DOI:
10.1039/C8QI01055B
(Research Article)
Inorg. Chem. Front., 2019,
6, 184-191
MOFs containing a linear bis-pyridyl-tris-amide and angular carboxylates: exploration of proton conductivity, water vapor and dye sorptions†
Received
30th September 2018
, Accepted 9th November 2018
First published on 9th November 2018
Abstract
Exploration of a new type of water-stable proton conducting material is of great interest owing to its applications as an electrolytic material in proton exchange membrane fuel cells (PEMFC). Herein, we report four such new metal organic frameworks of Cd(II) and Co(II) containing a new linear bis-pyridyl-tris-amide ligand (L) and angular dicarboxylates 4,4′-oxybisbenzoic acid (OBA) and benzene-1,3-diacrylic acid (BDA) as linkers. The MOFs are found to exhibit a general formula of {[M(L)(dicarboxylate)]·xDMF·yH2O}n with varying amounts of solvent. Two MOFs containing BDA exhibited two-dimensional rectangular grid-like networks with grid dimensions of 25 × 23 Å2, while those with OBA exhibited 3D-networks consisting of the mab topology with rectangular channels of dimensions 31 × 21 Å2 and 31 × 17 Å2. All four exhibited a solvent accessible volume of 36–49%. AC impedance analyses on MOFs 1–4 reveal that they exhibit appreciable proton conductivities (PC): σ = 2.2 × 10−3, 9.5 × 10−4, 1.2 × 10−3 and 6.6 × 10−4 S cm−1, respectively, at 353 K and 98% relative humidity (RH). The high PC values could be attributed to the hydrogen bonding between water molecules and also with amides and carboxylates of the framework. Furthermore, a gas sorption study revealed that MOFs 1–4 show preferential adsorption of water vapor (195–330 cc g−1 at p/p0 ∼ 1) over nitrogen given the hydrophilic nature of the channels and the stability of MOFs under humid conditions. In addition, all these materials have shown capability in selectively adsorbing cationic dyes (methylene blue, MB and crystal violet, CV).
Introduction
The design and synthesis of novel electrolyte materials for proton exchange membrane fuel cells are of immense interest given their applications in various fields.1–5 One of the most popular materials that is being used as an electrolyte membrane is hydrated Nafion. This material was known to exhibit the highest proton conductivity of the order 1 × 10−1 S cm−1 at temperatures of 60–80 °C and 98% relative humidity (RH). However, this material is expensive, preventing large scale commercial applications, and also it is prone to dehydration which results in a drop of its proton conductivity at elevated temperatures.6–8 Therefore, it is necessary to develop low cost novel electrolyte crystalline solid materials exhibiting efficient proton conduction under ambient conditions. In this context, metal organic frameworks (MOFs)9–18 or porous coordination polymers (PCPs)19–25 and covalent organic frameworks (COFs)26–29 are being considered as an alternative class of materials given their structural diversity, tunability and porosity. The crystalline nature of these materials provides a precise understanding of their structural features that can be utilized for deducing proton conduction pathways which in turn offers vital clues for designing new efficient proton conductors.30–39
In the recent literature, several MOFs have been designed and explored for their proton conducting abilities. In particular, the introduction of proton conducting functional groups, such as –OH, –COOH, –SO3H, and –PO3H, and proton carriers, such as imidazole, triazole, benzylimidazole and ammonium cations, on to frameworks has been explored extensively.40–46 For instance, Kitagawa and coworkers have shown that MOFs (MIL-53) containing imidazole or histamine moieties exhibit proton conductivities of 2.2 × 10−5 S cm−1 at 120 °C and 1.7 × 10−3 S cm−1 at 150 °C, respectively, under anhydrous conditions.47 Hupp and coworkers studied the effect of included solvent molecules such as H2O, MeOH, EtOH or MeCN on proton conduction in HKUST-1 which varies in the range of 10−5 to 10−7 S cm−1.48 Furthermore, Ponomareva and Fedin and their coworkers impregnated a chromium-based MOF (Cr-bdc-MIL-101) with strong acids (H2SO4, H3PO4, CF3SO3H, or p-CH3C6H5SO3H) or an acidic salt (CsHSO4) and have shown that its proton conductivity can be varied in the range of 10−7 to 10−2 S cm−1 at 150 °C depending on the concentration and nature of the doped acids.34 Recently, we have reported the proton conductivity of a 3D-coordination polymer in which the proton conduction arises from the included one-dimensional water cages. The infinite hydrogen bonded water cages promoted the proton transfer via the Grotthuss-type proton-hopping mechanism.49
On the other hand, design of materials for water adsorption is of high importance owing to their utility in the purification of biofuels, the electro-catalytic oxidation of alcohols in fuel cells, and in the design of cooling devices and heat pumps.50–54 Several MOFs containing 3D- or 2D-porous networks have been explored for their water sorption ability to date.55–61 However, it is a challenge to design water stable porous MOFs as several of them are sensitive to water.62,63 We have recently reported some water stable and water vapor adsorbing porous MOFs which contain hydrophilic functional groups such as imidazole or amides in their organic struts.64 To date, some MOFs have been shown to exhibit proton conductivity and few have been shown to exhibit water sorption capability. However, to the best of our knowledge, few CPs have been reported exhibiting the combination of both the properties.65–67 The CPs which exhibit both the properties can serve as excellent candidates in the design of fuel cells.
