Diphenylaminostyryl-substituted quinolizinium derivatives as fluorescent light-up probes for duplex and quadruplex DNA†
Received
26th February 2019
, Accepted 10th March 2019
First published on 14th March 2019
Abstract
(E)-2-[1′-((Diphenylamino)styryl)quinolizinium (3a) and 2,2′-{(phenylimino)-bis[(E)-1′′,1′′′-styryl]}-bis[quinolizinium] (3b) were synthesized, and their interactions with duplex DNA and quadruplex DNA were investigated with a particular focus on their ability to operate as DNA-sensitive fluorescent probes. Due to the significantly different size and steric demand of these quinolizinium derivatives they exhibit different binding modes. Thus, 3a intercalates into duplex DNA and binds through π stacking to quadruplex DNA, whereas 3b favours groove binding to both DNA forms. The emission intensity of these compounds is very low in aqueous solution, but it increases drastically upon association with duplex DNA by a factor of 11 (3a) and >100 (3b) and with quadruplex DNA by a factor of >100 (3a) and 10 (3b), with emission bands between 600 and 750 nm.
Introduction
The detection of nucleic acids is an important tool in clinical, forensic, and biological studies and applications.1 And among the different chemical or biological tools that may be used for DNA analysis,2 emission spectroscopy is one of the most versatile and efficient methods.3 As a result, numerous fluorescent probes have been reported that allow the staining as well as qualitative and quantitative detection of DNA in cell-free medium and in cells.4 Specifically, these molecular probes that indicate the nucleic acid selectively with an increase in the emission intensity upon association with the biomacromolecule (light-up probes) are very useful fluorimetric markers.5 Along with the regular duplex DNA there is also an increasing interest in the detection of non-canonical DNA forms, with G-quadruplex DNA (G4-DNA) being the most prominent one. As this DNA form is proposed to have essential biological relevance and function,6 probes have been developed recently that enable its selective fluorimetric detection.7
In this context, it has been demonstrated that styryl dyes with a cationic hetarene unit exhibit favourable properties for DNA sensing:10–12 (i) the cationic charge of the dyes increases the affinity of the probe to DNA;8 (ii) in donor–acceptor substituted styryl dyes ICT (intramolecular charge transfer) or TICT (twisted intramolecular charge transfer) states are possible, and these properties may change upon binding of the dye to DNA, thus enabling sensitive detection; and (iii) the large Stokes shifts in donor–acceptor substituted styryl dyes reduce the interference between excitation and detection light.8,9 As a result, styryl dyes are widely used as probes in fluorescence-based bioanalytical applications, specifically DNA staining.10–12
In our studies on the development of DNA-sensitive fluorescent probes, we have demonstrated that annelated quinolizinium derivatives are versatile DNA-binding ligands that may be used for fluorimetric detection.13 In addition, we have shown that especially donor-substituted quinolizinium derivatives have favourable photophysical properties and may therefore be used as versatile building blocks in fluorescent light-up probes.14 Based on these observations we proposed that styryl-substituted quinolizinium derivatives may also be promising DNA-sensitive light-up probes with useful absorption and emission properties. So far, only a few styrylquinolizinium derivatives are known, and some of these have been already applied for fluorimetric DNA detection.15 Among the derivatives investigated so far, the aminostyryl-substituted quinolizinium derivatives appeared to be the most promising candidates as they exhibit the typical advantages of donor–acceptor dyes. Therefore, we decided to vary this motif and employ a diphenylaminostyryl substituent as a donor unit, because the triarylamine unit is well known for its strong electron-donating properties,16 and it was already shown to be a useful functionality in fluorescent chemosensors.17 Herein, we present the synthesis of the resulting diphenylamino-substituted styrylquinolizinium derivatives 3a and 3b along with investigations of their interactions with duplex and quadruplex DNA with a particular focus on their potential application as DNA-sensitive fluorescent probes.
