DOI:
10.1039/C8RA09081E
(Paper)
RSC Adv., 2019,
9, 1115-1122
Enhanced electrochemical performance of nanoplate nickel cobaltite (NiCo2O4) supercapacitor applications†
Received
2nd November 2018
, Accepted 26th December 2018
First published on 9th January 2019
Abstract
Well-ordered, unique interconnected nanostructured binary metal oxides with lightweight, free-standing, and highly flexible nickel foam substrate electrodes have attracted tremendous research attention for high performance supercapacitor applications owing to the combination of the improved electrical conductivity and highly efficient electron and ion transport channels. In this study, a unique interconnected nanoplate-like nickel cobaltite (NiCo2O4) nanostructure was synthesized on highly conductive nickel foam and its use as a binder-free material in energy storage applications was assessed. The nanoplate-like NiCo2O4 nanostructure electrode was prepared by a simple chemical bath deposition method under optimized conditions. The NiCo2O4 electrode delivered an outstanding specific capacitance of 2791 F g−1 at a current density of 5 A g−1 in a KOH electrolyte in a three-electrode system as well as outstanding cycling stability with 99.1% retention after 3000 cycles at a current density of 7 A g−1. The as-synthesized NiCo2O4 electrode had a maximum energy density of 63.8 W h kg−1 and exhibited an outstanding high power density of approximately 654 W h kg−1. This paper reports a simple and cost-effective process for the synthesis of flexible high performance devices that may inspire new ideas for energy storage applications.
Introduction
The development of renewable energy conversion systems is an urgent requirement to meet the needs of high power electronic devices, electric vehicles, and electrochemical energy storage devices as well as address ecological concerns.1–3 Among the various electrochemical energy storage systems, supercapacitors have attracted increasing attention in recent years because they exhibit a good balance between energy density and power density, excellent long-life cycling stability with a rapid charge/discharge rate over batteries, and store more energy than conventional capacitors.4–9
Electrode materials can be classified into two main groups based on the charge storage mechanism: electric double-layer capacitors (EDLCs) and pseudocapacitors (PCs).10,11 Generally, EDLCs consist of conductive porous carbon materials, such as carbon spheres, activated carbon, and graphene.12–16 On the other hand, the practical applications of EDLCs have been limited by their low specific capacitance and low energy density. The main pseudocapacitive materials, such as metal oxides, hydroxides and conducting polymers, exhibit higher specific capacitance and energy densities through rapid, reversible, multi-electron, surface faradaic reactions.17–21 In particular, some low-cost transition metal oxides and hydroxides, such as ZnO, Fe2O3, MnO2, Mn3O4 Co3O4, NiO, and Co(OH)2, have been developed as candidates.22–26
Among the various electroactive materials reported thus far, nickel oxide (NiO) is a typical PC material owing to its strong theoretical capacity, excellent reversibility, well-maintained and fascinating morphology, suitable pore size, large specific area, and excellent reliability.27–29 However, NiO as an active material in PCs has been restricted because of its lower rate behavior, poor cycling stability, and lower electrochemical activity.30–32 To overcome this problem, further efforts have been made to prepare nanocomposites that combine NiO with other electroactive materials. Among the various PCs components, cobalt oxides (Co3O4 and CoO) have become promising candidates for supercapacitor electrode applications owing to their low toxicity, low parity cost, facile preparation, and good corrosion stability.33,34 Moreover, binary metal oxides exhibit better electrochemical performance compared to single-component metal oxides owing to their outstanding electrical conductivity and multiple oxide states.
In particular, ternary nickel cobaltite (NiCo2O4) has attracted considerable attention for its ultrahigh specific capacitance because ternary NiCo2O4 combines the characteristics of simple transition metal oxides, has high specific capacitance and electronic conductivity, and better electron transport between the electrolyte and electrode surface area than binary metal oxides of nickel oxide (NiO) and cobalt oxide (Co3O4).35,36 In particular, NiCo2O4 has attracted considerable attention, owing to its superior conductivity to other binary metal oxides, such as ZnFe2O4, CoMoO4, MnMoO4, Zn3V2O8, and NiMnO3. Nickel cobaltite (NiCo2O4) has excellent redox activity, natural abundance, low cost, environmentally benign characteristics, and nontoxicity.37,38 Thus, the fabrication of nanoplate-structured NiCo2O4 using a simple and novel method to enhance its electrochemical performance is the focus of this research. Until now, there are only a few reports on the use of NiCo2O4 as an electrode material for supercapacitor applications. The chemical bath deposition approach is a more common method for the preparation of functional materials than hydrothermal methods, microwave irradiation method, thermal annealing treatment approach, and mechano-chemical synthesis. Despite this, little attention has been paid to the synthesis of NiCo2O4 electrode materials. The production of high-performance supercapacitors for nanostructured NiCo2O4 materials by a simple method remains a challenge.