So far, bis-pyridyl-mono-amides or bis-pyridyl-bis-amides containing aryl or alkyl spacers have been shown to form PCPs/MOFs with the ability to encapsulate various water clusters in their hydrophilic channels.49,68 Nevertheless, no CPs/MOFs have been reported with a linear bis-pyridyl-tris-amide to date, and the additional amide group in L is expected to increase the hydrophilic nature of the MOFs. Considering the above facts and in search of better performing porous materials for both water and proton conduction, in this manuscript, a new bis-pyridyl linear tri-amide (L, Scheme 1) has been designed in order to synthesize MOFs containing hydrophilic channels to explore vapor sorption and proton conductivity. In addition to the amide containing ligand, two types of angular dicarboxylates (OBA and BDA) have been utilized to synthesize MOFs of Cd(II) and Co(II). As anticipated, these MOFs are found to exhibit appreciable amounts of water encapsulation and vapor sorption abilities. Furthermore, all the four MOFs studied here are found to exhibit significant proton conducting properties. Moreover, the MOFs have shown the ability for cationic dye (MB and CV) sorption from aqueous solution.
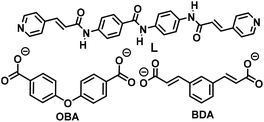 |
| Scheme 1 Structural drawing for L, OBA and BDA. | |
Results and discussion
The ligand L was synthesized by the condensation reaction of 1,4-diaminobenzanilide and 4-pyridylacrylic acid in the presence of pyridine and triphenyl phosphite.69,70 The complexation reactions of ligand L and angular dicarboxylic acids (OBA and BDA) with Cd(II) and Co(II) are carried out in DMF, MeOH and H2O under solvothermal conditions.
Single crystals suitable for X-ray diffraction are obtained for their respective MOFs {[Cd(L)(BDA)]·(DMF)·16H2O}n, 1, {[Co(L)(BDA)]·(DMF)·13H2O}n, 2, {[Cd(L)(OBA)]·(2DMF)·8H2O}n, 3, and {[Co(L)(OBA)]·(2DMF)·6H2O}n, 4. Pertinent crystallographic details of MOFs 1–4 are shown in Table 1. Single crystal analysis revealed that MOFs 1 and 2 are iso-structural and crystallized in the P
space group while MOFs 3 and 4 crystallized in P21/n and have a network isostructurality with huge differences in cell parameters. The MOFs 1 and 2 are found to exhibit two-dimensional networks containing rectangular grids whereas 3 and 4 exhibit three-dimensional networks with the mab-topology containing channels.
Table 1 Crystallographic parameters for MOFs 1–4
Compound |
MOF-1 |
MOF-2 |
MOF-3 |
MOF-4 |
Formula |
C44H79CdN6O24 |
C44H73CoN6O21 |
C49H60CdN7O18 |
C49H59CoN7O14 |
M. wt |
1123.16 |
1048.11 |
1082.44 |
996 |
T (K) |
293 K |
293 K |
293 K |
293 K |
System |
Triclinic |
Triclinic |
Monoclinic |
Monoclinic |
Space group |
P![[1 with combining macron]](https://www.rsc.org/images/entities/char_0031_0304.gif) |
P![[1 with combining macron]](https://www.rsc.org/images/entities/char_0031_0304.gif) |
P21/n |
P21/n |
a (Å) |
10.029(6) |
9.774(7) |
24.42(2) |
23.803(2) |
b (Å) |
13.435(7) |
13.183(10) |
9.548(8) |
9.7214(10) |
c (Å) |
22.070(13) |
22.520(12) |
28.679(18) |
23.932(2) |
α (°) |
104.66(2) |
103.44(3) |
90 |
90 |
β (°) |
96.42(3) |
95.59(2) |
111.44(7) |
103.862(4) |
γ (°) |
95.68(3) |
96.68(3) |
90 |
90 |
Vol. (Å3) |
2834(3) |
2780(3) |
6224(8) |
5376.5(8) |
Z
|
2 |
2 |
4 |
4 |
D (mg m−3) |
1.2799(14) |
0.9469(10) |
1.0952(14) |
0.9941(1) |
R
1 [I > 2σ(I)] |
0.0813 |
0.0647 |
0.0765 |
0.0726 |
wR2 (on F2, all data) |
0.2176 |
0.1712 |
0.2065 |
0.2201 |
The asymmetric units of MOFs 1 and 2 contain one unit each of the corresponding metal ion, ligand, BDA and DMF and several water molecules. In both the structures, the metal exhibits a pentagonal bipyramidal geometry in which the metal is ligated by five O-atoms from carboxylates of BDA in the equatorial positions (Cd–O: 2.246(1), 2.352(4), 2.246(1), 2.487(4), 2.722(5) Å) and the axial positions are occupied by two N-atoms of the two different ligands (Cd–N: 2.319(4), 2.306(5) Å) (Fig. 1a). The carboxylates form M2(COO)2 Secondary Building Units (SBUs) which are linked further to form a double chain through BDA moieties (Fig. 1b). These double chains are connected by linear ligand L to form a 2D-rectangular grid network (Fig. 1c) such that the double chains of M(BDA) within the layer are separated from each other by a distance of 31.66 Å. In both the structures, L exhibits near planarity and the middle amide group points in an opposite direction to terminal amide groups. The rectangular grids are double walled either by dicarboxylate or L moieties. The L units form a dimer via π–π interactions with an interlayer separation of 3.75 Å (Fig. 1d). Within the dimer, the double bonds are found to have a crisscross alignment making the MOFs photo-stable. The 2D rectangular grids are further stacked on top of each other via N–H⋯O (carboxylate) and C–H⋯O (amide) hydrogen bonding interactions such that there exist continuous channels across the layers. The lattice solvent water and DMF molecules form aggregates in the channels across 2D-layers via O−H⋯O hydrogen bonding (Fig. 1e). The aggregates of solvents are hydrogen bonded further to the network with amide and carboxylate moieties. Both the structures contain rectangular grid type cavities of dimensions 25 × 23 Å2 and 25 × 22 Å2 in MOFs 1 and 2, respectively. PLATON calculations reveal that MOFs 1 and 2 contain 45% and 42% solvent accessible voids, respectively.
