DOI:
10.1039/C8PY01435C
(Paper)
Polym. Chem., 2019,
10, 627-632
π-Expanded diketopyrrolopyrroles as acceptor building blocks for the formation of novel donor–acceptor copolymers†
Received
5th October 2018
, Accepted 24th December 2018
First published on 9th January 2019
Abstract
The incorporation of rigid and planar, π-extended diketopyrrolopyrrole (EDPP) units into alternating donor–acceptor copolymers results in a variety of low band gap copolymers with thiophene-based EDPP (EDPPT) as acceptor blocks in combination with donor units as thiophene-2,5-diyl or cyclopentadithiophene-2,7-diyl (CPDT). These copolymers are characterized by a broad absorption range in the UV/Vis/NIR region and emission features extending into the Near Infrared (NIR) region. Furthermore, the EDPPT-based copolymers show small optical HOMO/LUMO gaps ranging from 1.65 to 1.81 eV. Replacing the terminal thiophene units of the EDPPT building blocks by fluorene moieties (EDPPF) results in the corresponding EDPPF building blocks for the formation of a second set of EDPPF-based copolymers. These copolymers display distinctly increased HOMO/LUMO energy gaps and well-resolved, mirror-symmetrical absorption and emission features with steep absorption edges and small Stokes shifts when compared to EDPPT copolymers, thus reflecting electronically only weakly interacting EDPPF structural units.
Introduction
Diketopyrrolopyrrole (DPP) derivatives are very stable, electron-poor chromophores that have been extensively used, in combination with electron-rich comonomers, for the construction of various so-called donor–acceptor-type (DA-type) alternating copolymers, for applications e.g. in organic field-effect transistors (OFETs) or organic photovoltaic devices (OPV devices).1–8 After the application of the first DPP-based donor–acceptor-type copolymer (pBBTDPP2) in OPV devices, with a power conversion efficiency (PCE) of 4.0%, by Janssen and coworkers,1 DPP became one of the most frequently used acceptor building blocks in the field of organic optoelectronics.9,10 Until now, a broad range of DPP-based copolymers have been introduced showing PCEs up to 9.4%.11 Most of them only vary in their donor structural unit, which is combined with thiophene-substituted DPP moieties, which are N-alkylated with branched alkyl chains for increased solubility.12,13 For developing novel DPP-based donor–acceptor copolymers also new designs of the DPP-based acceptor building blocks are highly desired. Ladder-type, π-extended DPP (EDPP) derivatives prepared by bridging the two DPP amide nitrogens with neighboring aryl/heteroaryl substituents via vinylene bridges have been introduced by Gryko and co-workers and show distinctly bathochromically shifted absorption features.14,15 Furthermore, the EDPP building block was previously used for the formation of DAD-type small molecules, showing strong red emission, high two-photon absorption cross-sections and promising application potential in cell imaging assays.14,15 Thus, EDPP seems to be a highly promising candidate as a novel acceptor block for the generation of DA-type copolymers. The primary goal of this study is to incorporate such π-extended DPP units, namely EDPPTs with two terminal thiophene rings or EDPPFs with terminal fluorene units, into the corresponding alternating, donor–acceptor copolymers by copolycondensation with suitable, preferably electron-rich comonomers.