Results
Synthesis
The styrylquinolizinium derivatives 3a and 3b were synthesized by a Knoevenagel-condensation18 of 2-methylquinolizinium (1)19 with the mono- and diformyl-substituted triphenylamine derivatives 2a and 2b20 in 70% and 67% yield, respectively (Scheme 1). The chemical structures of the ligands were confirmed by 1D and 2D 1H NMR and 13C NMR spectroscopy, mass spectrometry and elemental analysis (cf. ESI†).
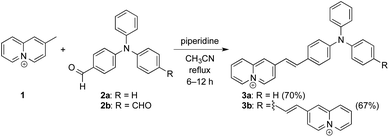 |
| Scheme 1 Synthesis of styrylquinolizinium derivatives 3a and 3b. | |
Absorption and emission properties
The absorption and emission properties of compounds 3a and 3b were investigated in different solvents, namely CH2Cl2, CHCl3, CH3CN, MeOH, EtOH, DMSO and aqueous buffer at pH 7. The absorption spectra of 3a and 3b display a long-wavelength absorption maximum ranging from 450 nm (3a) and 473 nm (3b) in aqueous buffer to 500 nm (3a) and 512 nm (3b) in CH2Cl2 (Table 1, Fig. S1†). In most solvents, the emission intensity of derivatives 3a and 3b is very weak. Thus, in DMSO, MeOH, CH3CN and buffer solution only a weak, broad emission band was observed between 600 and 750 nm, whereas in the protic polar solvent EtOH the emission intensity at 695 nm is slightly higher.
Table 1 Absorption and emission properties of 3a and 3b in different solvents
Solvent |
λ
abs
/nm |
lg εb |
λ
fl
/nm |
λ
abs – λfld/cm−1 |
Φ
fl
|
Long-wavelength absorption maximum; c = 10 μM.
ε = Molar extinction coefficient in cm−1 M−1.
Fluorescence emission maximum; 3a: λex = 460 nm, 3b: λex = 500 nm.
Difference between absorption maximum and emission maximum at lowest energy.
Fluorescence quantum yield relative to rhodamine B in EtOH (Φfl = 0.7) (ref. 21).
In 10 mM BPE buffer solution at pH 7.0 and 20 °C.
|
3a
|
CHCl3 |
495 |
4.57 |
638 |
6993 |
0.34 |
CH2Cl2 |
500 |
4.56 |
695 |
5128 |
0.21 |
DMSO |
458 |
4.55 |
725 |
3745 |
0.02 |
CH3CN |
460 |
4.58 |
736 |
3623 |
0.01 |
EtOH |
472 |
4.59 |
695 |
4484 |
0.04 |
MeOH |
465 |
4.60 |
710 |
4081 |
0.01 |
Bufferf |
450 |
4.12 |
660 |
4716 |
<0.01 |
3b
|
CHCl3 |
513 |
4.71 |
620 |
9345 |
0.27 |
CH2Cl2 |
512 |
4.65 |
690 |
5617 |
0.12 |
DMSO |
480 |
4.75 |
740 |
3846 |
<0.01 |
CH3CN |
480 |
4.77 |
738 |
3875 |
<0.01 |
EtOH |
490 |
4.69 |
695 |
4878 |
0.03 |
MeOH |
484 |
4.79 |
715 |
4329 |
0.02 |
Bufferf |
473 |
4.23 |
682 |
4784 |
<0.01 |
In contrast, both 3a and 3b show more intense emission in the less polar, chlorinated solvents CHCl3 (3a: 638 nm, 3b: 620 nm) and CH2Cl2 (3a: 695 nm, 3b: 690 nm). In general, the emission quantum yields in CHCl3 (Φfl = 0.34 and 0.27) are higher as compared to those in CH2Cl2 (Φfl = 0.21 and 0.12) (cf. ESI, Fig. S1, B1 and B2†).