In this study, a nanostructure of NiCo2O4 nanoplate-like structure was grown uniformly on highly conductive nickel foam for high-performance supercapacitor applications through a chemical bath deposition method. By rationally controlling the growth kinetics of NiCo2O4, the electrode exhibited a honeycomb nanostructure on a nickel foam surface, which provided rapid channels for ion and electron transfer and formed abundant electrochemically active sites exposed to the electrolyte surface area. The NiCo2O4 nanoplate's electrode exhibited a high specific capacitance of 2791 F g−1 at a current density of 5 A g−1 and delivered an excellent energy density 63.8 W h kg−1 and high power density of 654 W kg−1 in a KOH electrolyte. This might be because the unique interconnected nanoplate's structure exhibits good reversibility and lower charge-transfer resistance of 0.2 Ω during the faradaic process. These supercapacitors also exhibited excellent long-life cycling stability of approximately 99.1% retention after 3000 cycles, which might be because the nanoplate nanostructure allows convenient ion transport within and between the combs.
Experimental section
Preparation of the NiCo2O4 nanoplate material on nickel foam
All chemicals applied, such as nickel nitrate hexahydrate, cobalt nitrate hexahydrate, urea, and ammonium fluoride, were of analytical grade and used as received. The nanoplate-like NiCo2O4 nanostructure was synthesized by a simple chemical bath deposition method. The process involved a solvothermal reaction of nickel and cobalt ions as inorganic components with urea and ammonium fluoride to form a precursor/self-sacrificing template, which was followed by heat treatment in air to generate nanoplate-like structures. Prior to synthesis, a piece of nickel (1 cm × 1 cm) foam was cleaned by being immersed in 2 M HCl under ultrasonication in sequence for 15 min, and rinsed with absolute ethanol and deionized (DI) water, respectively, which results in the removal of the surface layer and greasing of Ni oxide. In particular, an aqueous homogeneous solution of all chemicals applied, such as 0.42 g of Ni (NO3)2·6H2O and 0.86 g of Co(NO2)3·6H2O, 0.22 g of CH4N2O and 1.80 g of urea were dissolved in 100 mL of deionized water with constant magnetic stirring for 50 min. After stirring, the homogeneous solution formed a clear pink color solution, which indicates the active electrode of nickel cobaltite (NiCo2O4) material. The resulting clear solution containing a piece of nickel foam was transferred into a 100 mL capped bottle and kept at 120 °C for 12 h. After cooling to room temperature, the as-prepared nickel foam was separated by centrifugation and rinsed with absolute ethanol and distilled water. The as-prepared product was then dried with a dryer for 30 min. Finally, for the heat treatment step, the resulting prepared product was annealed under 400 °C for 3 h at a heating rate of 20 °C min−1 in air. The as-prepared samples supported on nickel foam were obtained. The total average NiCo2O4 loading was 3 mg cm−2.
Material characterization
The morphology, microstructure, and internal structural properties of the samples were studied by field emission scanning electron microscopy (FESEM, S-4800, Hitachi) at an acceleration voltage of 15 kV and field emission transmission electron microscopy (FETEM, JEOL, JEM-2100F) operated at 200 kV. The crystalline phase structure of the as-prepared sample was investigated by powder X-ray diffraction (XRD, D8 ADVANCE Bruker) at a voltage of 40 kV and a current of 40 mA using Cu Kα radiation (k = 0.1542 nm). The elemental composition of the material was determined by X-ray photoelectron spectroscopy (XPS, VG Scientific ESCALAB 250) using Al Kα radiation (Scheme 1).
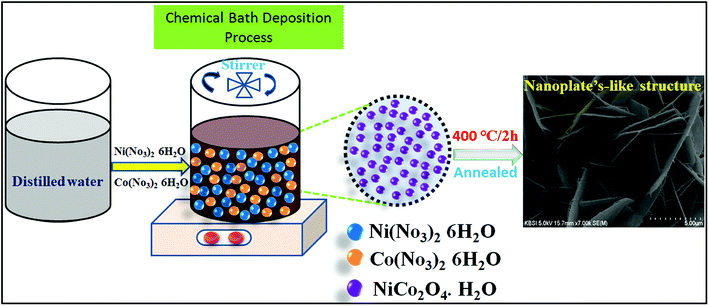 |
| Scheme 1 Schematic of the preparation process of NiCo2O4 nanoplate's structure. | |
Electrochemical measurements
Electrochemical characterization of the NiCo2O4 were carried out in a 3 M aqueous KOH solution on an electrochemical workstation (BioLogic-SP150 Korea) using a conventional three electrode system. A three-electrode system containing Ag/AgCl and platinum foil (Pt) as the reference and counter electrode, respectively, was used. The as-prepared NiCo2O4 electrode were directly used as the working electrode. Cyclic voltammetry (CV) of the resulting product was performed over the potential range from −0.2 to 0.5 V vs. Ag/AgCl at different scan rates. The galvanostatic charge–discharge curves test of the prepared electrode was performed over the potential range, 0 to 0.4 V vs. Ag/AgCl, at various current densities (A). Electrochemical impedance spectroscopy (EIS) was conducted over the frequency range, 0.1 Hz to 100 kHz with an AC amplitude of 5 mV. The specific capacitance (Csc, F g−1) of the electrode from GCD plots, energy density (E, W h kg−1) and power density (P, W kg−1) for the three electrode system were calculated using the following equations (eqn (1), (2), and (3)) given below:39 |
 | (1) |
|
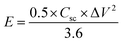 | (2) |
|
 | (3) |
where I (A) is the discharge current, Δt (s) is the discharge time, m (g) is the mass of the electroactive material of the active electrode, and ΔV (V) is the voltage window drop, respectively.
