DOI:
10.1039/C8QM00620B
(Research Article)
Mater. Chem. Front., 2019,
3, 496-504
A cascade-type electron extraction design for efficient low-bandgap perovskite solar cells based on a conventional structure with suppressed open-circuit voltage loss†
Received
30th November 2018
, Accepted 22nd January 2019
First published on 25th January 2019
Abstract
The tandem architecture for perovskite solar cells has proven successful in promoting the development of such cells. A low-bandgap perovskite solar cell, which typically acts as a back cell, is one of the critical components for tandem perovskite solar cells. However, nowadays, highly efficient low-bandgap perovskite solar cells are mostly based on the inverted structure, which restricts the development of conventional perovskite tandem cells. Therefore, efficient low-bandgap perovskite solar cells based on the conventional structure need to be developed to further extend the availability of device architectures and interfacial materials for tandem cells. Here, by modifying the electron transport materials, we successfully demonstrated an efficient low-bandgap perovskite solar cell based on the conventional structure. A ZnO/SnO2/C60-SAM tri-layer was used to engineer the energy level alignment of electron transport layers to reduce the energy loss occurring at the interface and simultaneously suppress the interfacial recombination and improve the charge extraction, resulting in a reduced open-circuit voltage loss for the device. Finally, our low-bandgap perovskite solar cells achieved a power conversion efficiency of 13.8%, which is the record result for conventional device structures to date.
Introduction
Since organic–inorganic hybrid perovskite solar cells (PVSCs) were first reported in 2009, the power conversion efficiency (PCE) of this kind of solar cell has skyrocketed to a certified record efficiency of 23.7%, approaching that of crystalline silicon solar cells.1 The rapid development of PVSCs can be ascribed to their excellent photovoltaic properties and the choice of various compositions in the perovskite family.2–5 However, the development of single-junction PVSCs has slowed down in recent years and a new device structure needs to be developed to overcome the bottleneck. As is well known, the tandem solar cell is a proven strategy to break through the Shockley–Queisser limit for single cells, thereby promoting the development of PVSCs. The two typical device structures for tandem PVSCs are the inverted structure and conventional structure. In inverted tandem PVSCs, the wide-bandgap front cell and low-bandgap back cell are in an inverted arrangement (ITO/hole transport layer (HTL)/perovskite/electron transport layer (ETL)/electrode, p–i–n structure) while in conventional tandem PVSCs, the front cell and back cell have a conventional arrangement (n–i–p structure). Therefore, both highly efficient wide-bandgap front cells and low-bandgap back cells are needed in both inverted and conventional structures, for different architectures of the tandem PVSCs. In the perovskite family, bandgaps ranging from around 1.2 to 1.9 eV can be easily tuned through composition engineering.5,6 Nowadays, highly efficient wide-bandgap PVSCs (around 1.7 to 1.9 eV) based on inverted and conventional structures have been successfully demonstrated, whereas the low-bandgap PVSCs (around 1.2 eV) still suffer from inferior performance, particularly those with the conventional structure.5,7–12 Therefore, it is an urgent target to develop efficient low-bandgap PVSCs based on the conventional structure to further extend the availability of device architectures and interfacial materials for tandem cells.
In organic–inorganic hybrid perovskites, when using tin (Sn) to partially replace lead (Pb) in FAxMA1−xPbI3 (0 ≤ x ≤ 1), where FA+ and MA+ represent formamidinium and methylammonium cations, respectively, the bandgap of perovskite can be easily tuned from around 1.6 to 1.2 eV, leading to an expansion of the absorption edge from around 775 to 1050 nm.13 The lowest-bandgap perovskites were achieved when the amounts of Sn and Pb were stoichiometrically identical in the perovskite lattice (i.e., FAxMA1−xSn0.5Pb0.5I3).5,11,13–16 In the past two years, a great effort has been made toward developing low-bandgap FAxMA1−xSn0.5Pb0.5I3 PVSCs based on the inverted structure, leading to a substantial improvement in PCE from 10% to around 18%.17–23 However, compared with the inverted structure, conventional FAxMA1−xSn0.5Pb0.5I3 PVSCs have attracted less attention. In 2014, Hayase and co-workers for the first time demonstrated a low-bandgap PVSC based on the conventional structure with absorption up to 1060 nm by forming MASn0.5Pb0.5I3.11 Their device obtained a PCE of 4.18%, and this value was improved to 7.27% by Kanatzidis and co-workers several months later, which still stands as the best result for low-bandgap PVSCs based on the conventional structure to date, to the best of our knowledge.5 One reason for the inferior performances of conventional low-bandgap PVSCs was the high open-circuit voltage (Voc) loss, which is defined by the difference between the Voc of the PVSCs and the optical bandgap (Eg) of the active layer divided by the elementary charge (q) (i.e. Eg/q − Voc).24,25 The high Voc loss probably resulted from the large energy level mismatching between the conduction band (CB) of the low-bandgap perovskites and TiO2.11 Therefore, a new ETL should be considered to substitute the high-temperature-processed TiO2, in order to provide a better energy level alignment to suppress the Voc loss and hence improve the device performance. Considering its electron mobility, light transparency and low-temperature processability, SnO2, which is widely used for high-performance PVSCs, is a good candidate for TiO2 substitution.26–28 However, the CB mismatching between SnO2 and perovskites could also cause a large Voc loss, which therefore necessitates a modification of the energy level of SnO2.
Herein, a self-assembled monolayer, C60-SAM, was used to modify the energy level of the SnO2 ETL. It has been reported that modification by C60-SAM could up-shift the CB of SnO2 from −4.5 eV to −4.1 eV, which matches well with the CB of low-bandgap FA0.5MA0.5Sn0.5Pb0.5I3 perovskite (−4.0 eV).29,30 In this case, the low-bandgap PVSCs obtained an improved Voc from 0.35 V to 0.48 V, with an improved PCE from 5.7% to 9.0%. Subsequently, we inserted a layer of ZnO to form a cascade-type ETL of ZnO/SnO2/C60-SAM to further reduce the energy level mismatching between SnO2/C60-SAM and indium tin oxide (ITO) and to suppress the interfacial recombination as well, which hence further reduced the Voc loss for the whole device. Eventually, we demonstrated a highly-efficient low-bandgap FA0.5MA0.5Sn0.5Pb0.5I3 PVSC with PCE greater than 13%. To the best of our knowledge, this is the most efficient low-bandgap PVSC based on the conventional device structure to date.