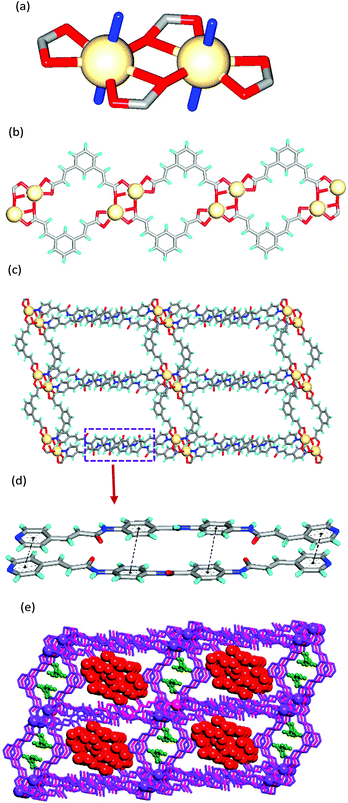 |
| Fig. 1 Illustrations of the crystal structure of MOF-1: (a) coordination environment around the Cd(II) ion and bimetallic SBU; (b) 1D-double chain via M2(CO2)2 SBUs; (c) double-walled 2D-layer containing rectangular grids; (d) π–π interactions between two L units and crisscross alignment of double bonds; (e) rectangular channels across the stacking of 2D layers: arc like channels occupied by DMF (green) and rectangular channels (space filling mode) by water molecules. | |
MOFs 3 and 4 crystallized in the P21/n space group with significant differences in cell parameters, in particular, along the z-axis. The asymmetric units possess one unit each of the metal ion (Cd(II) for 3 and Co(II) for 4), ligand, OBA and uncoordinated water molecules (eight in 3 and six in 4) and two free DMF molecules. In both the structures, the metal ion adopts a pentagonal bipyramidal geometry where the metal is ligated by five O-atoms from the carboxylates of OBA in the equatorial positions (Cd–O: 2.392(5), 2.399(4), 2.647(5), 2.331(5), and 2.260(4) Å) and the axial positions are occupied by two N-atoms of the two different ligands (Cd–N: 2.281(5) and 2.296(5) Å) (Fig. 2a) similar to that observed in MOFs 1 and 2. Furthermore, the metal ions and carboxylates are found to form similar SBUs of M2(COO)2 (Cd–Cd: 3.902(2) Å in 3 and Co–Co: 4.0354(8) Å in 4). Interestingly, these SBUs are interconnected by OBA moieties such that they form a corrugated 2D-network containing arc-shaped channels that are occupied by DMF molecules (Fig. 2b). These 2D corrugated layers are pillared by ligand L to form a 3D-network (Fig. 2c). The consideration of ligands and linkers as spacers and the Cd2 unit as a six-connected node (Fig. S2a†) resulted in the 3D-network with the mab topology (Fig. S2b†). The 3D-network contains rectangular grid-type channels of dimensions 31 × 21 Å2 for 3 and 31 × 17 Å2 for 4. The pillaring of the layers occurred through the z-axis that represents the interlayer separation which is somewhat higher for 3 (21.64 Å) than 4 (18.790 Å). As a result, the overall volume of 3 is observed to be higher than that of 4 by 848 Å3. PLATON calculations reveal that the crystal lattices of 3 and 4 contain 49% and 39% of guest accessible volume, respectively. Within the 3D-network, the ligand molecules are found to form infinite dimers via π–π interactions with centroid to centroid distances of pyridine moieties of about 3.7 Å. In these stacks, the double bonds are aligned in a crisscross manner with –C
C– distances of 3.757 Å and 4.067 Å making the MOFs photo-stable (Fig. 2d).
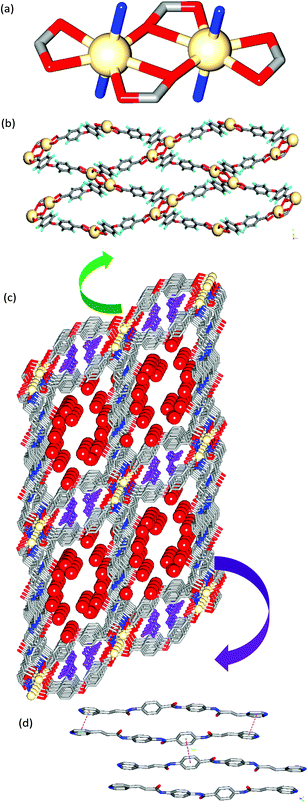 |
| Fig. 2 Illustrations of the crystal structure of MOF-3: (a) coordination environment around the Cd(II) ion; (b) 2D-corrugated layer via M2(CO2)2 SBUs; (c) 3D-network formed by the pillaring of metal carboxylate 2D layers by L units; notice two types of channels occupied by DMF and water molecules; and (d) π–π interactions between two L units and the crisscross alignment of double bonds. | |
The phase purities of the bulk materials were confirmed by the PXRD measurements, which are in good agreement with the simulated PXRD patterns. The thermal, water and chemical stabilities of the porous materials are of importance for exploring their practical applications, in particular, proton conductivity and vapor sorption. Interestingly, all the MOFs are found to be air stable and retain their crystallinity under ambient conditions for several days. Furthermore, the thermal and chemical stabilities of all the MOFs were investigated. Thermogravimetric analysis (TGA) shows that all the MOFs were stable up to 330 °C; the as-synthesized samples lose the water molecules in a temperature range of 50–110 °C, with further weight loss observed up to 330 °C corresponding to DMF molecules and above which the decomposition of the host framework is observed (Fig. S12†). The structural integrity of all the MOFs against common organic solvents such as water and methanol is also explored by suspending the fresh crystalline samples in these solvents for one month. The PXRD patterns clearly confirm the structural stability of the MOFs (Fig. S25–S28†) under these conditions.