Results and discussion
The morphology, interior structure, and crystalline properties of the as-prepared NiCo2O4 nanomaterial was examined by SEM, TEM and high-resolution TEM (HR-TEM), as shown in Fig. 1. Fig. 1a–c shows the morphology of the nanoplate-like NiCo2O4 at various magnifications. The nickel foam is covered uniformly by NiCo2O4 nanoplate structures, indicating that the nanoplates are composed of many nanosheets. The fine nanoplates had a mean diameter of 50–80 nm. As shown in Fig. 1c, the internal structure of the as-prepared material has larger pores because of the empty spaces between the plates. In particular, this nanostructure has high specific surface areas with a bimodal pore size distributions, which play a key role in the high energy density and power density suitable for energy storage applications. Moreover, FE-SEM images of the working electrode showed that every nanoplate had grown uniformly on the nickel foam and had a rough surface area. The SEM image (Fig. S1a†) of the NiCo2O4 at low magnification shows that a layer of precursor is evenly covered on the surface of Ni foam. After the nanoplate's growth, the NiCo2O4 nanoplates covered the surface of nickel foam uniformly and also nanoplates grown on nickel foam were uniformly distributed with no overlap. The magnified image in the inset reveals shows a low magnification SEM image of the visible networks of Ni foam, in which we can see that the Ni foam has a porous network structure with smooth surface and also no impurities. Fig. 1d and e presents TEM and HR-TEM images taken on the surface of the NiCo2O4 nanoplates coated on the surface of nickel foam. As shown in Fig. 1d, the internal structure of the active material clearly showed large pores and nanoplate regions. Atomic force microscopy (AFM) is a promising technique for studying some structural information and thickness measurements about the as-obtained material. Fig. S2a and b† shows topology of the surface of NiCo2O4 on Ni foil. It can be seen that a plate structure of NiCo2O4 is formed on Ni foil. The AFM images reveal a uniform surface roughness, a smooth surface, and good adhesion to the substrate with a narrow particle size distribution. Also, it can be observed that the surface is converted to a relatively uniform size. Furthermore, AFM measurement indicated that the average thickness of these NiCo2O4 nanoplate was around ∼20 to 30 nm. Overall, AFM indicates that the NiCo2O4 contains more electrocatalytic activity sites for the reaction of the redox couple in the KOH electrolyte and has a larger surface area between the electrode and electrolyte. The lattice fringes in the HR-TEM image separated by d = 0.243 nm were assigned to the (311) plane of NiCo2O4 (JCPDS) data, as shown in Fig. 1e.
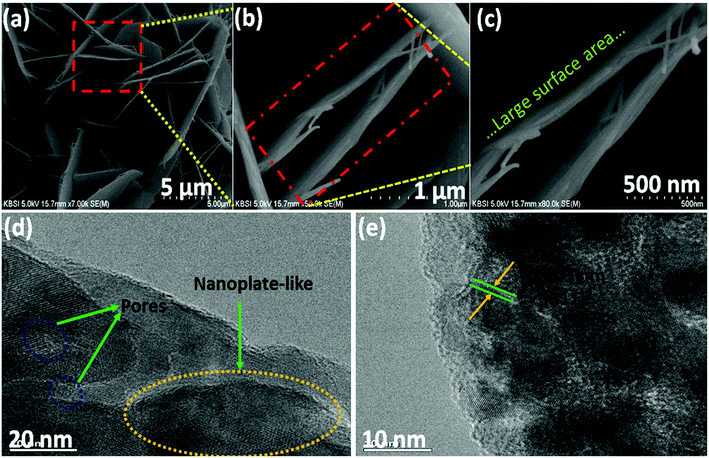 |
| Fig. 1 (a–c) Low- and high-magnification FE-SEM images and (d and e) TEM and HR-TEM images of the NiCo2O4 nanoplate material. | |