Experimental
Precursor solution preparation
The FASnI3 precursor solution was prepared by dissolving 372 mg of SnI2, 172 mg of formamidinium iodide (FAI) and 15.6 mg of SnF2 in 1 mL of mixed solvent of N,N-dimethylmethanamide (DMF) and dimethyl sulfoxide (DMSO), where the ratio of DMF
:
DMSO was 4
:
1. The MAPbI3 precursor solution was prepared by dissolving 461 mg of PbI2 and 159 mg of methylammonium iodide (MAI) in 0.7 mL of the aforementioned mixed solvent. The FA0.5MA0.5Sn0.5Pb0.5I3 precursor solution was obtained by mixing stoichiometrically identical amounts of FASnI3 and MAPbI3 precursor solutions, followed by stirring for 12 hours.
Device fabrication
ITO glass substrates were sequentially washed with isopropanol, acetone, distilled water and isopropanol, then dried in an oven. For device fabrication, ITO glass was treated by plasma treatment, followed by the deposition of 10 nm-thick ZnO nanoparticles (purchased from Sigma Aldrich) through spin-coating. Subsequently, 40 nm-thick SnO2 nanoparticles (purchased from Alfa Aesar) were spin-coated on the ITO/ZnO substrate, followed by annealing at 150 °C for 30 min. For SnO2 modification, C60-SAM solution (1.5 mg mL−1 in chlorobenzene) was spin-coated above the SnO2 layer at a spin speed of 4000 rpm for 30 s, and then spin-treated with chlorobenzene to wash away the residual C60-SAM. From the X-ray photoelectron spectroscopy (XPS) and atomic force microscope (AFM) measurements shown in Fig. S1 (ESI†), we confirmed the existence of C60-SAM above the SnO2 layer via the appearance of the N 1s signal, and observed that the C60-SAM-deposition process had no influence on the SnO2 morphology. Finally, the FA0.5MA0.5Sn0.5Pb0.5I3 precursor solution was spin-coated above the ITO/ZnO/SnO2/C60-SAM layer to form a 500 nm-thick perovskite film, followed by the deposition of a 40 nm-thick poly(3-hexylthiophene-2,5-diyl) (P3HT) layer, 10 nm-thick MoO3 layer and 100 nm-thick Ag electrode.
Perovskite film characterization
Ultraviolet-visible light absorption spectra were recorded on an HP8453 spectrophotometer. Scanning electron microscope (SEM) images were obtained by a Zeiss EVO 18 SEM. The crystalline structure of the perovskite films was investigated by an X-ray diffractometer (PANalytical X’pert PRO) equipped with a Cu-Kα X-ray tube. The XPS data were obtained by using ESCALAB 250Xi equipment. Ultraviolet photoelectron spectroscopy (UPS) data were obtained using a K-ALPHA+ instrument. AFM images were obtained by a Bruker Multimode 8 scanning probe microscope.
Perovskite solar cell characterization
The current density–voltage (J–V) characteristics for the devices were measured under conditions of 100 mW cm−2 air mass, 1.5 global (AM 1.5G) illumination with a solar simulator (Taiwan, Enlitech, SS-F5). The light intensity was calibrated with a National Renewable Energy Laboratory-calibrated silicon photodiode using a KG5 filter. The external quantum efficiency (EQE) of the devices was measured on a commercial QE measurement system (QE-R3011, Enlitech). For the transient photovoltage (TPV) measurements, the device was serially connected to a digital oscilloscope (Tektronix TDS 3052C), and the oscilloscope's input impedance was set to 1 MΩ to form open-circuit conditions. The TPV was measured under 0.3 sun illumination. An attenuated laser pulse (0.54 μJ cm−2; 530 nm) was used as a small perturbation to the device's background illumination. The laser pulses were generated from an optical parametric amplifier (TOPAS-Prime) pumped by a mode-locked Ti:sapphire oscillator-seeded regenerative amplifier, with a pulse energy of 1.3 mJ at 800 nm and a repetition rate of 1 kHz (SpectraPhysics Spitfire Ace). The time resolution of the overall measurement was approximately 1 ns. The light-dependent Voc measurements were performed under the J–V measurement system, and the light intensity was modulated from 0.1 sun to 1 sun. The transient photocurrent (TPC) and electrochemical impedance spectroscopy (EIS) measurements were carried out using a Paios 4.0 Measurement Instrument (FLUXiM AG, Switzerland).
Results and discussion
Although SnO2 has been successfully applied in medium-bandgap PVSCs, there has been no report of low-bandgap PVSCs based on a SnO2 ETL in a conventional structure. In our study, the low-bandgap perovskite of FA0.5MA0.5Sn0.5Pb0.5I3 was chosen as the active layer to absorb light up to around 1050 nm. The valence band (VB) of FA0.5MA0.5Sn0.5Pb0.5I3 perovskite was measured as −5.2 eV by UPS as shown in Fig. S2 (ESI†), and the CB of −4.0 eV was confirmed by its optical bandgap. The large energy offset of CBs between SnO2 (−4.5 eV) and FA0.5MA0.5Sn0.5Pb0.5I3 perovskite (−4.0 eV) can be expected to cause a large Voc loss in the device.31–34 Therefore, energy level modification of SnO2 is needed in this case.