Proton conducting abilities of MOFs 1–4
The presence of several water molecules in the lattice and their clustering via hydrogen bonding in the framework prompted us to investigate the proton conductivities (PC) of these four MOFs. The proton conductivities of the as-synthesized materials were evaluated by conductive alternating current (ac) impedance on a pressed pellet of the powder samples via the quasi-two-probe method and the PC values of MOFs 1–4 are 4.5 × 10−4, 9.7 × 10−6, 4.7 × 10−5 and 4.6 × 10−6 S cm−1, respectively, at 353 K and 50% RH. The Nyquist plots for all MOFs are depicted in Fig. 3. However, with increasing humidity, the PC values of each sample are found to increase and attain maximum values of 2.2 × 10−3, 9.5 × 10−4, 1.2 × 10−3 and 6.6 × 10−4 S cm−1 at 353 K and 98% RH, under different relative humidity (%RH) conditions, which clearly indicate the role of water molecules as proton carriers. These observed PC values are more effective than those of the recently reported Mg-MOF (MIT-25)71 and Zn-MOF encapsulated with water chains.72 Furthermore, the temperature dependent PC study of all the MOFs was carried out and it was observed that at 293 K, MOF-1 exhibits the lowest PC value (6.2 × 10−6 S cm−1) and further reaches the maximum (2.2 × 10−3 S cm−1) with the increase in temperature up to 353 K (Fig. S14†). Similar behaviour is also observed in the case of MOFs 2, 3 and 4 (Fig. ESI†).
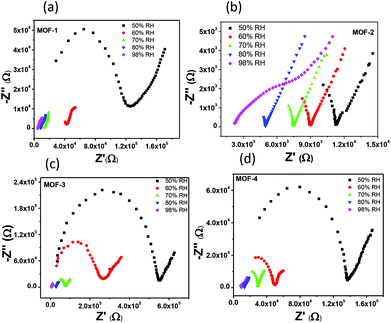 |
| Fig. 3 (a)–(d) Nyquist plots for MOFs 1–4, respectively, at 80 °C and under different relative humidity (%RH) conditions. | |
Vapour sorption abilities of MOFs 1–4
To examine their porosity, N2 sorption studies of all the materials were carried out at 298 K. Prior to these studies, the as-synthesized samples were soaked in acetone and acetonitrile in order to exchange water and DMF with the volatile solvent. These volatile solvents were evacuated from the channels by heating the materials at 120 °C under vacuum for 12 h. All the MOFs were found to show low uptake values for N2 at low pressure. They adsorbed 150–380 cc g−1 at high pressure with the type III sorption profile indicating their low sorption affinity towards N2 (Fig. S13†). In general, MOFs containing polar groups such as imidazoles or amides can act as primary adsorbing centers for water due to their hydrophilic nature. MOFs presented here contain amides and carboxylates which interact with water vapor and alcohols; moreover, the thermal and chemical stabilities of all the MOFs prompted us to carry out water and MeOH sorption studies. Interestingly, all the materials 1–4 have shown greater ability to adsorb water with some differences associated with their solvent accessible voids. All the MOFs exhibited the type II adsorption profile (Fig. 4) and the amounts of water adsorbed in MOFs 1–4 up to p/p0 ∼ 0–0.9 are 254, 195, 330 and 180 cc g−1, respectively, and the corresponding surface areas of 1–4 are 792, 658, 905 and 753 m2 g−1, respectively. Interestingly, the PXRD patterns of the MOFs 1–4 confirm the crystallinity and integrity of the frameworks after vapour sorption (Fig. S21–S24†). The sorption amounts and surface areas are better than those of some of the recently reported MOFs (ESI, Table 2†). The amount of MeOH adsorbed by MOFs 1–4 is 207, 190, 225 and 175 cc g−1, respectively. The MOFs 1–4 were found to show greater uptake of water compared to methanol given their molecular size differences. The hysteresis nature in the desorption profiles could be due to the strong interactions between the frameworks and adsorbed solvent molecules within the crystal lattice.
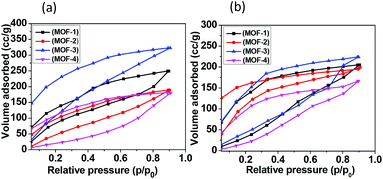 |
| Fig. 4 Adsorption and desorption isotherms for MOFs 1–4 at 273 K and one bar pressure: (a) water vapor sorption; and (b) methanol vapour sorption. | |
Dye adsorption by MOFs 1–4
Organic dyes are widely used in many industrial applications such as paper, textiles, printing, leather, and fertilizers. These dyes are stable at room temperature and under UV and are non-biodegradable. All the dyes are toxic and cause severe problems to aquatic life and may be damaging to human life.73 Thus, removing dyes from waste water quickly and economically remains a great challenge for researchers. The adsorption by porous materials is an efficient, cost-effective and simple process. To date, several CPs have been explored for the removal of dyes from waste water and have been shown to exhibit preferential adsorption depending on the nature of the framework. Cationic frameworks preferentially adsorb anionic dyes and vice versa.49,74 On the other hand, very few examples have been reported for such selective sorption with neutral CPs/MOFs.56,75 The presence of large channels with several solvent molecules, and the water stability of these MOFs prompted us to study dye removal from aqueous solutions. Therefore, the cationic (methylene blue, MB) and anionic dye (methyl orange, MO) sorptions by all the four MOFs were investigated. The aqueous dye (MB or MO) solutions are prepared with 10−5 molar concentrations, and a typical dye adsorption experiment was carried out by immersing 10 mg of the freshly prepared MOFs 1–4 in the respective dye solutions. These solutions are monitored by UV-vis spectrometry at different time intervals (Fig. 5). From the UV spectra, the decrease of the concentration of MB dye can be seen with time as the materials adsorb the dye from aqueous solutions and a change in the color of the material was observed (Fig. S16†). However, no such change in the concentration was observed for solutions of MO (Fig. S17†) indicating the zero affinity of MOFs towards the anionic dye sorption. The MOFs 1 and 3 adsorbed all of the MB in 30 min from the solution, whereas, MOFs 2 and 4 were found to adsorb in 60 min. MOFs 1 and 3 adsorbed MB at a faster rate compared to MOFs 2 and 4 given their more solvent occupied volumes in the crystal lattice. The size selectivity of the MOFs 1–4 towards cationic dyes was further verified by considering another set of cationic dyes such as crystal violet (CV) and rhodamine B (RB). MOFs 1–4 are found to adsorb CV at a lower rate compared to MB (Fig. S18†). However, MOFs 1–4 are found to show zero propensity towards RB (Fig. S19†). The probable reason for the selective adsorption of cationic dyes by MOFs 1–4 over anionic dyes could be the presence of carboxylates and hydrophilic functional groups on the framework which can form better interactions with MB.