Results and discussion
Monomer synthesis
Scheme 1 shows the synthetic pathway towards the thiophene-based, π-expanded diketopyrrolopyrrole-derivatives 7a and 7b (EDPPT). In contrast to the non-alkylated EDPPTs previously reported by Gryko and coworkers,14 it is mandatory to start from alkylated thiophenes (1a and 1b) to ensure an adequate solubility of the corresponding copolymers. First, 1a and 1b were lithiated in the 5-position using the sterically hindered base lithium diisopropylamide (LDA) followed by formylation with dimethylformamide (DMF),16 under generation of 4-alkylthiophene-2-carbaldehydes 2a and 2b in good yields. The aldehydes were oxidized in the presence of conc. aqueous ammonia and iodine to obtain the nitriles 3a and 3b.17 The formation of the nitriles can be monitored by 13C-NMR spectroscopy where the aldehyde resonance at 183 ppm is replaced by the nitrile carbon signal at 115 ppm. DPPs 4a and 4b were generated by condensation with diisopropyl succinate (DIPS) under standard conditions.14 The red-colored solids show very poor solubility in common organic solvents, caused by intermolecular, hydrogen interactions of the strong polar DPP cores.10 However, the addition of trifluoroacetic acid (TFA) inhibits the hydrogen bond interactions and, thus, increases the solubility. Therefore, the bromination of DPP-derivatives 4a and 4b with N-bromosuccinimide (NBS) was carried out in a 1
:
2 mixture of TFA and chloroform, obtaining 5a and 5b as purple-colored solids. To obtain the final EDPPT building blocks, 5a and 5b were first N-alkylated with bromoacetaldehyde diethyl acetal under alkaline conditions in the presence of tetrabutylammonium bisulfate (TBAHS).14 The N-alkylated DPP-derivatives 6a and 6b show an improved solubility and a strong yellow fluorescence in solution. Finally, 6a and 6b were cyclized under “super-acidic” conditions, using an excess of trifluoromethanesulfonic acid (TfOH).14 The solution color immediately turned from deep purple to green after injection of the acid. Monomers 7a and 7b were obtained as blue solids, after work-up and precipitation into methanol, in good overall yields of 24 and 23%, respectively. In addition to the changes in color, the successful implementation of the vinylene bridge between the aromatic substituent and the amide nitrogen results in the generation of two aromatic doublet resonances at 7.86 and 6.73 ppm in the respective 1H-NMR spectra.
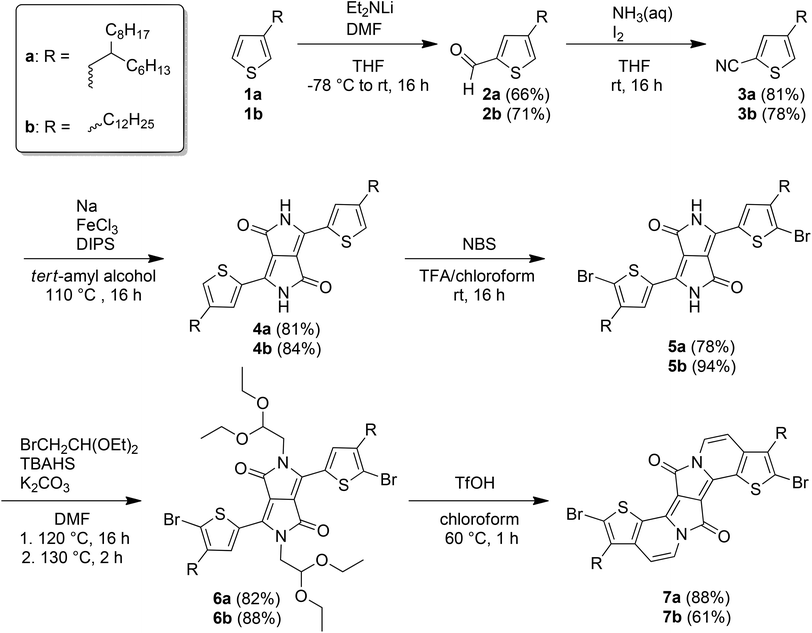 |
| Scheme 1 Synthetic pathway to the EDPPT monomers 7a and 7b. | |
Synthesis of the EDPPT-based copolymers
Previous studies showed that EDPPT derivatives still have a strong electron withdrawing (accepting) character,15 allowing for the formation of novel DA-type copolymers when polymerized with corresponding π-donor comonomers. For this purpose, 7a and 7b were copolymerized with a variety of frequently used thiophene-based donor monomers (see Fig. 1).18 The donor blocks differ considerably in their donor strength and in the number of solubilizing groups, and were generated according to literature procedures.19,20 All copolymers were synthesized by Stille-type polycondensations from 7a or 7b and the corresponding di-stannylated donor monomers in the presence of tetrakis(triphenylphosphine)palladium(0) as a catalyst (see Scheme 2).21 The copolymers were purified by Soxhlet extraction with methanol, acetone, ethyl acetate, hexane and chloroform. The highest molecular weight fractions were used for further investigations.