Self-assembled monolayers are widely used as modifying agents not only to modulate the surface tension, surface energy and energy level of metal oxides, but also to passivate the surface traps of metal oxides.29,35–38 Among all of the known modifiers, C60-SAM, whose molecular structure is shown in Fig. 1b, has proven to be one of the most successful, up-shifting the CB of SnO2 to −4.1 eV, and thereby reducing the CB offset between the ETL and FA0.5MA0.5Sn0.5Pb0.5I3 perovskite.29 The carboxyl end-groups in C60-SAM can strongly bond with SnO2 to form a self-assembled monolayer, leaving the C60 bodies exposed on the exterior, which can affect the morphologies and crystallinity of the upper perovskite layer.38,39
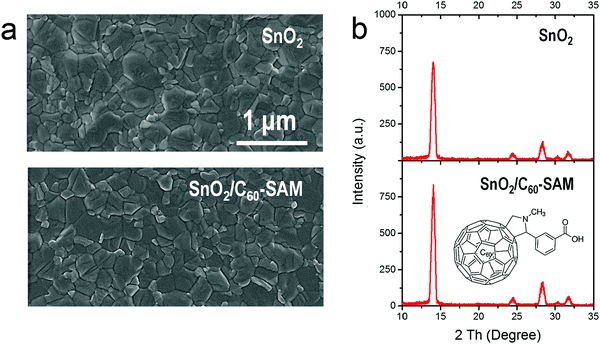 |
| Fig. 1 (a) SEM images of FA0.5MA0.5Sn0.5Pb0.5I3 film above SnO2 and SnO2/C60-SAM substrates. (b) XRD pattern of FA0.5MA0.5Sn0.5Pb0.5I3 film above SnO2 and SnO2/C60-SAM substrates. The molecular structure of C60-SAM is also shown. | |
Morphologies and crystal qualities
Fig. 1a shows top-view SEM images of FA0.5MA0.5Sn0.5Pb0.5I3 perovskite films prepared on SnO2 and SnO2/C60-SAM substrates, respectively. In both cases full-coverage films with similar morphologies were obtained, suggesting that no negative impact on the perovskite film morphology was caused by the modification with C60-SAM. Subsequently, X-ray diffraction (XRD) measurements were performed to reveal the crystal quality of the perovskite films. As can be seen in Fig. 1b, compared with the perovskite film fabricated above the SnO2 substrate, the perovskite film fabricated above the SnO2/C60-SAM substrate obtained better crystallinity, which in turn improved the absorption of light by the FA0.5MA0.5Sn0.5Pb0.5I3 film, as shown in Fig. S3 (ESI†). We attributed the better crystallinity of the perovskite film to the passivation effect of C60-SAM toward the SnO2 surface, which has been widely reported previously.30,39,40 The removal of defects on the SnO2 surface facilitated a better growth of the upper perovskite layer.33,38
To further confirm this, we estimated the trap density of the ETL/perovskite film by studying an electron-only device based on the device structure of ITO/ETL/perovskite/PCBM/Ag. As shown in Fig. 2, the overall trap density of SnO2/C60-SAM/FA0.5MA0.5Sn0.5Pb0.5I3 film is 2.0 × 1015 cm−3, which is lower than that of the SnO2/FA0.5MA0.5Sn0.5Pb0.5I3 film (3.1 × 1015 cm−3). This result can be attributed to the reduced trap density on the SnO2 surface owing to passivation by C60-SAM and the improved perovskite crystal quality. Films with better absorption and lower trap density can be expected to be more suitable for photovoltaic applications.
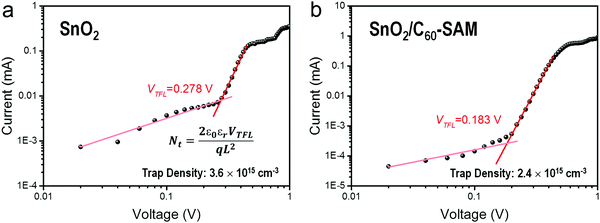 |
| Fig. 2 Trap density evaluation by dark current–voltage measurements of the electron-only device with the device structure of ITO/ETL/perovskite/PCBM/Ag, where ETL is SnO2 in (a) and SnO2/C60-SAM in (b). The pink lines represent the Ohmic regime of each case, and the red lines indicate the trap-filled limit (TFL) regime with the onset voltage (VTFL). The trap density (Nt) of FA0.5MA0.5Sn0.5Pb0.5I3 thin films with different ETLs can be extracted by the equation displayed in (a), where ε0, q and L represent vacuum permittivity, elementary charge and thickness of the perovskite film, respectively; εr is the average relative dielectric constant, which is generally assigned as 25.41 | |
Device properties
To estimate the influence of SnO2 modification by C60-SAM on the photovoltaic properties, PVSCs based on the device architecture of ITO/ETL/FA0.5MA0.5Sn0.5Pb0.5I3/P3HT/MoO3/Ag were fabricated, as shown in Fig. 3a. Firstly, we compared the ETLs of SnO2 and SnO2/C60-SAM. The J–V curves of the PVSCs with different ETLs are shown in Fig. 3b and their performances are summarised in Table 1. The EQEs of both cases are shown in Fig. S4 (ESI†) and the deviations between the short-circuit current density (Jsc) measured by the solar simulator and the integrated Jsc calculated from the EQE spectra are less than 5%. The PVSC based on the bare SnO2 ETL suffered from an inferior PCE of 5.7% with an ultra-poor Voc of 0.35 V and small fill factor (FF) of 59%, resulting in a high Voc loss of 0.85 V. However, after the SnO2 ETL was modified by C60-SAM, the PVSC based on the SnO2/C60-SAM ETL obtained an improved Voc of 0.48 V and an improved FF of 65.5%. We attributed this result to three possible reasons. First, the C60-SAM passivated the surface traps on the SnO2 surface, which weakened the trap-assisted recombination at the interface between the ETL and perovskite, as we will discuss later. Second, the perovskite film based on the SnO2/C60-SAM substrate obtained better crystal quality. Third, the SnO2/C60-SAM obtained a higher CB (−4.1 eV) than that of bare SnO2 (−4.5 eV), which reduced the energy offset between the ETL and perovskite and hence suppressed the energy loss at the interface.
