler, M. Aumailley and R. Timpl, Eur. J. Biochem., 1992, 210, 911 CrossRef CAS PubMed;
(b) D. Arosio and C. Casagrande, Adv. Drug Delivery Rev., 2016, 97, 111 CrossRef CAS PubMed.
-
(a) F. Danhier, A. Le Breton and V. Préat, Mol. Pharm., 2012, 9, 2961 CrossRef CAS PubMed;
(b) K. Temming, R. M. Schiffelers, G. Molema and R. J. Kok, Drug Resist. Updates, 2005, 8, 381 CrossRef CAS PubMed.
-
(a) J. Hedouin, C. Schneider, I. Gillaizeau and C. Hoarau, Org. Lett., 2018, 20, 6027 CrossRef CAS PubMed;
(b) N. Gigant, L. Chausset-Boissarie and I. Gillaizeau, Chem. –Eur. J., 2014, 20, 7548 CrossRef CAS PubMed.
- K.-P. Tseng, Y.-T. Tsai, C.-C. Wu, J.-J. Shyue, D. M. Bassani and K.-T. Wong, Chem. Commun., 2013, 49, 11536 RSC.
- 6 is a well-established cRGD derivative for conjugation with imaging probes, see for example:
(a) I. Dijkgraaf, A. Y. Rijnders, A. Soede, A. C. Dechesne, G. Wilma van Esse, A. J. Brouwer, F. H. M. Corstens, O. C. Boerman, D. T. S. Rijkersa Rob and M. J. Liskamp, Org. Biomol. Chem., 2007, 5, 935 RSC;
(b) S. S. van Berkel, A. J. Dirks, S. A. Meeuwissen, D. L. L. Pingen, O. C. Boerman, P. Laverman, F. L. van Delft, J. J. L. M. Cornelissen and F. P. J. T. Rutjes, ChemBioChem, 2008, 9, 1805 CrossRef CAS PubMed;
(c) J. Paleček, G. Dräger and A. Kirschning, Synthesis, 2011, 653 Search PubMed;
(d) P. Verwilst, S. V. Eliseeva, L. Vander Elst, C. Burtea, S. Laurent, S. Petoud, R. N. Muller, T. N. Parac-Vogt and W. M. De Borggraeve, Inorg. Chem., 2012, 51, 6405 CrossRef CAS PubMed;
(e) H. Li, H. Zhou, S. Krieger, J. J. Parry, J. J. Whittenberg, A. V. Desai, B. E. Rogers, P. J. A. Kenis and D. E. Reichert, Bioconjugate Chem., 2014, 25, 761 CrossRef CAS PubMed;
(f) P. Verwilst, S. V. Eliseeva, S. Carron, L. Vander Elst, C. Burtea, G. Dehaen, S. Laurent, K. Binnemans, R. N. Muller, T. N. Parac-Vogt and W. M. De Borggraeve, Eur. J. Inorg. Chem., 2011, 2011, 3577 CrossRef CAS.
- For other azide-containing cRGD derivatives, see for example:
(a) C. Wängler, S. Maschauer, O. Prante, M. Schäfer, R. Schirrmacher, P. Bartenstein, M. Eisenhut and B. Wängler, ChemBioChem, 2010, 11, 2168 CrossRef PubMed;
(b) M. Galibert, O. Renaudet, P. Dumy and D. Boturyn, Angew. Chem., Int. Ed., 2011, 50, 1901 CrossRef CAS PubMed.
- A novel synthetic approach towards azidocylopeptide 6 was optimized and is based on a convenient solid-phase cyclization adapted to further scale up, see the ESI† for details.
-
(a) S. V. Smith, J. Inorg. Biochem., 2004, 98, 1874 CrossRef CAS PubMed;
(b) Y. Song, E. K. Kohlmeir and T. J. Meade, J. Am. Chem. Soc., 2008, 130, 6662 CrossRef CAS PubMed.
- A stable trans (E) configuration for azobenzene-based compounds 2a–d is assumed. See:
(a) K.-P. Tseng, Y.-T. Tsai, C.-C. Wu, J.-J. Shyue, D. M. Bassani and K.-T. Wong, Chem. Commun., 2013, 49, 11536 RSC;
(b) J. Zeitouny, C. Aurisicchio, D. Bonifazi, R. De Zorzi, S. Geremia, M. Bonini, C.-A. Palma, P. Samorì, A. Listorti, A. Belbakrab and N. Armaroli, J. Mater. Chem., 2009, 19, 4715 RSC;
(c) E. Vaselli, C. Fedele, S. Cavalli and P. A. Netti, ChemPlusChem, 2015, 80, 1547 CrossRef CAS.
-
(a) A. Beeby, S. Faulkner, D. Parker and J. A. G. Williams, J. Chem. Soc., Perkin Trans. 1, 2001, 2, 1268 RSC;
(b) S. Faulkner, A. Beeby, M.-C. Carrié, A. Dadabhoy, A. M. Kenwright and P. G. Sammes, Inorg. Chem. Commun., 2001, 4, 187 CrossRef CAS.
-
(a) Z. Hashami, A. F. Martins, A. M. Funk, V. C. Jordan, S. Petoud, S. V. Eliseeva and Z. Kovacs, Eur. J. Inorg. Chem., 2017, 43, 4965 CrossRef PubMed;
(b) M. Isaac, L. Raibault, C. Cepeda, A. Roux, D. Boturyn, S. V. Eliseeva, S. Petou and O. Sénèque, Chem. –Eur. J., 2017, 23, 10992 CrossRef CAS PubMed.
-
(a) A. J. Amoroso and S. J. A. Pope, Chem. Soc. Rev., 2015, 44, 4723 RSC;
(b) R. D. Teo, J. Termini and H. B. Gray, J. Med. Chem., 2016, 59, 6012 CrossRef CAS PubMed.
Footnote |
† Electronic supplementary information (ESI) available: Experimental procedures, characterization data, NMR spectra and details of photophysical measurements. See DOI: 10.1039/c8ra09419e |
|
This journal is © The Royal Society of Chemistry 2019 |