 |
| Fig. 1 Structures of the EDPPT copolymers. | |
 |
| Scheme 2 General concept for the synthesis of EDPPT-based copolymers. | |
The thiophene- (T), bithiophene- (2T) and thieno[3,2-b]thiophene-based (TT) copolymers PEDDPTHD-T, PEDDPTHD-2T and PEDDPTHD-TT were generated from 7a (with bulky 2-hexyldecyl sidechains) in good to moderate yields of 50, 58 and 31%, respectively. In particular, PEDDPTHD-2T and PEDDPTHD-TT show very poor solubility in chloroform. In the case of PEDDPTHD-TT, the polymer already precipitated during the reaction, thus lowering the yield and molecular weight significantly. PEDDPTHD-T shows the highest number-average molecular weight (Mn) of 23.1 kDa, while PEDDPTHD-2T and PEDDPTHD-TT show lower Mn values of 14.6 and 10.4 kDa, respectively. In comparison with conventional DPP-polymers, which can reach molecular weights >50 kDa, the strong π–π-stacking tendency of the EDPPT-based polymers may increase the aggregation potential and inhibit the formation of longer polymer chains, even when decorated with bulky 2-hexyldecyl sidechains.22 To exclude that aggregation effects affect the obtained molecular weight distributions, the Mn of PEDDPTHD-T was crosschecked by the usage of high temperature GPC at 160 °C with trichlorobenzene as the solvent resulting in nearly similar values of Mn = 22.2 kDa. Also a change of the polymerization conditions to Pd2(dba)3/PPh3 as a catalyst and toluene/DMF as a solvent did not result in increased molecular weights. Therefore, we further tried to increase the molecular weights of the EDPPT-based copolymers by using other donor building blocks that contain additional solubilizing groups (see Fig. 1, bottom).
Surprisingly, the copolymerization of 7a with alkylated donor comonomers did not increase the molecular weights of the corresponding polymers in comparison with the previously introduced copolymers. The benzodithiophene (BDT)-based copolymer PEDDPTHD-BDT was synthesized in 68% yield, showing a molecular weight of Mn = 23.3 kDa, which is comparable to PEDDPTHD-T. The copolymerization of 7a with cyclopentadithiophene- (CPDT) and dithienopyrrole- (DTP)-based comonomers only resulted in the formation of oligomers (PEDDPTHD-CPDT: Mn = 5.6 kDa and PEDDPTHD-DTP: Mn = 2.7 kDa). In comparison, when using the EDPPT-based monomer 7b with linear alkyl chains, 2–3 times higher molecular weights have been achieved (PEDDPTDo-CPDT: Mn = 12.6 kDa and PEDDPTDo-DTP: Mn = 7.6 kDa). The higher molecular weights of PEDDPTDo-CPDT and PEDDPTDo-DTP may result from the sterically less demanding situation during coupling with comonomers containing linear alkyl sidechains. Concurrently, linear sidechains may support an intermolecular π–π-stacking of the EDPPT-units leading to reduced polymer solubility. Detailed data on molecular weights and yields can be found in the ESI.†
The changes in the optical properties, going ahead with the planarization of the π-system when going from DPP to EDPP and during the incorporation of EDPP into a donor–acceptor copolymer, are illustrated in Fig. 2. The absorption maximum of DPP 4a peaks at 531 nm, while a distinct bathochromic shift to 625 nm is observed after cyclization (7a), accompanied by a color change from red to deep blue in the solid state (see Fig. 3) as a result of an extension of the π-conjugated system.14 In the photoluminescence (PL) spectra, the emission maximum of 4a (λmax. = 543 nm) is also shifted to higher wavelengths after cyclization (7a: λmax. = 634 nm). The corresponding copolymers show further red-shifted and broadened absorption and PL bands and a slightly increased Stokes shift. As shown for PEDDPTHD-T, the formation of the DA-type copolymer results in the expansion of absorption into the NIR region, with a broad absorption range from 500 up to 900 nm. The long wavelength absorption band peaks at 776 nm, with an intense shoulder at 724 nm. Furthermore, the absorption in the lower wavelength region (λmax. = 399 nm) is strongly increased in comparison with that of 4a and 7a. The emission maximum of PEDDPTHD-T is shifted towards the NIR region (λmax. = 764 nm, with a shoulder around 840 nm). No fluorescence is visible to the human eye (see Fig. 3). In comparison with a non-bridged DPP copolymer with a similar backbone structure (PDPP3T),12 the absorption and emission features are blue shifted probably due to the lower molecular weight of PEDPPTHD-T (longest wavelength absorption maximum for PEDPPTHD-T: 780 nm vs. 830 nm for PDPP3T). The wider bandgap value (1.69 eV for PEDPPTHD-Tvs. 1.33 eV for PDPP3T) is reflected in the corresponding HOMO and LUMO energy levels: HOMO: for PEDPPTHD-T −5.00 eV vs. −5.30 eV for PDPP3T; LUMO: for PEDPPTHD-T −3.31 eV vs. −3.74 eV for PDPP3T. The photoluminescence of PEDPPTHD-T is weak as known for related DPP-derivatives.
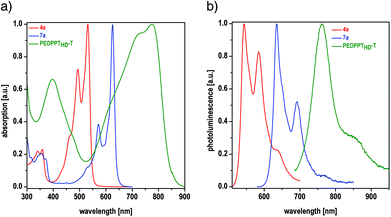 |
| Fig. 2 Normalized UV/Vis absorption (a) and photoluminescence (PL) (b) spectra of 4a, 7a and PEDDPTHD-T in chloroform. | |
 |
| Fig. 3 Top: Photographic images of 4a, 7a and PEDPPTHD-T powders; Bottom: photographic images of 4a, 7a and PEDPPTHD-T solutions in chloroform under day light (left); photographic images of 4a, 7a and PEDPPTHD-T, in chloroform solution under UV-light illumination, excited at 365 nm (right). | |
The optical properties of the EDPPT-based copolymers are depicted in Fig. 4. For a better overview, only one polymer of each backbone type (HD or Do sidechain, in each case the copolymer with the higher molecular weight) is further discussed. UV/vis and PL solution spectra were recorded in chloroform; solid state UV/vis spectra were obtained from spin-coated polymer films (spin coating from 8 mg ml−1 solutions in chloroform) on quartz glass plates. All copolymers show absorption and fluorescence features that are extended into the NIR region, with absorption maxima (in solution) in a range from 683 nm (PEDDPTHD-BDT) up to 776 nm (PEDDPTHD-T). Moreover, more intense absorption features also appear in the UV range at around 400 nm in comparison with the monomers (7a and 7b). For those copolymers bearing no additional solubilizing alkyl groups at the donor units, strong aggregation behavior in solution is observed, as evident from the appearance of additional low energy absorption bands, not only in the film absorption spectra. Even at low concentrations, the less soluble PEDDPTHD-T and PEDDPTHD-2T copolymers show only minor differences in the shape and position of the absorption spectra between the solid state and solution spectra as indication of the high tendency for π–π-interactions and aggregation of the EDPP moieties already in solution. Beside the T- and 2T-based copolymers, all other copolymers show the additional bathochromically shifted and broadened absorption features only in the solid state. In particular, the absorption maximum of PEDDPTHD-DTP is red-shifted from 740 to 771 nm with an absorption onset at >950 nm.