To assess whether the low emission quantum yields of compounds 3a and 3b are caused by conformational changes in the excited state, the fluorescence spectra were recorded in media of high viscosity, namely aqueous solutions with increasing glycerol content.22 Whereas in aqueous solution compounds 3a and 3b are basically non-fluorescent (Φfl ≈ 0.002), an increasing content of glycerol (wt% glycerol: 0, 50, 100%) resulted in a large increase in the fluorescence quantum yield (Φfl = 0.30 for 3a and Φfl = 0.14 for 3b), along with a red shift of the absorption maxima from λabs = 450 nm to 472 nm (3a) and λabs = 473 nm to 493 nm (3b) (Fig. S2†).
DNA binding properties
Spectrophotometric and spectrofluorimetric titrations.
The interactions of derivatives 3a and 3b with calf thymus (ct) DNA and the quadruplex forming oligonucleotide d[A(GGGTAA)3GGG] (22AG) were monitored by photometric and fluorimetric titrations in BPE buffer solution (ct DNA) or in K-phosphate buffer (22AG) at 20 °C and pH 7 (Fig. 1 and 2). In both cases, the absorption spectrum of the quinolizinium derivatives 3a and 3b changed significantly upon addition of ct DNA and 22AG. Thus, upon addition of ct DNA to a solution of 3a or 3b, the absorbance of these compounds firstly decreased with a slight red shift on addition of ct DNA until ligand–DNA ratios (LDR) of >1.4 (3a) and >0.2 (3b) were achieved. Further addition of ct DNA led to an increase in the absorbance with a red shift of the absorption maximum to 470 nm (Δλ = 20 nm; 3a) and 513 nm (Δλ = 40 nm, 3b) (Fig. 1A). On titration of 22AG to compounds 3a and 3b the absorbance at 450 nm (3a) and 473 nm (3b) decreased until LDRs of >20 (3a) and >18.6 (3b) were achieved. At a higher concentration of 22AG (LDR <0.4 and <0.7, resp.) a red shifted absorption peak was formed at 480 nm (Δλ = 30 nm) (3a) and 505 nm (Δλ = 32 nm) (3b) (Fig. 1B). The data from spectrophotometric titrations were used to determine the binding constant, Kb, obtained from the fitting of the experimental binding isotherms to the theoretical model (cf. ESI, Fig. S3†).24 Thus, ligands 3a and 3b bind to ct DNA with binding constants of Kb = 4.3 × 104 M−1 and Kb = 8.8 × 104 M−1.
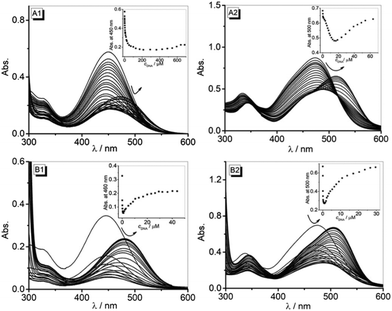 |
| Fig. 1 Photometric titration of 3a (1) and 3b (2) with ct DNA (A, c = 1.88 mM in base pairs) in BPE buffer (10 mM, pH 7.0; solutions of ligands with 10% v/v DMSO, cLigand = 20 μM) and with 22AG (B, c = 200 μM) in K-phosphate buffer at pH 7.0 at 20 °C (cLigand = 20 μM). The arrows indicate the changes of absorption upon addition of DNA. Inset: Changes of the ligand absorption with increasing DNA concentration. | |
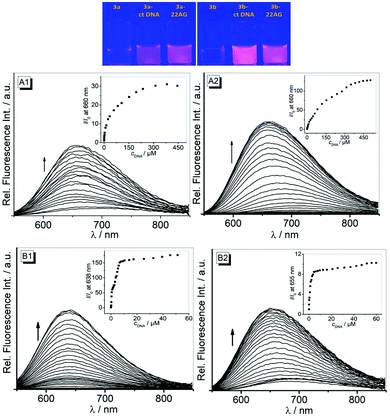 |
| Fig. 2 Fluorimetric titration of 3a (1) and 3b (2) (c = 10 μM) with ct DNA (A, c = 1.88 mM) in BPE buffer (10 mM; solutions of ligands with 10% v/v DMSO) and with 22AG (B, c = 200 μM) in K-phosphate buffer; pH = 7.0, T = 20 °C, 3a: λex = 460 nm, 3b: λex = 500 nm. The arrows indicate the changes of emission intensity upon addition of DNA. Inset: Plot of relative fluorescence intensity of 3a and 3bversus cDNA (corrected with regard to the change of the absorption at the excitation wavelength). Top: Pictures of emission color of 3a and 3b in the absence and presence of DNA. | |
The emission intensities of both compounds 3a and 3b increased significantly in the presence of DNA (Fig. 2). Thus, the addition of ct DNA and 22AG to derivative 3a resulted in a continuous increase of the weak emission band by a factor of 30 (ct DNA, Φfl = 0.16) and 176 (22AG, Φfl = 0.26) and small blue shifts (Δλ ≈ 20 nm) of the emission maximum (Fig. 2, A1 and B1).