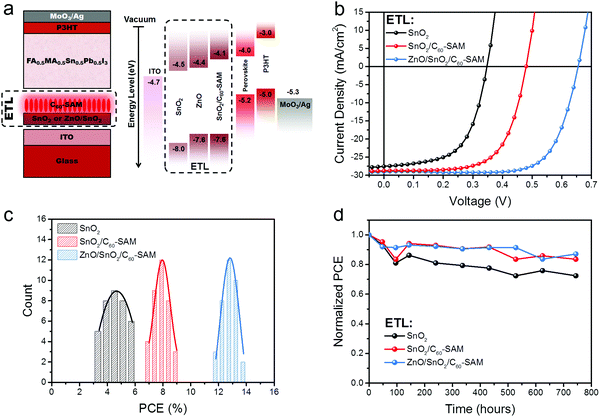 |
| Fig. 3 (a) Device architecture and energy level of each layer in the device. (b) J–V curves of PVSCs with different ETLs. (c) Statistical performances of 36 PVSCs with different ETLs. (d) Stability measurements of PVSCs with different ETLs, stored in an N2-filled glovebox. | |
Table 1 Performances of PVSCs with the structure ITO/ETL/FA0.5MA0.5Sn0.5Pb0.5I3/P3HT/MoO3/Ag
ETL |
V
oc (V) |
J
sc (mA cm−2) |
FF (%) |
PCE (%) |
V
oc loss (V) |
SnO2 |
0.35 |
27.6 |
59.0 |
5.7 |
0.85 |
SnO2/C60-SAM |
0.48 |
28.7 |
65.6 |
9.0 |
0.72 |
ZnO/SnO2/C60-SAM |
0.66 |
29.0 |
72.5 |
13.8 |
0.54 |
However, as seen in the energy level diagram in Fig. 3a, there was still a large energy offset between the SnO2/C60-SAM and ITO layers, which must be the main reason why the device still suffered from a large Voc loss of 0.72 V. Clearly, it can be expected that if one extra ETL with a CB around −4.4 eV could be inserted between the SnO2/C60-SAM and ITO layers, this would further reduce the Voc loss for the overall device. Among all of the widely used ETLs, ZnO nanoparticles, which are low-temperature solution-processable, are one of the most appropriate because of their high electron mobility and satisfactory CB of −4.4 eV.35,42 We therefore inserted a ZnO layer between ITO and SnO2/C60-SAM to fabricate a conventional PVSC based on the device structure of ITO/ZnO/SnO2/C60-SAM/FA0.5MA0.5Sn0.5Pb0.5I3/P3HT/MoO3/Ag. As shown in Fig. 3b and Table 1, we found that the formation of a cascade-type ETL of ZnO/SnO2/C60-SAM suppressed the Voc loss notably further to 0.54 V, resulting in a better FF of 72.5% and a dramatically improved PCE of 13.8%. To the best of our knowledge, this is the best result so far for low-bandgap PVSCs based on the conventional device structure. Moreover, as seen in Fig. 3c and d, the device based on the ETL of SnO2/C60-SAM and ZnO/SnO2/C60-SAM obtained better reproducibility and stability compared with the device based on the ETL of bare SnO2. This can again be attributed to the reduced number of trap states on the SnO2 surface and the better crystal quality of the perovskite film. The best device maintained almost 90% of its initial performance when stored in an N2-filled glovebox for 1 month.
Charge recombination and extraction properties
Besides an appropriate energy level alignment of each layer, the recombination properties in PVSCs also strongly affects the total Voc loss of the device.43 In a PVSC, typically, trap-assisted recombination dominates the monomolecular recombination and causes a severe Voc loss in the device.44 Such recombination becomes severe at perovskite crystal surfaces and the interface between the perovskite and ETL/HTL.45,46 Therefore, it is important to passivate the surface traps in perovskites as well as in ETL/HTL materials, and to spatially separate the photo-generated holes and electrons into different materials, aiming to prolong the recombination time. The overall monomolecular recombination of the device can be measured via the light-intensity-dependent Voc of the device (the relationship between Voc and the natural logarithm of light intensity, i.e. ln(I)).47 Generally, if the slope of this relationship has a value around 1 kbT/q, this represents that the recombination process is dominated by bimolecular recombination (where kb is the Boltzmann constant and T is the temperature), while the slope tends to be 2 kbT/q when monomolecular recombination (or trap-assisted recombination) is involved.48 As seen in Fig. 4a, the device with the ZnO/SnO2/C60-SAM ETL obtained the smallest slope, tending to 1 kbT/q, compared with the larger slopes of the devices with ETLs of SnO2/C60-SAM (slope of 1.37 kbT/q) and SnO2 (slope of 1.52 kbT/q). This result illustrated that the device with the bare SnO2 ETL suffered from the most severe monomolecular recombination, while such recombination was suppressed after the C60-SAM modification and an even better suppression was achieved by using the cascade-type ETL of ZnO/SnO2/C60-SAM.