The crystallinity and phase composition of the as-prepared NiCo2O4 nanoplate on nickel foam were examined by XRD, as shown in Fig. 2a. XRD showed that the NiCo2O4 material was crystalline. The XRD peaks at 18.91°, 31.15°, 36.70°, 38.40°, 44.62°, 55.44°, 59.09°, 64.98°, and 68.31° 2θ were indexed to the (111), (220), (311), (222), (400), (422), (511), (440), and (531) planes, respectively, of the cubic spinal-like NiCo2O4 Joint Committee in Powder Diffraction Standards (JCPDS card no. 20-0781),40 which is consistent the TEM analysis. XPS provided further evidence of the chemical composition and elemental oxidation states of the as-prepared NiCo2O4 sample. The XPS survey spectrum revealed nickel, cobalt, and oxygen in accordance with the literature values for NiCo2O4 (Fig. 2b). EDX analysis of the as-prepared product showed nickel, cobalt and oxygen (Fig. 2b), indicating a pure phase without impurities. The atomic percentage of Ni, Co, and O were 41.87%, 35.39%, and 22.73% (5
:
4
:
3), respectively. A Gaussian fit of Ni 2p deconvoluted spectrum at 855.8, 861.8, 873.5, and 879.9 eV revealed Ni2+ 2p3/2, Ni3+ 2p3/2, Ni2+ 2p1/2 and Ni3+ 2p1/2, respectively (Fig. 2d), indicating the presence of two spin–orbit doublets and two shakeup satellites denoted as (“Sat.”) as well as +2 and +3 oxidation states in the prepared NiCo2O4 material.41 In the case of the Co 2p (Fig. 2e) deconvoluted spectrum, multiple peaks at 780.5, 789.6, 795.1 and 804.2 eV were assigned to Co2+ 2p3/2, Co2+ 2p3/2, Co3+ 2p1/2, and Co2+ 2p1/2, respectively.42 The high resolution O 1s spectrum (Fig. 2f) was deconvoluted into two main peaks, 530.2 and 530.9 eV, which confirmed the formation of metal–oxygen bonding in the presence of O 1s.43 The data clearly show that the presence of valence and mixed valence of metal ions, Ni2+/Ni3+, Co2+/Co3+, and O 1s, on the surface of the as-prepared NiCo2O4 sample, which is expected to provide sufficient active sites for the redox reaction in the electroactive activity.
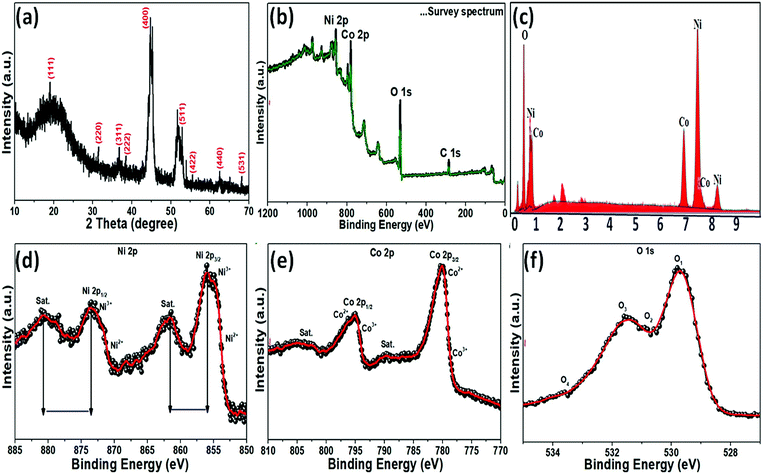 |
| Fig. 2 Spectroscopic and microscopic characterization of the nanoplate-like NiCo2O4 nanostructure. (a) XRD pattern. (b) XPS survey spectrum. (c) EDX spectrum and high-resolution XP spectra of (d) Ni 2p, (e) Co 2p and (f) O 1s for the as-prepared NiCo2O4 sample. | |
The electrochemical performance of as-prepared NiCo2O4 as an electrode material for supercapacitors was investigated using a three-electrode system in a 3 M KOH aqueous solution as the electrolyte. Fig. 3a presents the CV curves of the active electrode. All CV curves showed a pair of redox peaks at various scan rates from 10–100 mV s−1 over the voltage range of −0.2 to 0.5 V, indicating similar pseudocapacitive behavior and good rate capability. In addition, with increasing scan rates, the redox peaks current intensity areas become larger as the scan sweep increased. Furthermore, due to polarization of the electroactive sample, the anodic peaks shifted enormously towards a positive potential, whereas the cathodic peaks moved towards a negative potential with increasing scan rate because of the low resistance and fast ion and electron transfer rates. The strong peaks can be closely related to the chemical composition and morphology of the electrode material for the reversible faradaic redox reactions and redox reaction corresponding to an alkaline electrolyte according to the equations during the electrochemical measurements.