Similarly, the weak emission of compound 3b increased by factors of 128 and 10 on addition of ct DNA (Φfl = 0.40) and 22AG (Φfl = 0.13), respectively, and the emission maxima were slightly blue shifted to 660 nm and 655 nm on addition of ct DNA and 22AG (Fig. 2, A2 and B2). From the fluorescence titration experiments, the limit of detection (LOD) of 3a and 3b was estimated to be 0.02 μM and 0.79 μM for ct DNA and 0.05 μM and 0.01 μM for 22AG, respectively (cf. ESI, Table S1†).23
To check whether the fluorimetric analysis is disturbed by the formation of singlet oxygen and subsequent DNA damage, the irradiation of a 3b–DNA complex (LDR = 0.3) under aerobic conditions was monitored by CD spectroscopy (cf. ESI, Fig. S5†). Under these conditions, no significant changes in the CD signals corresponding to the DNA absorption (<300 nm) were observed, which indicates negligible changes in the DNA structure after irradiation. In another experiment, we examined the influence of oxygen on the excited singlet state of 3b by recording emission spectra at different oxygen concentrations. Namely, the spectra were measured under anaerobic conditions, in air or in oxygen-saturated solution under otherwise identical conditions (cf. ESI, Fig. S6†). Notably, the fluorescence intensity of ligand 3b and of the 3b–ct DNA complex is essentially independent of the oxygen concentration, which indicates that oxygen does not significantly quench the excited singlet state.
CD- and LD-spectroscopic analysis
Complementary analysis with circular dichroism (CD) and flow linear dichroism (LD) spectroscopy revealed that in the presence of ct DNA, ligands 3a and 3b display induced circular dichroism (ICD) and LD bands in the absorption range of the ligands (Fig. 3). A solution of ligand 3a with ct DNA exhibited negative ICD bands at 247 nm, 310 nm, 420 nm and 500 nm, all of which increased in intensity from LDR = 0.3 to 1.0. At higher LDR values, however, the negative long-wavelength bands at 420 nm and 500 nm developed into a very intense bisignate band with zero transition at the absorption maximum. Simultaneously, the initially intense positive CD signal of the DNA at 278 nm decreased drastically (Fig. 3, A1). At the same time, the addition of ct DNA to the solution of ligand 3a (LDR = 0–1.0) led to the formation of a gradually increasing negative LD signal in the absorption region of the ligand (350–550 nm). Notably, the intensity of the negative LD signal at 258 nm decreased with increasing LDR values (Fig. 3, B1).