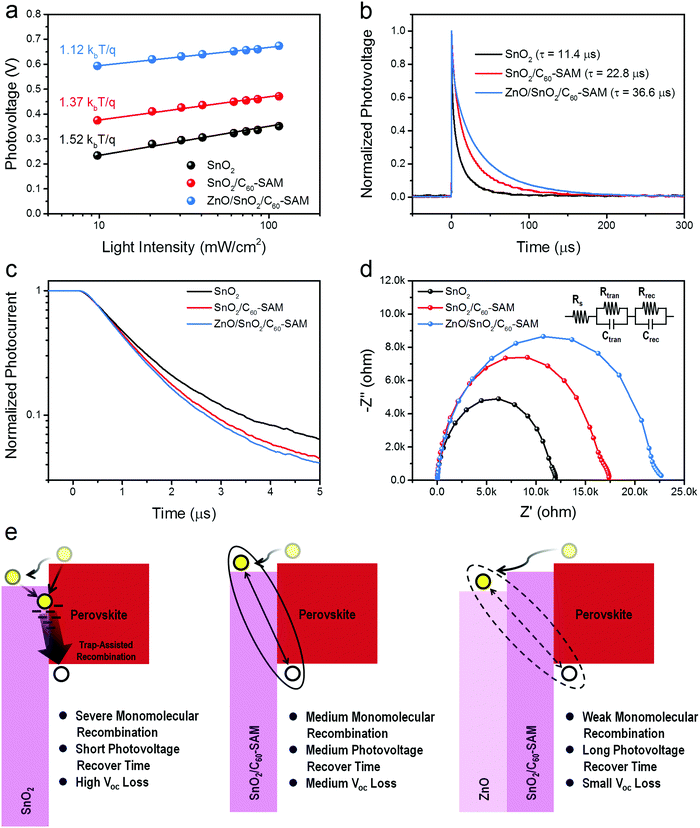 |
| Fig. 4 Charge recombination and extraction characterization of FA0.5MA0.5Sn0.5Pb0.5I3 solar cells with different ETLs: (a) photovoltage as a function of light intensity and (b) TPV measurements. (c) TPC measurements and (d) EIS measurements. For the devices with bare SnO2, SnO2/C60-SAM and ZnO/SnO2/C60-SAM ETLs, the extracted Rtran values are 1.9 kΩ, 1.0 kΩ and 0.8 kΩ, respectively, while the extracted Rrec values are 11.2 kΩ, 15.8 kΩ and 19.5 kΩ, respectively. (e) Schematic diagrams of the charge recombination situations in cases of different ETLs. | |
To provide a deeper understanding of the recombination properties of PVSCs with different ETLs, TPV measurements were also conducted. The decay of photovoltage results from the recombination of photo-excited electrons and holes, and therefore a longer photovoltage decay time reflects a slower charge recombination in the device.49Fig. 4b shows the normalized photovoltage as a function of time in PVSCs with different ETLs, and the lifetime τ of each case was extracted by single exponential fitting. The device with the bare SnO2 ETL obtained the shortest lifetime of 11.4 μs, while longer lifetimes of 22.8 μs and 36.6 μs were achieved in the SnO2/C60-SAM and ZnO/SnO2/C60-SAM ETL cases, respectively. The fact that the longest lifetime was achieved by the ZnO/SnO2/C60-SAM ETL illustrated that the photogenerated charge carriers in this case had the most favourable opportunity to be transported to the electrodes before they recombined with each other. Both the weak monomolecular recombination and the small charge recombination rate strongly support the significant Voc improvement in the device.
Charge extraction properties are also important for a high-performance PVSC to reach low Voc loss, because a faster charge extraction also assists the suppression of charge recombination. TPC is a suitable technique to investigate the charge extraction properties within the whole device. Fig. 4c shows the TPC results of PVSCs with different ETLs. It is obvious that a faster decay of photocurrent occurred in the device with the ZnO/SnO2/C60-SAM ETL compared with the other two cases. These results suggest that the cascade-type ETL is efficient for charge extraction in the device, which can be attributed to the better energy-level alignment and the suppressed interfacial recombination. To cross check these results, EIS was conducted to study the interfacial properties of the low-bandgap PVSCs, including charge extraction and charge recombination properties. Fig. 4d shows the Nyquist plots from the impedance spectra results for the devices with different ETLs, and the circuit model we used for data analysis. The extracted transport resistance (Rtran) values are 1.9 kΩ, 1.0 kΩ and 0.8 kΩ for the device with bare SnO2, SnO2/C60-SAM and ZnO/SnO2/C60-SAM ETLs, respectively. A smaller Rtran value reflects fewer barriers for charge transport, therefore, the device with ZnO/SnO2/C60-SAM ETL obtained the best charge transport property, which was also consistent with the TPC results. In addition, the extracted recombination resistance (Rrec) values are 11.2 kΩ, 15.8 kΩ and 19.5 kΩ for the device with bare SnO2, SnO2/C60-SAM and ZnO/SnO2/C60-SAM ETLs, respectively. A larger Rrec value reflects larger barriers for charge recombination, therefore, the photoexcited charge carriers are the most difficult to recombine in the device with the ZnO/SnO2/C60-SAM ETL, compared with the other two cases.
Considering together the passivation effect of C60-SAM, the cascade-type ETL, the charge recombination and extraction properties of the devices, we schematically illustrate in Fig. 4e the factors affecting recombination, and their consequences for device performance, in the devices with different ETLs. In the device with the bare SnO2 ETL, the surface defects of SnO2 become traps at the interface between the SnO2 and perovskite. The photo-excited electrons in the perovskite or the transferred electrons in SnO2 can be trapped by such surface trap states, leading to severe monomolecular recombination and fast recombination rate for the photo-generated charge carriers. However, in the device with the SnO2/C60-SAM ETL, the surface traps of SnO2 have been passivated by C60-SAM. The removal of trap states at the interface allows successful electron transfer from the perovskite to SnO2, separating the photo-excited electrons and holes, and therefore suppressing the monomolecular recombination and slows down the recombination rate. Finally, in the device with the ZnO/SnO2/C60-SAM ETL, photo-excited electrons are easily transferred from the perovskite to the ZnO layer because of the gradient energy level alignment. The spatial separation of photo-excited electrons and holes dramatically reduces their recombination at the interface, leading to a small Voc loss. A similar phenomenon was previously observed while using PCBM/C60 as the ETL to form a spike structure that dramatically suppressed the interfacial recombination through the spatial separation of photo-excited electrons and holes.20
Conclusions
Using a cascade-type tri-layer of ZnO/SnO2/C60-SAM as the ETL, we successfully demonstrated a highly efficient low-bandgap PVSC with a PCE of 13.8%. To the best of our knowledge, this is the best result thus far for any conventional low-bandgap PVSC, being almost two-times higher than the previous highest published PCE to date, and provides the possibility of an efficient conventional tandem PVSC. We consider the extremely large Voc loss to be one of the main causes of the inferior performance of low-bandgap PVSCs based on the conventional structure, while our findings prove that the utilization of a cascade-type ETL (i.e. ZnO/SnO2/C60-SAM) is an efficient strategy to reduce the Voc loss. First, in the context of energy level alignment, the C60-SAM up-shifted the energy level of SnO2 from −4.5 eV to −4.1 eV, which minimized the offset of CBs between SnO2 and perovskite, and hence reduced the energy loss. In addition, the larger energy offset between ITO and SnO2/C60-SAM was also reduced by the insertion of ZnO. Such a cascade-type ETL provided a smooth pathway for the electrons, facilitating a better electron extraction and a low Voc loss in the device. Second, in the context of recombination suppression, the C60-SAM passivated the surface traps of SnO2 and consequently reduced the trap-assisted recombination at the interface. Moreover, the fast electron transfer from the perovskite to the additional ZnO layer spatially separated the photo-excited electrons and holes, leading to a further suppression of interfacial recombination, which also facilitated a low Voc loss in the device. However, the Voc loss of 0.54 V is still higher than those in pure Pb-based PVSCs (∼0.45 V).50 Therefore, there is still room to further develop low-bandgap PVSCs based on the conventional structure.