 |
| Fig. 4 Top: Normalized UV/Vis absorption spectra of EDPPT-based copolymers in chloroform (left); normalized photoluminescence (PL) emission spectra in chloroform (excitation wavelengths are listed in Table 1, right); Bottom: normalized solid state UV/Vis absorption spectra of thin polymer films. | |
The emission maxima of the EDPPT-based copolymers range from 700 to 800 nm. In addition, all polymers show a broad emission shoulder (vibrational progression) at a higher wavelength. Again, PEDDPTHD-BDT shows the highest energy PL maximum (723 nm), while the emission maximum of PEDDPTDo-CPDT is red-shifted to 800 nm, most probably caused by the stronger electron-donating character of the CPDT-unit thus increasing the donor–acceptor character of the copolymer. The Stokes shifts of the EDPP-based copolymers are increased in comparison with those of the monomers 7a and 7b, and range from 21 nm (PEDDPTHD-2T) to 70 nm (PEDDPTDo-CPDT). Exceptions are observed for PEDDPTHD-T and PEDDPTHD-TT. Due to the strong aggregation in solution, the determined “negative” Stokes shift is virtually caused by the fact that the emitting species are not the aggregates causing the longest wavelength absorption band. All in all, the EDPPT-polymers show attractive optical properties with absorption and emission features shifted into the NIR region if compared to most of the previously published, “conventional” DPP-polymers.18
The electronic energy levels, namely the energetic position of the HOMO/LUMO levels, of the polymers are depicted in Fig. 5. All polymers can be described as low bandgap polymeric semiconductors with optical bandgaps below 2 eV. The smallest HOMO/LUMO gap is observed for PEDDPTDo-DTP (1.60 eV). Furthermore, PEDDPTDo-DTP, PEDDPTDo-CPDT and PEDDPTHD-BDT show nearly similar HOMO values of −5.18, −5.19 and −5.21 eV, respectively. For these polymers, the position of the LUMO level seems to be mainly determined by the EDPPT acceptor moiety. Increasing the donor strength of the electron-rich comonomer building blocks from BDT to DTP results in an increased HOMO energy level with a decreased HOMO/LUMO gap. A similar clear trend could not be observed for the T-, 2T- and TT-based copolymers, maybe due to differences in the molecular weights, and thus in the lengths of effectively conjugated segments.
 |
| Fig. 5 Electronic properties of the EDPPT-based copolymers; HOMO levels are estimated by photoelectron spectroscopy; optical bandgaps (Eg) are calculated from the onset of absorption in the solid state; LUMO levels are determined as the difference of the HOMO and Eg. | |
EDPPF-based copolymers
In addition to the previously presented DA-type copolymers, two other copolymers were synthesized from a fluorene-based EDPP monomer (EDPPF) (see Fig. 6). Monomer 8 was synthesized according to a literature procedure.15 With a total number of ten annulated rings, monomer 8 is a π-expanded DPP derivative with a fully planar structure and was copolymerized with distannylated BDT and CPDT comonomers in Stille-type couplings, as already described for the EDPPT-based copolymers, to afford PEDPPF-BDT and PEDPPF-CPDT in moderate yields of 56 and 44% with comparably high Mn values of 23.4 and 16.2 kDa, respectively. Surprisingly, the EDPPF-based copolymers show significant differences in their optical properties when compared to their EDPPT-based counterparts (see Fig. 7). The absorption maximum of PEDPPF-BDT (645 nm) is only slightly red-shifted (by 15 nm) if compared to that of monomer 8, still showing distinct vibronic progressions with sharp absorption and emission edges, strong red fluorescence in solution and a small Stokes shift (10 nm). Similar findings apply for PEDPPF-CPDT with an absorption maximum at 665 nm. This behavior suggests a less pronounced π-conjugation, both on intra- and intermolecular scales, most probably caused by a significant distortion at the EDPPF-BDT or PEDPPF-CPDT single bond linkages. It is expected that the EDPPF-based copolymers show no or very weak push–pull (charge transfer) interactions between donor and acceptor units (EDPPF). Furthermore, the HOMO/LUMO gaps of the EDPPF-based copolymers are much larger (see Table 1) than those of the EDPPT-based copolymers.