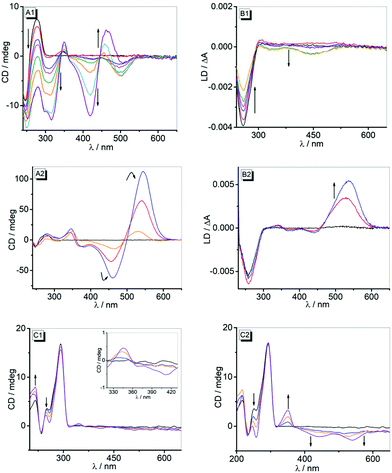 |
| Fig. 3 CD spectra (A) and LD spectra (B) of ct DNA (c = 50 μM) in the absence and presence of 3a (1) and 3b (2) at LDR = 0 (black), 0.3 (red), 0.5 (blue), 0.6 (magenta), 0.8 (green), 1.0 (orange), 1.5 (cyan), 2.0 (violet) in BPE buffer solution (10 mM, pH 7.0; solutions of ligands with 10% v/v DMSO). CD spectra of 22AG (c = 20 μM) in the absence and presence of 3a (C1) and 3b (C2) at LDR = 0 (black), 0.5 (blue), 1.0 (orange), 2.0 (violet) in K-phosphate buffer at pH 7 at 20 °C (solutions of ligands with 1% DMSO). | |
The complexation of compound 3b with ct DNA resulted in the formation of positive ICD signals at 347 nm and 545 nm, along with a negative signal at 460 nm. Up to an LDR value of 0.5, the intensities of the two very strong positive CD bands at 545 nm and 460 nm increased, but at a larger LDR of 1.0 these CD bands decreased (Fig. 3, A2). The LD spectra of complexes of 3b and ct DNA revealed a very strong positive band at 536 nm that increased from LDR = 0.3 to 0.5 and subsequently disappeared at larger LDR = 1 in favour of a weak bisignate band with maxima at 540 nm and 440 nm. Concurrently, very weak positive and negative LD signals were observed at 340 nm (+), 375 nm (−) and 440 nm (−) that strongly broadened with increasing LDR values (Fig. 3, B2).
On addition of derivatives 3a and 3b to G4-DNA 22AG the characteristic CD pattern of the hybrid-type structure of the quadruplex, i.e. with positive bands at 290 nm and 270 nm along with a negative band at 235,25 did not change essentially (Fig. 3, C1 and C2). In the complex of 3a and 22AG, only a very weak bisignate ICD band was observed with maxima at 345 nm (+) and 410 nm (−) (Fig. 3, C1). In contrast, more pronounced ICD signals developed in the absorption range of the derivative 3b upon addition of G4-DNA 22AG. Specifically, with increasing LDR from 0 to 2.0, a positive band at 350 nm and two very broad negative bands with maxima at 440 nm and 540 nm were observed (Fig. 3, C2). CD-spectroscopic analysis was also performed to determine the influence of the association of 3a and 3b on the quadruplex melting temperature, Tm (cf. ESI, Fig. S7†). Thus, the determination of the DNA melting temperature by monitoring the temperature-dependent CD intensity revealed an increase in the melting temperature, ΔTm, in the presence of the ligands (3a: ΔTm = 3 °C, 3b: ΔTm = 9 °C, at LDR = 2).