|
NiCo2O4 + H2O + OH− ↔ NiOOH + 2CoOOH + e−
| (4) |
|
2CoOOH + OH− ↔ 2CoO2 + H2O + e−
| (5) |
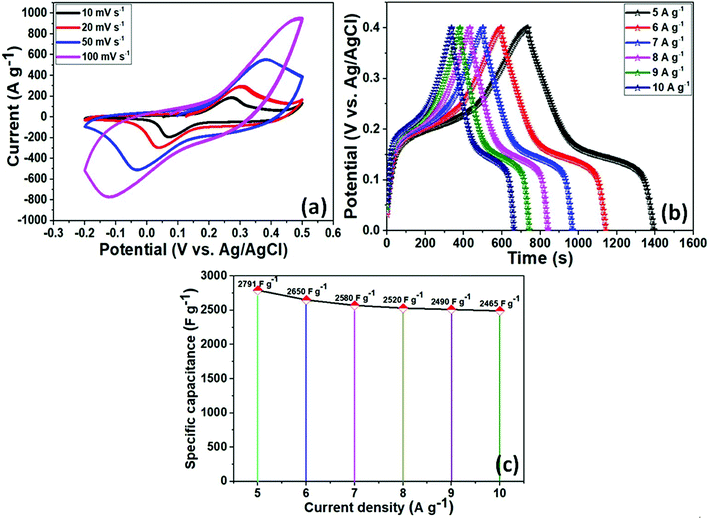 |
| Fig. 3 (a) CV curves of the NiCo2O4 nanoplate's obtained at various scan rates of 10–100 mV s−1; (b) galvanostatic charge–discharge curves of the NiCo2O4 at different current densities of 5–10 A g−1; (c) specific capacitance of the three electrode material at various current densities. | |
In addition, galvanic charge–discharge (GCD) measurements were taken to evaluate the specific capacitance of the electroactive material in a 3 M KOH aqueous electrolyte solution between 0–0.5 V (vs. Ag/AgCl) at various current densities of 5–10 A g−1. The resulting curves were attributed to the faradaic redox process and were close to battery type charge storage, as shown in Fig. 3b. The voltage plateaus in the charge–discharge plots were retained at high scan rates, which indicates the high rate capability of the electrode material. According to eqn (1), the NiCo2O4 electrode exhibited a specific capacitance of 2791, 2650, 2570, 2530, 2510, and 2490 F g−1 at current densities of 5, 6, 7, 8, 8, 9, and 10 A g−1, respectively (as shown in Fig. 3c). The specific capacitance was also superior to those of recently reported flexible electrodes with NiCo2O4 agglomerated particles (351 F g−1 at a current density of 1 A g−1),44 NiCo2O4 nanoflat-like particles (764 F g−1 at the scan rate of 2 mV s−1),45 NiCo2O4 flower-like nanostructure (658 F g−1 at a current density of 1 A g−1),46 NiCo2O4 nanosheets-like (899 F g−1 at the current density of 1 A g−1),47 NiCo2O4 nanoflakes (1270 F g−1 at the current density of 1 A g−1),48 NiCo2O4 nanoneedles nanostructure (1427 F g−1 at the current density of 8 A g−1),49 NiCo2O4 hollow microspheres (764 F g−1 at a current density of 2 A g−1),50 and chain-like NiCo2O4 nanowires (1284 F g−1 at the current density of 2 A g−1).51
Fig. 4a shows the Nyquist plot of the device. The electrochemical characteristics of the electrode were examined further by electrochemical impedance spectroscopy (EIS). The Nyquist plots of the electrode were prepared at the open circuit potential over the frequency range, 0.01 Hz to 100 kHz. In the three electrodes, the Rs value was 0.2 Ω, which indicates the low-frequency range. In addition, the NiCo2O4 electrode showed an almost vertical line, indicating the diffusion of ions at the interface of the electrode/electrolyte, better conductivity and rapid electron transfer kinetics. Fig. 4b presents Ragone plots of the NiCo2O4 electrode of the energy and power densities. The device exhibited an excellent energy density of 63.8 W h kg−1 at a power density of 333 W kg−1, even at a low energy density of 54.4 W h kg−1 at a maximum power density of 654 W kg−1, which is superior to those reported previously, such as NiCo2O4-NC (28 W h kg−1 and 8.5 W kg−1),52 NiCo2O4-GNF//AC (33.8 W h kg−1 and 5 W kg−1),53 NiCo2O4 mesoporous spinal-like (17.72 W h kg−1 and 25.42 W kg−1),54 and NiCo2O4-rGO (23.32 W h kg−1 and 324.9 W kg−1).55
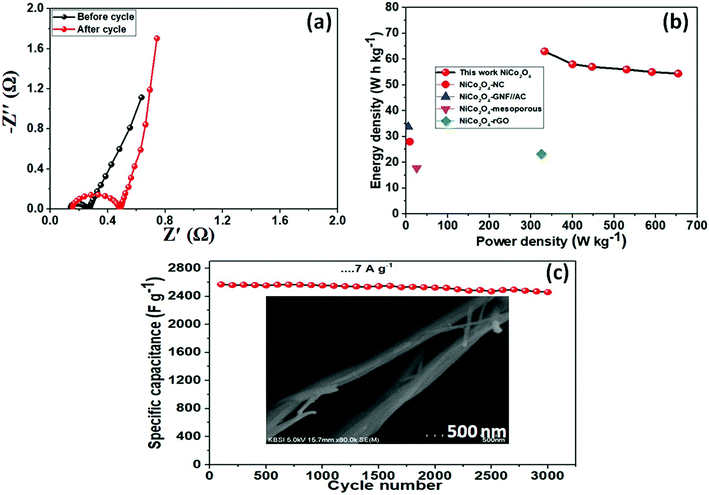 |
| Fig. 4 (a) Nyquist plots of the NiCo2O4 electrode, (b) Ragone plots of the device and (c) cyclic performance of NiCo2O4 nanoplates during 3000 cycles at a scan rate of 7 A g−1. | |