Conflicts of interest
The authors declare no competing interests.
Acknowledgements
This study was financially supported by the Ministry of Science and Technology of the People's Republic of China (No. 2017YFA0206600), the Science and Technology Program of Guangzhou, China (No. 201607020010) and the National Natural Science Foundation of China (No. 21761132001, 51573057 and 91733302).
References
- National Renewable Energy Laboratory (NREL), https://www.nrel.gov/pv/assets/images/effciency-chart.png, accessed September 2018.
- C. C. Stoumpos, C. D. Malliakas and M. G. Kanatzidis, Semiconducting tin and lead iodide perovskites with organic cations: phase transitions, high mobilities, and near-infrared photoluminescent properties, Inorg. Chem., 2013, 52, 9019–9038 CrossRef CAS PubMed.
- H. J. Snaith, Perovskites: the emergence of a new era for low-cost, high-efficiency solar cells, J. Phys. Chem. Lett., 2013, 4, 3623–3630 CrossRef CAS.
- J. H. Noh, S. H. Im, J. H. Heo, T. N. Mandal and S. I. Seok, Chemical management for colorful, efficient, and stable inorganic–organic hybrid nanostructured solar cells, Nano Lett., 2013, 13, 1764–1769 CrossRef CAS PubMed.
- F. Hao, C. C. Stoumpos, R. P. H. Chang and M. G. Kanatzidis, Anomalous band gap behavior in mixed Sn and Pb perovskites enables broadening of absorption spectrum in solar cells, J. Am. Chem. Soc., 2014, 136, 8094–8099 CrossRef CAS PubMed.
- G. E. Eperon, S. D. Stranks, C. Menelaou, M. B. Johnston, L. M. Herz and H. J. Snaith, Formamidinium lead trihalide: a broadly tunable perovskite for efficient planar heterojunction solar cells, Energy Environ. Sci., 2014, 7, 982–988 RSC.
- M. Hu, C. Bi, Y. Yuan, Y. Bai and J. Huang, Stabilized wide bandgap MAPbBrxI3−x perovskite by enhanced grain size and improved crystallinity, Adv. Sci., 2015, 3, 1500301 CrossRef PubMed.
- Y. Zhou, F. Wang, Y. Cao, J. Wang, H. Fang, M. A. Loi, N. Zhao and C.-P. Wong, Benzylamine-treated wide-bandgap perovskite with high thermal-photostability and photovoltaic performance, Adv. Energy Mater., 2017, 7, 1701048 CrossRef.
- Z. Yang, A. Rajagopal, S. B. Jo, C.-C. Chueh, S. Williams, C.-C. Huang, J. K. Katahara, H. W. Hillhouse and A. K.-Y. Jen, Stabilized wide bandgap perovskite solar cells by tin substitution, Nano Lett., 2016, 16, 7739–7747 CrossRef CAS PubMed.
- Y. Lin, B. Chen, F. Zhao, X. Zheng, Y. Deng, Y. Shao, Y. Fang, Y. Bai, C. Wang and J. Huang, Matching charge extraction contact for wide-bandgap perovskite solar cells, Adv. Mater., 2017, 29, 1700607 CrossRef PubMed.
- Y. Ogomi, A. Morita, S. Tsukamoto, T. Saitho, N. Fujikawa, Q. Shen, T. Toyoda, K. Yoshino, S. S. Pandey, T. Ma and S. Hayase, CH3NH3SnxPb(1–x)I3 perovskite solar cells covering up to 1060 nm, J. Phys. Chem. Lett., 2014, 5, 1004–1011 CrossRef CAS PubMed.
- C.-M. Tsai, H. Wu, S. Chang, C. Huang, C.-H. Wang, S. Narra, Y.-W. Yang, C.-L. Wang, C.-H. Hung and E. W.-G. Diau, Role of tin chloride in tin-rich mixed-halide perovskites applied as mesoscopic solar cells with a carbon counter electrode, ACS Energy Lett., 2016, 1, 1086–1093 CrossRef CAS.
- W. Liao, D. Zhao, Y. Yu, N. Shrestha, K. Ghimire, C. R. Grice, C. Wang, Y. Xiao, A. J. Cimaroli, R. J. Ellingson, N. J. Podraza, K. Zhu, R.-G. Xiong and Y. Yan, Fabrication of efficient low-bandgap perovskite solar cells by combining formamidinium tin iodide with methylammonium lead iodide, J. Am. Chem. Soc., 2016, 138, 12360–12363 CrossRef CAS PubMed.
- F. Zuo, S. T. Williams, P.-W. Liang, C.-C. Chueh, C.-Y. Liao and A. K.-Y. Jen, Binary-metal perovskites toward high-performance planar-heterojunction hybrid solar cells, Adv. Mater., 2014, 26, 6454–6460 CrossRef CAS PubMed.
- J. Liu, G. Wang, Z. Song, X. He, K. Luo, Q. Ye, C. Liao and J. Mei, FAPb1-xSnxI3 mixed metal halide perovskites with improved light harvesting and stability for efficient planar heterojunction solar cells, J. Mater. Chem. A, 2017, 5, 9097–9106 RSC.
- Z. Yang, A. Rajagopal, C.-C. Chueh, S. B. Jo, B. Liu, T. Zhao and A. K.-Y. Jen, Stable low-bandgap Pb-Sn binary perovskites for tandem solar cells, Adv. Mater., 2016, 28, 8990–8997 CrossRef CAS PubMed.