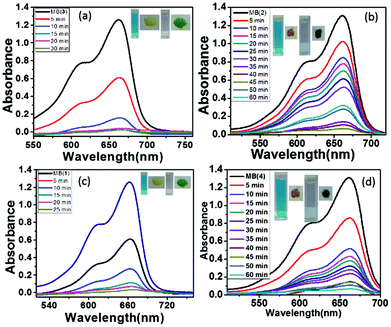 |
| Fig. 5 (a)–(d) UV-vis spectra for the uptake of methylene blue from aqueous solutions at various time intervals for MOFs 1–4, respectively. | |
Conclusions
In summary, for the first time, we have synthesized a linear bis-pyridyl tris-amide (L). Ligand L in combination with angular di-carboxylates such as benzene-1,3-diacrylic acid and 4,4′-oxybisbenzoic acid was shown to form four new MOFs of Cd(II) and Co(II). The dicarboxylate BDA was shown to have a propensity to form a 1D double chain through M2(BDA)2 SBUs. Furthermore, these 1D double chains are connected by L to form 2D-rectangular grid-type networks containing large cavities whereas, dicarboxylate OBA has shown the tendency to form 2D-corrugated layers via M2(COO)2 SBUs. These layers are further interconnected by L to form a 3D-network with the mab topology. All the four MOFs contain a plethora of solvent molecules in their channels. The presence of hydrogen bonded water molecules resulted in appreciable proton conductivities σ = 2.2 × 10−3, 9.5 × 10−4, 1.2 × 10−3 and 6.6 × 10−4 S cm−1 for MOFs 1–4, respectively, at 353 K and 98% relative humidity (RH). The hydrophilic nature of the channels resulted in the selective adsorption of water vapor over nitrogen. Furthermore, all the MOFs have shown the tendency to selectively adsorb cationic dyes such as methylene blue and crystal violet.
The present work opens up new opportunities to design and synthesize multifunctional materials which can exhibit proton conduction, vapor sorption, selective dye adsorption and other practical applications.
Experimental section
All the chemicals, such as pyridine, 4-pyridinecarboxaldehyde, piperidine, triphenyl phosphite, 4,4′-oxybisbenzoic acid, N,N′-dimethylformamide and metal salts, were purchased from local chemical suppliers, and 4,4′-diamino benzanilide was purchased from Sigma Aldrich and used without purification. NMR spectra were recorded on a Bruker DRX400 spectrometer. IR spectra were recorded on a PerkinElmer Instrument Spectrum Rx Serial No. 73713. Melting points were measured using a Fisher Scientific melting point apparatus, cat. no. 12-144-1. The X-ray powder diffraction (XRPD) data were recorded with a Bruker D8-Advance diffractometer at room temperature. Thermogravimetric analysis (TGA) data were recorded under a nitrogen atmosphere at a heating rate of 5 °C min−1 with a PerkinElmer instrument, Pyris Diamond TG/DTA. The UV spectra with dye adsorption measurements were recorded with the use of a Shimadzu (model no. UV2450) UV-vis spectrophotometer. The gas and vapor sorption experiments were performed using a Quantachrome autosorb iQ automated gas sorption analyzer. The impedance studies were conducted by electrochemical impedance spectroscopy (EIS) using Gamry Instruments, reference 3000 galvanostat between frequency ranges of 100 Hz and 1 MHz. The equivalent circuit model diagram for the impedance measurement of MOFs 1–4 is depicted in Fig. S15† which indicates the single phase of the materials.
Synthesis of L
In a round bottom flask, 4-pyridine acrylic acid (1.49 g, 0.01 mol) and 4,4′-diaminobenzanilide (1.134 g, 0.005 mol) were taken in pyridine (10 mL) and stirred for about 15–20 minutes. To this mixture, triphenyl phosphite (3.163 mL, 0.01 mol) was added and refluxed for 12 hours, the mixture was allowed to cool to room temperature, excess pyridine was washed with distilled water and acetone dried under vacuum and the light orange solid was recrystallized from ethanol: DMF mixture, yield: 70%, and further characterized by 1H NMR.