The long-term cycling stability and coulombic efficiency of the as-prepared product as an electrode material was examined by repeating the charge–discharge plots. As shown in Fig. 4c, the NiCo2O4 nanoplate-like structure exhibited excellent long-term electrochemical stability and high rate stability with a very slight decrease to 99.1%, even after 3000 cycles (only 0.9% loss after 3000 cycles) in a three-electrode system at a current density of 7 A g−1. This is superior to those of previously reported 3D hierarchical flower-shaped NiCo2O4 microspheres (93.2% retention after 1000 cycles),56 hierarchical spinal NiCo2O4 nanowires (84.7% retention after 500 cycles),57 NiCo2O4@MnO2 nanowire arrays (88% retention after 2000 cycles),58 NiCo2O4@NiO nanowire arrays (93.1% retention after 3000 cycles),59 NiCo2O4@CoMoO4 nanowire/nanoplates arrays (74.1% retention after 1000 cycles),60 ultrathin porous NiCo2O4 nanosheet arrays (20% reduced after 3000 cycles),61 and NiCo2O4@NiMoO4 nanowires (90.6% retention after 2000 cycles).62 TEM showed that the nanohoneycombs still existed in the NiCo2O4 electrode (inset in Fig. 4c) after long term cycling, suggesting that there was no noticeable change in the morphology of the sample. Such excellent cycling stability of the NiCo2O4 electrode could be attributed to the following: the unique honeycomb structure, which can alleviate the volume changes during the charge–discharge process to guarantee good stability; gradual penetration of the electrolyte ions into the electroactive material; and reduced volume expansion resulting from the rapid and long-term faradaic reaction.
Conclusion
NiCo2O4 with a unique interconnected nanoplate-like structure was fabricated on the surface of the highly conductive nickel foam through a simple chemical bath deposition reaction. The interconnected honeycomb nanostructure electrode can enhance the pathway for electron transport originating from the good electronic conductivity of the nickel foam. In addition, it can reduce the transport distance of ions and enhance electrode–electrolyte contact, which leads to higher material activation, and endow it with a high specific capacitance and excellent long life cycling stability. The NiCo2O4 nanoplate electrode delivered a high specific capacitance of 2791 F g−1 at a current density of 5 A g−1 and exhibited a significantly high energy density of 63.8 W h kg−1 and high power density of 654 W kg−1, highlighting its potential for energy storage applications. The nanoplate structure electrode material for electrochemical capacitors displayed excellent cycling stability of 99.1% retention after 3000 cycles, even at a high current density of 7 A g−1. As a result, the NiCo2O4 electrode provides a path for high-performance supercapacitors because of its low-cost simple chemical bath deposition approach and environmental friendliness.
Conflicts of interest
There are no conflicts to declare.
Acknowledgements
This work was financially supported by BK 21 PLUS, Creative Human Resource Development Program for IT Convergence (NRF-2015R1A4A1041584), Pusan National University, Busan, South Korea. We would like to thank KBSI, Busan for SEM, TEM, XRD, XPS and EDX analysis.
References
- J. L. Liu, J. Wang, C. H. Xu, H. Jiang, C. Z. Li, L. L. Zhang, J. Y. Lin and Z. X. Shen, Adv. Sci., 2017, 1700322 Search PubMed.
- Y. A. Kumar and H.-J. Kim, Energies, 2018, 11, 3285 CrossRef CAS.
- S. G. Deng, D. L. Chao, Y. Zhong, Y. X. Zeng, Z. J. Yao, J. Y. Zhan, Y. D. Wang, X. L. Wang, X. H. Lu, X. H. Xia and J. P. Tu, Energy Storage Materials, 2018, 12, 137–144 CrossRef.
- Y. Y. Zheng, J. Xu, Y. Zhang, X. S. Yang, Y. J. Zhang and Y. Y. Shang, New J. Chem., 2018, 42, 150–160 RSC.
- X. Wu, Z. C. Han, X. Zheng, S. Y. Yao, X. Yang and T. Y. Zhai, Nano Energy, 2017, 31, 410–418 CrossRef CAS.
- M. H. Yu, D. Lin, H. B. Feng, Y. X. Zeng, Y. X. Tong and X. H. Lu, Angew. Chem., Int. Ed., 2017, 56, 5454–5459 CrossRef CAS PubMed.
- Y. A. Kumar, S. S. Rao, D. Punnoose, C. V. Thulasivarma, C. V. V. M. Gopi, K. Prabakar and H. J. Kim, R. Soc. Open Sci., 2017, 4, 170427 CrossRef PubMed.
- Z. Gao, W. L. Yang, J. Wang, B. Wang, Z. S. Li, Q. Liu, M. L. Zhang and L. H. Liu, Energy Fuels, 2013, 27, 568–575 CrossRef CAS.