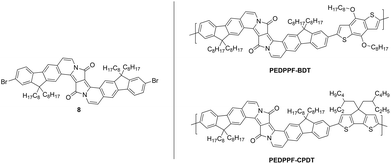 |
| Fig. 6 Structure of the EDPPF monomer 8 and the corresponding EDPPF-based copolymers PEDPPF-BDT and PEDPPF-CPDT. | |
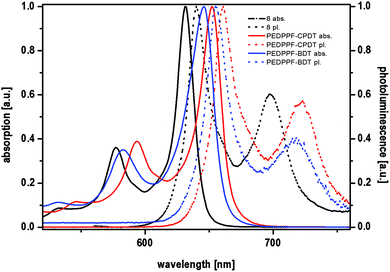 |
| Fig. 7 Normalized absorption and PL spectra of 8, PEDPPF-CPDT and PEDPPF-BDT in chloroform. | |
Table 1 Optical and electronic properties of the EDPPT and EDPPF copolymers
Compound |
λ
max.abs. a [nm] |
λ
max.abs. b [nm] |
λ
max.em. c [nm] |
E
HOMO [eV] |
E
LUMO [eV] |
E
opt.g [eV] |
Absorption maxima in chloroform.
Absorption maxima in a thin film.
Emission maxima in chloroform; excitation wavelengths are given in brackets.
|
PEDPPTHD-T
|
776 |
780 |
764 (670) |
−5.00 |
−3.31 |
1.69 |
PEDPPTHD-2T
|
710 |
806 |
731 (640) |
−5.08 |
−3.35 |
1.73 |
PEDPPTHD-TT
|
721 |
735 |
739 (640) |
−4.83 |
−3.18 |
1.65 |
PEDPPTHD-BDT
|
683 |
739 |
723 (650) |
−5.21 |
−3.40 |
1.81 |
PEDPPTDo-DTP
|
740 |
771 |
759 (670) |
−5.18 |
−3.58 |
1.60 |
PEDPPTDo-CPDT
|
730 |
732 |
800 (730) |
−5.19 |
−3.47 |
1.72 |
PEDPPF-BDT
|
652 |
662 |
662 (400) |
−5.41 |
−3.33 |
2.08 |
PEDPPF-CPDT
|
665 |
669 |
675 (650) |
−5.26 |
−3.20 |
2.06 |
Conclusions
In summary, we have successfully synthesized the first EDPPT-based, alternating donor–acceptor copolymers with Mn's up to 23.3 kDa (PEDDPTHD-BDT). All copolymers show broad absorption regions from 500 to 900 nm, with absorption and emission features extended into the NIR region. In combination with small bandgaps and strong aggregation tendency already in solution, especially PEDDPTHD-T and PEDDPTHD-2T seem to be promising candidates for applications in organic, optoelectronic devices. By replacing the terminal thiophene by fluorene units, two EDPPF-based copolymers were also generated. The absorption spectra of PEDPPF-BDT and PEDPPF-CPDT are only slightly red-shifted when compared to the monomeric EDPPF chromophore. Steep absorption and emission edges and small Stokes shifts point to a distinctly reduced donor–acceptor interaction and mirror the optical properties of isolated EDPPF chromophores.
Conflicts of interest
There are no conflicts to declare.
Acknowledgements
Funded by the Deutsche Forschungsgemeinschaft (DFG, German Research Foundation) – SCHE 410/24-3.
Notes and references
- M. M. Wienk, M. Turbiez, J. Gilot and R. A. J. Janssen, Adv. Mater., 2008, 20, 2556 CrossRef CAS.
- K. H. Hendriks, W. Li, M. M. Wienk and R. A. J. Janssen, J. Am. Chem. Soc., 2014, 136, 12130 CrossRef CAS PubMed.
- J. C. Bijleveld, A. P. Zoombelt, S. G. J. Mathijssen, M. M. Wienk, M. Turbiez, D. M. de Leeuw and R. A. J. Janssen, J. Am. Chem. Soc., 2009, 131, 16616 CrossRef CAS PubMed.
- H.-W. Lin, W.-Y. Lee and W.-C. Chen, J. Mater. Chem., 2012, 22, 2120 RSC.