Syntheses of MOFs 1 and 2
A mixture of L (12.25 mg, 0.025 mmol) and benzene-1,3-diacrylic acid (5.4 mg) (0.025 mmol) was dissolved in 4 mL of DMF and MeOH (1
:
1) in a glass tube. To this, 2 mL of the corresponding aqueous solution of metal salts (Cd(NO3)2·4H2O, 7.71 mg, 0.025 mmol for 1 and Co(NO3)2·4H2O, 7.27 mg, 0.025 mmol) was added. The glass tube was sealed and heated at 100 °C for two days and then slowly cooled down to room temperature which resulted in the single crystals of MOFs. The obtained solids are washed with DMF and water several times and dried in air. The yields of MOFs 1 and 2 were calculated to be 60 and 45%, respectively.
Elemental analysis of MOF-1.
Calcd for C44H79CdN6O24 (%): C, 47.01; H, 7.03; N, 7.47. Obsd (%): C, 48.20; H, 6.85, N, 8.40.
MOF-2.
Calcd for C44H73CoN6O21 (%): C, 50.38; H, 6.96; N, 8.01. Obsd (%): C, 51.80; H, 6.11; N, 9.54.
Syntheses of MOFs 3 and 4
A similar procedure was adopted for the synthesis of MOFs 3 and 4 by taking OBA (6.4 mg) (0.025 mmol) as a coligand. Light yellow and light orange crystals of MOFs 3 and 4, respectively, were obtained in two days in 50 and 55% yields, respectively.
Elemental analysis of MOF-3.
Calcd for C49H60CdN7O18 (%): C, 54.34; H, 5.5; N, 9.05. Obsd (%): C, 52.98; H, 5.69; N, 10.03.
MOF-4.
Calcd for C49H59CoN6O14 (%): C, 59.03; H, 5.92; N, 9.83. Obsd (%): C, 57.80; H, 4.96; N, 8.93.
Crystal structure determination by single crystal X-ray diffraction
All of the single crystal data were collected on a Bruker-APEX-II CCD X-ray diffractometer that uses graphite monochromated Mo Kα radiation (γ = 0.71073 Å) at a temperature of 150 K by the hemisphere method. The structures were solved by direct methods and refined by least-squares methods on F2 using SHELX-2014.76 Non-hydrogen atoms were refined anisotropically, and hydrogen atoms were fixed at calculated positions and refined using a riding model. The H atoms attached to the O atom or N atoms are located wherever possible and refined using the riding model. Some of the solvent molecules (DMF and H2O) are located which are found to exhibit high thermal motion in subsequent refinements. Therefore, final refinements for all MOFs were carried out by removing all solvent molecules and using the Platon squeeze option.77 The number of solvent molecules in the formulae of MOFs was deduced using the combination of the initial location of solvent molecules and TGA analysis.
Conflicts of interest
There are no conflicts to declare.
Acknowledgements
We acknowledge DST (SERB) (SR/S1/IC-08/2013), New Delhi, India, for financial support and DST-FIST for the single crystal X-ray diffractometer. S. K. K. acknowledges UGC and IIT Kharagpur for research fellowship.
References
-
P. Colomban, Proton Conductors: Solids, Membranes and Gels-Materials and Devices. Series: Chemistry of Solid State Materials, Cambridge University Press, Cambridge, UK, 1992 Search PubMed
.
- J. M. Taylor, K. W. Dawson and G. K. H. Shimizu, J. Am. Chem. Soc., 2013, 135, 1193 CrossRef CAS PubMed
.
- M. Yoon, K. Suh, S. Natarajan and K. Kim, Angew. Chem., Int. Ed., 2013, 52, 2688 CrossRef CAS PubMed
.
- K.-D. Kreuer, S. J. Paddison, E. Spohr and M. Schuster, Chem. Rev., 2004, 104, 4637 CrossRef CAS PubMed
.
- M. Yoon, K. Suh, H. Kim, Y. Kim, N. Selvapalam and K. Kim, Angew. Chem., Int. Ed., 2011, 50, 7870 CrossRef CAS PubMed
.
- K. A. Mauritz and R. B. Moore, Chem. Rev., 2004, 104, 4535 CrossRef CAS PubMed
.
- S. S. Ivanchev, Russ. J. Appl. Chem., 2008, 81, 569 CrossRef CAS
.
- S. J. Paddison, Annu. Rev. Mater. Res., 2003, 33, 289 CrossRef CAS
.
- Z. J. Lin, J. Lu, M. C. Hong and R. Cao, Chem. Soc. Rev., 2014, 43, 5867 RSC
.
- P. Ramaswamy, N. E. Wong and G. K. H. Shimizu, Chem. Soc. Rev., 2014, 43, 5913 RSC
.
- J. Y. Xiong, Y.-Z. Fan, R. Yang, S. Chen, M. Pan, J.-J. Jiang and C.-Y. Su, Chem. Commun., 2014, 50, 14631 RSC
.
- A. Schoedel, M. Li, D. Li, M. ÖKeeffe and O. M. Yaghi, Chem. Rev., 2016, 116, 12466 CrossRef CAS PubMed
.
- T. L. Easun, F. Moreau, Y. Yan, S. Yang and M. Schroder, Chem. Soc. Rev., 2017, 46, 239 RSC
.
- T. Kitao, Y. Zhang, S. Kitagawa, B. Wang and T. Uemura, Chem. Soc. Rev., 2017, 46, 3108 RSC
.
- S. Wang, M. Chen, Y. Xie, Y. Fan, D. Wang, J.-J. Jiang, Y. Li, H. Grützmacher and C.-Y. Su, Small, 2016, 12, 2365 CrossRef CAS PubMed
.
- W. P. Lustig, S. Mukherjee, N. D. Rudd, A. D. Desai, J. Li and S. K. Ghosh, Chem. Soc. Rev., 2017, 46, 3242 RSC
.
- K. Adil, Y. Belmabkhout, R. S. Pillai, A. Cadiau, P. M. Bhatt, A. H. Assen, G. Maurin and M. Eddaoudi, Chem. Soc. Rev., 2017, 46, 3402 RSC
.