- F. S. Wu, X. H. Wang, W. R. Zheng, H. W. Gao, C. Hao and C. W. Ge, Electrochim. Acta, 2017, 245, 685–695 CrossRef CAS.
- Y. A. Kumar and H.-J. Kim, New J. Chem., 2018, 42, 19971 RSC.
- Y. Zheng, Z. Li, J. Xu, T. Wang and X. Liu, Nano Energy, 2015, 20, 94–107 CrossRef.
- L. L. Zhang and X. Zhao, Chem. Soc. Rev., 2009, 38, 2520–2531 RSC.
- F. Markoulidis, C. Lei, C. Lekakou, D. Duff, S. Khalil, B. Martorana and I. Cannavaro, Carbon, 2014, 68, 58–66 CrossRef CAS.
- D. Bhattacharjya, M.-S. Kim, T. S. Bae and J. S. Yu, J. Power Sources, 2013, 244, 799–805 CrossRef CAS.
- J. Yin, D. Zhang, J. Zhao, X. Wang, H. Zhu and C. Wang, Electrochim. Acta, 2014, 136, 504–512 CrossRef CAS.
- A. K. Yedluri and H.-J. Kim, Dalton Trans., 2018, 47, 15545–15554 RSC.
- W. Wei, L. Mi, Y. Gao, Z. Zheng, W. Chen and X. Guan, Chem. Mater., 2014, 26, 3418–3426 CrossRef CAS.
- W. Wei, L. Mi, Y. Gao, Z. Zheng, W. Chen and X. Guan, Chem. Mater., 2014, 26, 3418–3426 CrossRef CAS.
- L. Mi, W. Wei, S. Huang, S. Cui, W. Zhang, H. Hou and W. Chen, J. Mater. Chem. A, 2015, 3, 20973–20982 RSC.
- W. Wei, S. Cui, L. Ding, L. Mi, W. Chen and X. Hu, ACS Appl. Mater. Interfaces, 2017, 9, 40655–40670 CrossRef CAS PubMed.
- F. Zou, X. Hu, Z. Li, L. Qie, C. Hu, R. Zeng, Y. Jiang and Y. Huang, Adv. Mater., 2014, 26, 6622–6628 CrossRef CAS PubMed.
- Y. A. Kumar, S. S. Rao, D. Punnoose, C. V. Thulasivarma, C. V. V. M. Gopi, K. Prabakar and H. J. Kim, R. Soc. Open Sci., 2017, 4, 170427 CrossRef PubMed.
- N. Choudhary, C. Li, J. Moore, N. Nagaiah, L. Zhai, Y. Jung and J. Thomas, Adv. Mater., 2017, 29, 1605336 CrossRef PubMed.
- J. Zhao, Z. Li, M. Zhang, A. Meng and Q. Li, ACS Sustainable Chem. Eng., 2016, 4, 3598–3608 CrossRef CAS.
- L. Li, L. Tan, G. Li, Y. Zhang and L. Liu, Langmuir, 2017, 33, 12087–12094 CrossRef CAS PubMed.
- Y. A. Kumar, S. S. Rao, D. Punnoose, C. V. Thulasivarma, C. V. V. M. Gopi, K. Prabakar and H. J. Kim, R. Soc. Open Sci., 2017, 4, 170427 CrossRef PubMed.
- M. M. Yao, Z. H. Hu, Z. J. Xu, Y. F. Liu, P. P. Liu and Q. Zhang, Electrochim. Acta, 2015, 158, 96–104 CrossRef CAS.
- P. Deng, H. Y. Zhang, Y. M. Chen, Z. H. Li, Z. K. Huang, X. F. Xu, Y. Y. Li and Z. Shi, J. Alloys Compd., 2015, 644, 165–171 CrossRef CAS.
- H. Yi, H. W. Wang, Y. T. Jing, T. Q. Peng and X. F. Wang, J. Power Sources, 2015, 285, 281–290 CrossRef CAS.
- A. C. Nwanya, S. U. Offiah, I. C. Amaechi, S. Agbo, S. C. Ezugwu, B. T. Sone, R. U. Osuji, M. Maaza and F. I. Ezema, Electrochim. Acta, 2015, 171, 128–141 CrossRef CAS.
- T. V. Thi, A. K. Rai, J. Gim and J. Kim, J. Power Sources, 2015, 292, 23–30 CrossRef CAS.
- M. J. Jing, C. W. Wang, H. S. Hou, Z. B. Wu, Y. R. Zhu, Y. C. Yang, X. N. Jia, Y. Zhang and X. B. Ji, J. Power Sources, 2015, 298, 241–248 CrossRef CAS.
- Y. A. Kumar and H.-J. Kim, Energies, 2018, 11, 3285 CrossRef CAS.
- M. J. Pang, G. H. Long, S. Jiang, Y. Ji, W. Han, B. Wang, X. L. Xi, Y. L. Xi, D. X. Wang and F. Z. Xu, Chem. Eng. J., 2015, 280, 377–384 CrossRef CAS.