- J. S. Lee, S. K. Son, S. Song, H. Kim, D. R. Lee, K. Kim, M. J. Ko, D. H. Choi, B. Kim and J. H. Cho, Chem. Mater., 2012, 24, 1316 CrossRef CAS.
- J. D. Yuen, J. Fan, J. Seifter, B. Lim, R. Hufschmid, A. J. Heeger and F. Wudl, J. Am. Chem. Soc., 2011, 133(51), 20799 CrossRef CAS PubMed.
- Y. Li, P. Sonar, S. P. Singh, M. S. Soh, M. van Meurs and J. Tan, J. Am. Chem. Soc., 2011, 133, 2198 CrossRef CAS PubMed.
- Z. Chen, M. J. Lee, R. Shahid Ashraf, Y. Gu, S. Albert-Seifried, M. Meedom Nielsen, B. Schroeder, T. D. Anthopoulos, M. Heeney, I. McCulloch and H. Sirringhaus, Adv. Mater., 2012, 24, 647 CrossRef CAS PubMed.
- C. B. Nielsen, M. Turbiez and I. McCulloch, Adv. Mater., 2013, 25, 1859 CrossRef CAS PubMed.
- M. Grzybowski and D. T. Gryko, Adv. Opt. Mater., 2015, 3, 280 CrossRef CAS.
- H. Choi, S.-J. Ko, T. Kim, P.-O. Morin, B. Walker, B. H. Lee, M. Leclerc, J. Y. Kim and A. J. Heeger, Adv. Mater., 2015, 27, 3318 CrossRef CAS PubMed.
- K. H. Hendriks, G. H. L. Heintges, V. S. Gevaerts, M. M. Wienk and R. A. J. Janssen, Angew. Chem., Int. Ed., 2013, 125, 8499 CrossRef.
- W. Li, K. H. Hendriks, A. Furlan, W. S. C. Roelofs, M. M. Wienk and R. A. J. Janssen, J. Am. Chem. Soc., 2013, 135, 18942 CrossRef CAS PubMed.
- M. Grzybowski, E. Glodkowska-Mrowka, T. Stoklosa and D. T. Gryko, Org. Lett., 2012, 14, 2670 CrossRef CAS PubMed.
- M. Grzybowski, V. Hugues, M. Blanchard-Desce and D. T. Gryko, Chemistry, 2014, 20, 12493 CrossRef CAS PubMed.
- P. Innocenti, K.-M. J. Cheung, S. Solanki, C. Mas-Droux, F. Rowan, S. Yeoh, K. Boxall, M. Westlake, L. Pickard, T. Hardy, J. E. Baxter, G. W. Aherne, R. Bayliss, A. M. Fry and S. Hoelder, J. Med. Chem., 2012, 55, 3228 CrossRef CAS PubMed.
- C. Quinton, V. Alain-Rizzo, C. Dumas-Verdes, G. Clavier, L. Vignau and P. Audebert, New J. Chem., 2015, 39(12), 9700 RSC.
- W. Li, K. H. Hendriks, M. M. Wienk and R. A. J. Janssen, Acc. Chem. Res., 2016, 49, 78 CrossRef CAS PubMed.
- X. Guo, F.
S. Kim, M. J. Seger, S. A. Jenekhe and M. D. Watson, Chem. Mater., 2012, 24, 1434 CrossRef CAS.
-
U. Asawapirom, Flüssigkristalline Polymere und Copolymere auf Thiophenbasis, Bergische Universität Wuppertal, Germany, 2003 Search PubMed.
- Z. Zhu, D. Waller, R. Gaudiana, M. Morana, D. Mühlbacher, M. Scharber and C. Brabec, Macromolecules, 2007, 40, 1981 CrossRef CAS.
- K. H. Hendriks, W. Li, M. M. Wienk and R. A. J. Janssen, Adv. Energy Mater., 2013, 3, 674 CrossRef CAS.
Footnote |
† Electronic supplementary information (ESI) available. See DOI: 10.1039/c8py01435c |
|
This journal is © The Royal Society of Chemistry 2019 |
Click here to see how this site uses Cookies. View our privacy policy here.