- H. Aggarwal, R. K. Das, P. M. Bhatt and L. J. Barbour, Chem. Sci., 2015, 6, 4986 RSC
.
- C. R. Kim, T. Uemura and S. Kitagawa, Chem. Soc. Rev., 2016, 45, 3828 RSC
.
- B. Zheng, J. Bai, J. Duan, L. Wojtas and M. J. Zaworotko, J. Am. Chem. Soc., 2011, 133, 748 CrossRef CAS PubMed
.
- A.-L. Li, Q. Gao, J. Xu and X.-H. Bu, Coord. Chem. Rev., 2017, 344, 54 CrossRef CAS
.
- G. Kumar and R. Gupta, Chem. Soc. Rev., 2013, 42, 9403 RSC
.
- S. Kitagawa, R. Kitaura and S. Noro, Angew. Chem., Int. Ed., 2004, 43, 2334 CrossRef CAS PubMed
.
- T. Yamada, K. Otsubo, R. Makiura and H. Kitagawa, Chem. Soc. Rev., 2013, 42, 6655 RSC
.
- W. Lin, W. J. Rieter and K. M. L. Taylor, Angew. Chem., Int. Ed., 2009, 48, 650 CrossRef CAS PubMed
.
- S. Chandra, T. Kundu, S. Kandambeth, R. Babarao, Y. Marathe, K. S. Kunjir and R. Banerjee, J. Am. Chem. Soc., 2014, 136, 6570 CrossRef CAS PubMed
.
- Y. X. Ye, L. Q. Zhang, Q. F. Peng, G. E. Wang, Y. C. Shen, Z. Y. Li, L. H. Wang, L. Ma, Q. H. Chen, Z. J. Zhang and S. Xiang, J. Am. Chem. Soc., 2015, 137, 913 CrossRef CAS PubMed
.
- Y. Peng, G. Xu, Z. Hu, Y. Cheng, C. Chi, D. Yuan, H. Cheng and D. Zhao, ACS Appl. Mater. Interfaces, 2016, 8, 18505 CrossRef CAS PubMed
.
- H. S. Sasmal, H. B. Aiyappa, S. N. Bhange, S. Karak, A. Halder, S. Kurungot and R. Banerjee, Angew. Chem., Int. Ed., 2018, 57, 10894–10898 CrossRef CAS PubMed
.
- H. Li, M. Eddaoudi, M. ŎKeeffe and O. M. Yaghi, Nature, 1999, 402, 276 CrossRef CAS
.
- A. Schneemann, V. Bon, I. Schwedler, I. Senkovska, S. Kaskel and R. A. Fischer, Chem. Soc. Rev., 2014, 43, 6062 RSC
.
- S. Horike, D. Umeyama and S. Kitagawa, Acc. Chem. Res., 2013, 46, 2376 CrossRef CAS PubMed
.
- H. Aggarwal, P. M. Bhatt, C. X. Bezuidenhout and L. J. Barbour, J. Am. Chem. Soc., 2014, 136, 3776 CrossRef CAS PubMed
.
- V. G. Ponomareva, K. A. Kovalenko, A. P. Chupakhin, D. N. Dybtsev, E. S. Shutova and V. P. Fedin, J. Am. Chem. Soc., 2012, 134, 15640 CrossRef CAS PubMed
.
- J. Zhang, L. Chen, D. Gui, H. Zhang, D. Zhang, W. Liu, G. Huang, J. Diwu, Z. Chaib and S. Wang, Dalton Trans., 2018, 47, 5161 RSC
.
- S. Biswas, J. Chakraborty, V. S. Parmar, S. P. Bera, N. Ganguli and S. Konar, Inorg. Chem., 2017, 56, 4956 CrossRef CAS PubMed
.
- Y.-H. Han, Y. X. Ye, C. Tian, Z. Zhang, S.-W. Du and S. C. Xiang, J. Mater. Chem. A, 2016, 4, 18742 RSC
.
- W. J. Phang, H. Jo, W. R. Lee, J. H. Song, K. Yoo, B. Kim and C. S. Hong, Angew. Chem., Int. Ed., 2015, 54, 5142 CrossRef CAS PubMed
.
- S. R. Kim, K. W. Dawson, B. S. Gelfand, J. M. Taylor and G. K. H. Shimizu, J. Am. Chem. Soc., 2013, 135, 963 CrossRef CAS PubMed
.
- D. K. Maity, K. Otake, S. Ghosh and H. Kitagawa, Inorg. Chem., 2015, 56, 1581 CrossRef PubMed
.
- S. Sen, N. N. Nair, T. Yamada, H. Kitagawa and P. K. Bharadwaj, J. Am. Chem. Soc., 2012, 134, 19432 CrossRef CAS PubMed
.
- Y. Ye, W. Guo, L. Wang, Z. Li, Z. Song, J. Chen, Z. Zhang, S. Xiang and B. Chen, J. Am. Chem. Soc., 2017, 139, 15604 CrossRef CAS PubMed
.
- D. Gui, X. Dai, Z. Tao, T. Zheng, X. Wang, M. A. Silver, J. Shu, L. Chen, Y. Wang, T. Zhang, J. Xie, L. Zou, Y. Xia, J. Zhang, J. Zhang, L. Zhao, J. Diwu, R. Zhou, Z. Chai and S. Wang, J. Am. Chem. Soc., 2018, 140, 6146 CrossRef CAS PubMed
.
- Y. Ye, W. Guo, L. Wang, Z. Li, Z. Song, J. Chen, Z. Zhang, S. Xiang and B. Chen, J. Am. Chem. Soc., 2017, 139, 15604 CrossRef CAS PubMed
.
- S. Khatua, A. K. Bar, J. A. Sheikh, A. Clearfield and S. Konar, Chem. – Eur. J., 2018, 24, 872 CrossRef CAS PubMed
.