- Y. Zheng, Z. Lin, W. Chen, B. Liang and H. Du, J. Mater. Chem. A, 2017, 5, 5886–5894 RSC.
- L. Huang, D. Chen, Y. Ding, S. Feng, Z. L. Wang and M. Liu, Nano Lett., 2013, 13, 3135–3139 CrossRef CAS PubMed.
- C. Zhang, X. Geng, S. Tang, M. Deng and Y. Du, J. Mater. Chem. A, 2017, 5, 5912–5919 RSC.
- M. Yu, J. Chen, J. Liu, S. Li and Y. Ma, Electrochim. Acta, 2015, 151, 99–108 CrossRef CAS.
- G. Nagaraju, S. C. Sekhar and J. S. Yu, Adv. Energy Mater., 2017, 7, 1702201 Search PubMed.
- R. Zou, K. Xu, T. Wang, G. He, Q. Liu, X. Liu, Z. Zhang and J. Hu, J. Mater. Chem. A, 2013, 1, 8560 RSC.
- Z. Gu and X. Zhang, J. Mater. Chem. A, 2016, 4, 8249 RSC.
- R. Zou, K. Xu, T. Wang, G. He, Q. Liu, X. Liu, Z. Zhang and J. Hu, J. Mater. Chem. A, 2013, 1, 8560 RSC.
- L. Wang, X. Jiao, P. Liu, Y. Ouyang, X. Xia, W. Lei and Q. Hao, Appl. Surf. Sci., 2018, 427, 174–181 CrossRef CAS.
- R. Ding, L. Qi, M. Jia and H. Wang, Electrochim. Acta, 2013, 107, 494–502 CrossRef CAS.
- C. T. Hsu and C. C. Hu, J. Power Sources, 2013, 242, 662–671 CrossRef CAS.
- H. Chen, J. Jiang, L. Zhang, T. Qi, D. Xia and H. Wan, J. Power Sources, 2014, 248, 28–36 CrossRef CAS.
- G. Gao, H. B. Wu, S. Ding, L. M. Liu and X. W. Lou, Small, 2015, 11, 804–808 CrossRef CAS PubMed.
- Y. Zhao, X. Zhang, J. He, L. Zhang, M. Xia and F. Gao, Electrochim. Acta, 2015, 174, 51–56 CrossRef CAS.
- K. H. Oh, G. S. Gund and H. S. Park, J. Mater. Chem. A, 2018, 6, 22106–22114 RSC.
- Y. Zhu, X. Ji, R. Yin, Z. Hu, X. Qiu, Z. Wu and Y. Liu, RSC Adv., 2017, 7, 11123 RSC.
- R. Zou, K. Xu, T. Wang, G. He, Q. Liu, X. Liu, Z. Zhang and J. Hu, J. Mater. Chem. A, 2013, 1, 8560 RSC.
- C. Young, R. R. Salunkhe, S. M. Alshehri, T. Ahamad, Z. Huang, J. Henzie and Y. Yamauchi, J. Mater. Chem. A, 2017, 5, 11834–11839 RSC.
- M. Yu, J. Chen, J. Liu, S. Li, Y. Ma, J. Zhang and J. An, Electrochim. Acta, 2015, 151, 99–108 CrossRef CAS.
- C. T. Hsu and C. C. Hu, J. Power Sources, 2013, 15, 662–671 CrossRef.
- X. Wang, W. S. Liu, X. Lu and P. S. Lee, J. Mater. Chem., 2012, 22, 23114–23119 RSC.
- Y. Lei, J. Li, Y. Wang, L. Gu, Y. Chang, H. Yuan and D. Xiao, ACS Appl. Mater. Interfaces, 2014, 6, 1773–1780 CrossRef CAS PubMed.
- C. Hao, S. Zhou, J. Wang, X. Wang, H. Gao and C. Ge, Ind. Eng. Chem. Res., 2018, 57, 2517–2525 CrossRef CAS.
- L. Yu, G. Zhang, C. Yuan and X. W. Lou, Chem. Commun., 2013, 49, 137–139 RSC.
- X. Liu, J. Liu and X. Sun, J. Mater. Chem. A, 2015, 3, 13900–13905 RSC.
- D. Cai, B. Liu, D. Wang, L. Wang, Y. Liu, H. Li, Y. Wang, Q. Li and T. Wang, J. Mater. Chem. A, 2014, 2, 4954–4960 RSC.
- J. Du, G. Zhou, H. Zhang, C. Cheng, J. Ma, W. Wei, L. Chen and T. Wang, ACS Appl. Mater. Interfaces, 2013, 5, 7405–7409 CrossRef CAS PubMed.
- L. Huang, W. Zhang, J. Xiang, H. Xu, G. Li and Y. Huang, Sci. Rep., 2016, 6, 31465 CrossRef CAS PubMed.
Footnote |
† Electronic supplementary information (ESI) available. See DOI: 10.1039/c8ra09081e |
|
This journal is © The Royal Society of Chemistry 2019 |
Click here to see how this site uses Cookies. View our privacy policy here.