- X. Xu, C.-C. Chueh, Z. Yang, A. Rajagopal, J. Xu, S. B. Jo and A. K.-Y. Jen, Ascorbic acid as an effective antioxidant additive to enhance the efficiency and stability of Pb/Sn-based binary perovskite solar cells, Nano Energy, 2017, 34, 392–398 CrossRef CAS.
- Y. Li, W. Sun, W. Yan, S. Ye, H. Rao, H. Peng, Z. Zhao, Z. Bian, Z. Liu, H. Zhou and C. Huang, 50% Sn-based planar perovskite solar cell with power conversion efficiency up to 13.6%, Adv. Energy Mater., 2016, 6, 1601353 CrossRef.
- D. Zhao, Y. Yu, C. Wang, W. Liao, N. Shrestha, C. R. Grice, A. J. Cimaroli, L. Guan, R. J. Ellingson, K. Zhu, X. Zhao, R.-G. Xiong and Y. Yan, Low-bandgap mixed tin-lead iodide perovskite absorbers with long carrier lifetimes for all-perovskite tandem solar cells, Nat. Energy, 2017, 2, 17018 CrossRef CAS.
- G. Kapil, T. S. Ripolles, K. Hamada, Y. Ogomi, T. Bessho, T. Kinoshita, J. Chantana, K. Yoshino, Q. Shen, T. Toyoda, T. Minemoto, T. N. Murakami, H. Segawa and S. Hayase, Highly efficient 17.6% tin-lead mixed perovskite solar cells realized through spike structure, Nano Lett., 2018, 18, 3600–3607 CrossRef CAS PubMed.
- M. Liu, Z. Chen, Q. Xue, S. H. Cheung, S. K. So, H.-L. Yip and Y. Cao, High performance low-bandgap perovskite solar cells based on a high-quality mixed Sn–Pb perovskite film prepared by vacuum-assisted thermal annealing, J. Mater. Chem. A, 2018, 6, 16347–16354 RSC.
- Z. Chen, M. Liu, Z. Li, T. Shi, Y. Yang, H.-L. Yip and Y. Cao, Stable Sn/Pb-based perovskite solar cells with a coherent 2D/3D interface, iScience, 2018, 9, 337–346 CrossRef CAS PubMed.
- G. Xu, P. Bi, S. Wang, R. Xue, J. Zhang, H. Chen, W. Chen, X. Hao, Y. Li and Y. Li, Integrating ultrathin bulk-heterojunction organic semiconductor intermediary for high-performance low-bandgap perovskite solar cells with low energy loss, Adv. Funct. Mater., 2018, 28, 1804427 CrossRef.
- J. Yao, T. Kirchartz, M. S. Vezie, M. A. Faist, W. Gong, Z. He, H. Wu, J. Troughton, T. Watson, D. Bryant and J. Nelson, Quantifying losses in open-circuit voltage in solution-processable solar cells, Phys. Rev. Appl., 2015, 4, 014020 CrossRef.
- K. Kawashima, Y. Tamai, H. Ohkita, I. Osaka and K. Takimiya, High-efficiency polymer solar cells with small photon energy loss, Nat. Commun., 2015, 6, 10085 CrossRef CAS PubMed.
- W. Ke, G. Fang, Q. Liu, L. Xiong, P. Qin, H. Tao, J. Wang, H. Lei, B. Li, J. Wan, G. Yang and Y. Yan, Low-temperature solution-processed
tin oxide as an alternative electron transporting layer for efficient perovskite solar cells, J. Am. Chem. Soc., 2015, 137, 6730–6733 CrossRef CAS PubMed.
- J. P. C. Baena, L. Steier, W. Tress, M. Saliba, S. Neutzner, T. Matsui, F. Giordano, T. J. Jacobsson, A. R. S. Kandada, S. M. Zakeeruddin, A. Petrozza, A. Abate, M. K. Nazeeruddin, M. Grätzel and A. Hagfeldt, Highly efficient planar perovskite solar cells through band alignment engineering, Energy Environ. Sci., 2015, 8, 2928–2934 RSC.
- Q. Jiang, Z. Chu, P. Wang, X. Yang, H. Liu, Y. Wang, Z. Yin, J. Wu, X. Zhang and J. You, Planar-structure perovskite solar cells with efficiency beyond 21%, Adv. Mater., 2017, 29, 1703852 CrossRef PubMed.
- L. Guan, X. Yin, D. Zhao, C. Wang, Q. An, J. Yu, N. Shrestha, C. R. Grice, R. A. Awni, Y. Yu, Z. Song, J. Zhou, W. Meng, F. Zhang, R. J. Ellingson, J. Wang, W. Tang and Y. Yan, Cost-effective hole transporting material for stable and efficient perovskite solar cells with fill factors up to 82%, J. Mater. Chem. A, 2017, 5, 23319–23327 RSC.
- C. Wang, D. Zhao, C. R. Grice, W. Liao, Y. Yu, A. Cimaroli, N. Shrestha, P. J. Roland, J. Chen, Z. Yu, P. Liu, N. Cheng, R. J. Ellingson, X. Zhao and Y. Yan, Low-temperature plasma-enhanced atomic layer deposition of tin oxide electron selective layers for highly efficient planar perovskite solar cells, J. Mater. Chem. A, 2016, 4, 12080–12087 RSC.
- J. Song, E. Zheng, J. Bian, X. Wang, W. Tian, Y. Sanehira and T. Miyasaka, Low-temperature SnO2-based electron selective contact for efficient and stable perovskite solar cells, J. Mater. Chem. A, 2015, 3, 10837–10844 RSC.
- J. Song, E. Zheng, X. Wang, W. Tian and T. Miyasaka, Low-temperature-processed ZnO–SnO2 nanocomposite for efficient planar perovskite solar cells, Sol. Energy Mater. Sol. Cells, 2016, 144, 623–630 CrossRef CAS.