- M. Sadakiyo, T. Yamada and H. Kitagawa, ChemPlusChem, 2016, 81, 691 CrossRef CAS
.
- D. Umeyama, S. Horike, M. Inukai, Y. Hijikata and S. Kitagawa, Angew. Chem., Int. Ed., 2011, 50, 11706 CrossRef CAS PubMed
.
- N. C. Jeong, B. Samanta, C. Y. Lee, O. K. Farha and J. T. Hupp, J. Am. Chem. Soc., 2012, 134, 51 CrossRef CAS PubMed
.
- K. Maity, T. Kundu, R. Banerjee and K. Biradha, CrystEngComm, 2015, 17, 4439 RSC
.
- J. J. Duan, W. Jin and S. Kitagawa, Coord. Chem. Rev., 2017, 332, 48 CrossRef CAS
.
- A. K. Hughes and K. Wade, Coord. Chem. Rev., 2000, 197, 191–229 CrossRef CAS
.
- N. Burtch, H. Jasuja and K. S. Walton, Chem. Rev., 2014, 114, 10575 CrossRef CAS PubMed
.
- A. Shigematsu, T. Yamada and H. Kitagawa, J. Am. Chem. Soc., 2012, 134, 13145 CrossRef CAS PubMed
.
- M. F. de Lange, K. J. F. M. Verouden, T. J. H. Vlugt, J. Gascon and F. Kapteijn, Chem. Rev., 2015, 115, 12205 CrossRef CAS PubMed
.
- A. K. Gupta, S. S. Nagarkar and R. Boomishankar, Dalton Trans., 2013, 42, 10964 RSC
.
- A. Dey, S. K. Konavarapu, H. S. Sasmal and K. Biradha, Cryst. Growth Des., 2016, 16, 5976 CrossRef CAS
.
- X. Zhang, Y. Zhao, S. Mu, C. Jiang, M. Song, Q. Fang, M. Xue, S. Qiu and B. Chen, ACS Appl. Mater. Interfaces, 2018, 10, 17301 CrossRef CAS PubMed
.
- D. O'Nolan, A. Kumar and M. J. Zaworotko, J. Am. Chem. Soc., 2017, 139, 8508 CrossRef PubMed
.
- C. Wang, X. Liu, N. K. Demir, J. P. Chen and K. Li, Chem. Soc. Rev., 2016, 45, 5107 RSC
.
- J. Canivet, A. Fateeva, Y. Guo, B. Coasne and D. Farrusseng, Chem. Soc. Rev., 2014, 43, 5594 RSC
.
- F. Jeremias, V. Lozan, S. K. Henninger and C. Janiak, Dalton Trans., 2013, 42, 15967 RSC
.
- J. Liu, P. K. Thallapally, B. P. McGrail, D. R. Brown and J. Liu, Chem. Soc. Rev., 2012, 41, 2308 RSC
.
- H. Li, M. Eddaoudi, M. O'Keeffe and O. M. Yaghi, Nature, 1999, 402, 276 CrossRef CAS
.
- A. Dey, D. Bairagi and K. Biradha, Cryst. Growth Des., 2017, 17, 3885–3892 CrossRef CAS
.
- X. Wang, Y. Wang, M. A. Silver, D. Gui, Z. Bai, Y. Wang, W. Liu, L. Chen, J. Diwu, Z. Chai and S. Wang, Chem. Commun., 2018, 54, 4429 RSC
.
- S. Bhattacharya, A. J. Bhattacharyya and S. Natarajan, Inorg. Chem., 2015, 54, 1254 CrossRef CAS PubMed
.
- N. Sikdar, D. Dutta, R. Haldar, T. Ray, A. Hazra, A. J. Bhattacharyya and T. K. Maji, J. Phys. Chem. C, 2016, 120, 13622 CrossRef CAS
.
- M. Garai and K. Biradha, IUCrJ, 2015, 2, 523 CrossRef CAS PubMed
.
- S. M. Aharoni, W. B. Hammond, J. S. Szobota and D. J. Masilamani, Polym. Sci. Educ., 1984, 22, 2579 CAS
.
- S. K. Konavarapu, A. Dey, A. Garai and K. Biradha, Chem. – Eur. J., 2018, 24, 1 CrossRef PubMed
.
- S. S. Park, A. J. Rieth, C. H. Hendon and M. Dincă, J. Am. Chem. Soc., 2018, 140, 2016 CrossRef CAS PubMed
.
- S. C. Sahoo, T. Kundu and R. Banerjee, J. Am. Chem. Soc., 2011, 133, 17950 CrossRef CAS PubMed
.
- L. Khezami and R. Capart, J. Hazard. Mater., 2005, 123, 223 CrossRef CAS PubMed
.
- Y.-Y. Jia, Y.-H. Zhang, J. Xu, R. Feng, M.-S. Zhang and X.-H. Bu, Chem. Commun., 2015, 51, 17439 RSC
.
- J. Tan, B. Zhou, C. Liang, H. Zinky, H.-L. Zhou and Y.-B. Zhang, Mater. Chem. Front., 2018, 2, 129 RSC
.
-
G. M. Sheldrick, SHELXL-2014, University of GÖttingen and Bruker AXS, Karlsruhe, Germany, 2014 Search PubMed
.
-
A. L. Spek, PLATON: A Multi Purpose Crystallographic Tool, Utrecht University, Utrecht, the Netherlands, 2002 Search PubMed
.
Footnote |
† Electronic supplementary information (ESI) available. CCDC 1856603–1856606. For ESI and crystallographic data in CIF or other electronic format see DOI: 10.1039/c8qi01055b |
|
This journal is © the Partner Organisations 2019 |