- Q. Jiang, X. Zhang and J. You, SnO2: a wonderful electron transport layer for perovskite solar cells, Small, 2018, 14, 1801154 CrossRef PubMed.
- L. Xiong, Y. Guo, J. Wen, H. Liu, G. Yang, P. Qin and G. Fang, Review on the application of SnO2 in perovskite solar cells, Adv. Funct. Mater., 2018, 28, 1802757 CrossRef.
- H.-L. Yip, S. K. Hau, N. S. Baek, H. Ma and A. K.-Y. Jen, Polymer solar cells that use self-assembled-monolayer modified ZnO/metals as cathodes, Adv. Mater., 2008, 20, 2376–2382 CrossRef CAS.
- L. Zuo, Q. Chen, N. D. Marco, Y.-T. Hsieh, H. Chen, P. Sun, S.-Y. Chang, H. Zhao, S. Dong and Y. Yang, Tailoring the interfacial chemical interaction for high-efficiency perovskite solar cells, Nano Lett., 2017, 17, 269–275 CrossRef CAS PubMed.
- J. Wei, F. Guo, X. Wang, K. Xu, M. Lei, Y. Liang, Y. Zhao and D. Xu, SnO2-in-polymer matrix for high-efficiency perovskite solar cells with improved reproducibility and stability, Adv. Mater., 2018, 1805153 CrossRef PubMed.
- A. Abrusci, S. D. Stranks, P. Docampo, H.-L. Yip, A. K.-Y. Jen and H. J. Snaith, High-performance perovskite-polymer hybrid solar cells via electronic coupling with fullerene monolayers, Nano Lett., 2013, 13, 3124–3128 CrossRef CAS PubMed.
- X. Liu, K.-W. Tsai, Z. Zhu, Y. Sun, C.-C. Chueh and A. K.-Y. Jen, A low-temperature, solution processable tin oxide electron-transporting layer prepared by the dual-fuel combustion method for efficient perovskite solar cells, Adv. Mater. Interfaces, 2016, 3, 1600122 CrossRef.
- C. Wang, C. Xiao, Y. Yu, D. Zhao, R. A. Awni, C. R. Grice, K. Ghimire, I. Constantinou, W. Liao, A. J. Cimaroli, P. Liu, J. Chen, N. J. Podraza, C.-S. Jiang, M. M. Al-Jassim, X. Zhao and Y. Yan, Understanding and eliminating hysteresis for highly efficient planar perovskite solar cells, Adv. Energy Mater., 2017, 7, 1700414 CrossRef.
- F. Brivio, A. B. Walker and A. Walsh, Structural and electronic properties of hybrid perovskites for high-efficiency thin-film photovoltaics from first-principles, APL Mater., 2013, 1, 042111 CrossRef.
- K. Hwang, Y.-S. Jung, Y.-J. Heo, F. H. Scholes, S. E. Watkins, J. Subbiah, D. J. Jones, D.-Y. Kim and D. Vak, Toward large scale roll-to-roll production of fully printed perovskite solar cells, Adv. Mater., 2015, 27, 1241–1247 CrossRef CAS PubMed.
- A. Rajagopal, Z. Yang, S. B. Jo, I. L. Braly, P.-W. Liang, H. W. Hillhouse and A. K.-Y. Jen, Highly efficient perovskite-perovskite tandem solar cells reaching 80% of the theoretical limit in photovoltage, Adv. Mater., 2017, 29, 1702140 CrossRef PubMed.
- Z. Chen, Z. Li, C. Zhang, X.-F. Jiang, D. Chen, Q. Xue, M. Liu, S. Su, H.-L. Yip and Y. Cao, Recombination dynamics study on nanostructured perovskite light-emitting devices, Adv. Mater., 2018, 30, 1801370 CrossRef PubMed.
- P. L. Qin, G. Yang, Z. W. Ren, S. H. Cheung, S. K. So, L. Chen, J. Hao, J. Hou and G. Li, Stable and efficient organo-metal halide hybrid perovskite solar cells via π-conjugated Lewis base polymer induced trap passivation and charge extraction, Adv. Mater., 2018, 30, 1706126 CrossRef PubMed.
- Q. Zeng, X. Zhang, X. Feng, S. Lu, Z. Chen, X. Yong, S. A. T. Redfern, H. Wei, H. Wang, H. Shen, W. Zhang, W. Zheng, H. Zhang, J. S. Tse and B. Yang, Polymer-passivated inorganic cesium lead mixed-halide perovskites for stable and efficient solar cells with high open-circuit voltage over 1.3 V, Adv. Mater., 2018, 30, 1705393 CrossRef PubMed.
- A. K. K. Kyaw, D. H. Wang, V. Gupta, W. L. Leong, L. Ke, G. C. Bazan and A. J. Heeger, Intensity dependence of current-voltage characteriztics and recombination in high-efficiency solution-processed small-molecule solar cells, ACS Nano, 2013, 7, 4569–4577 CrossRef CAS PubMed.
- D. Credgington, F. C. Jamieson, B. Walker, T.-Q. Nguyen and J. R. Durrant, Quantification of geminate and non-geminate recombination losses within a solution-processed small-molecule bulk heterojunction solar cell, Adv. Mater., 2012, 24, 2135 CrossRef CAS PubMed.
- V. Roiati, S. Colella, G. Lerario, L. De Marco, A. Rizzo, A. Listorti and G. Gigli, Investigating charge dynamics in halide perovskite sensitized mesostructured solar cells, Energy Environ. Sci., 2014, 7, 1889–1894 RSC.
- D. Bi, P. Gao, R. Scopelliti, E. Oveisi, J. Luo, M. Grätzel, A. Hagfeldt and M. K. Nazeeruddin, High-performance perovskite solar cells with enhanced environmental stability based on amphiphile-modified CH3NH3PbI3, Adv. Mater., 2016, 28, 2910–2915 CrossRef CAS PubMed.
Footnotes |
† Electronic supplementary information (ESI) available. See DOI: 10.1039/c8qm00620b |
‡ These authors contributed equally to this work. |
|
This journal is © the Partner Organisations 2019 |