Discussion
The absorption and emission properties of 3a and 3b in different solvents resemble the ones that have been reported previously for styryl substituted quinolizinium derivatives.15d Although the absorption and emission shifts of these compounds are slightly different in various polar aprotic and polar protic solvents (Table 1), there was no clear relationship identified between the absorption and emission energy and a particular solvent property. This observation indicates that several solvent properties, such as polarity and hydrogen bonding, contribute to the overall solvent effect to different extents. Notably, in CH2Cl2 and CHCl3, derivatives 3a and 3b exhibit significant red shifts of the absorption bands (Fig. S1†) which are frequently observed for cationic dyes due to the high polarizability of chloroalkane solvents.26 In addition, it should be considered that these compounds are poorly soluble in these solvents, which leads to dye aggregation. Thus, the red-shifted absorption in these solvents may indicate the aggregate formation.27 The emission intensity of 3a and 3b is very low in polar solvents (<0.04), most likely due to the radiationless deactivation of the excited state by conformational changes, such as rotation about the vinyl–arene bond, as commonly observed for styryl dyes.28 This assumption was clearly supported by the observation that the emission intensity increases with increasing viscosity of the solution, namely in glycerol–water mixtures (cf. ESI, Fig. S2†), as the rotational relaxation of the excited molecule becomes slower than the fluorescence at higher viscosity. Presumably, the slightly larger emission quantum yields of 3a and 3b in CHCl3 and CH2Cl2 (Φfl = 0.1 to 0.3) are the result of their low solubility in these solvents and are thus caused by an aggregation-induced enhancement of the emission intensity.29
The binding studies of 3a and 3b with ct DNA and 22AG by photometric, fluorimetric and polarimetric titrations clearly confirmed the association of these ligands with DNA. Firstly, the photometric titrations show a development of absorption bands that is characteristic of DNA-binding ligands, i.e. a hypochromic effect along with a red shift of the absorption band with progressing titration of DNA.30 Nevertheless, the lack of isosbestic points and the appearance of two distinctly different sections of the titration (Fig. 1) point to different binding modes that depend on the LDR. Thus, at the beginning of the titration, i.e. at large LDR values, the ligands form aggregates along the DNA backbone because there are not enough binding sites available. At smaller LDR values, i.e. with ample number of binding sites, the ligands may associate with the DNA by intercalation or groove binding. Notably, the sterically demanding diphenylamino-substituent severely hinders the access of a ligand close to an already occupied DNA binding site, which explains the large amount of DNA required to reach saturation. Unfortunately, this heterogeneous binding of both ligands with duplex and quadruplex DNA, that even changes in the course of the titrations, seriously hampers the determination of meaningful binding constants from the binding isotherms, as the ones calculated only reflect an apparent binding constant, i.e. an average constant from all contributing binding modes, thus showing different overall affinities of the ligands toward a particular DNA form. Hence, the apparent binding constants of ligands 3a and 3b with ct DNA show a higher overall affinity of 3b to this DNA form. The Kb values (3a: Kb = 4.3 × 104 M−1, 3b: Kb = 8.8 × 104 M−1) differ only by a factor of ca. 2 and are both comparable to the ones obtained for classical intercalators.30
Additional information about the binding mode was obtained by CD- and LD-spectroscopic studies. Thus, the strong bisignate ICD band in the long-wavelength absorption range of compound 3a at high LDR clearly confirms the aggregation of the dye at the DNA backbone (Fig. 3, A1).31 At lower LDR, a less intense negative ICD band appears, which indicates a different binding mode. But it should be noted that the latter band is still overlapped by the ICD signal of the aggregate, resulting in a combination of spectra that cannot be dissected. Similarly, the CD bands in the absorption range of DNA and the ligand (<320 nm) also severely overlap. As a result, this combination of overlapping bands, whose contribution also changes with LDR, does not allow a conclusive binding mode analysis at low LDR. At the same time, the negative LD signal in the absorption range of 3a (Fig. 3, B1) clearly indicates an intercalative binding mode of this ligand with ct DNA.32
In the case of ligand 3b, the very intense bisignate CD signal between 400 nm and 600 nm is maintained at all employed LDR ratios, and in each case the bands correspond to the two absorption maxima observed during photometric titration (Fig. 3 A2 and B2). This observation shows that this CD spectrum is also a combination of separate bands. Notably, the ICD bands of the ligand are very strong, as seen directly from the comparison with the DNA bands, and this is usually observed for groove binders.33 The LD-spectroscopic analysis supports this interpretation, as the positive LD band at the long-wavelength absorption of the ligand unambiguously indicates groove binding.31 The weak positive LD bands at 375 nm and 440 nm seem to contradict the latter interpretation, but it should be noted that the size and shape of the ligand do not allow for a complete accommodation and fit of the whole molecule within the groove, so that at least partially one of the quinolizinium units point outside the groove or even intercalate. In this arrangement, this quinolizinium chromophore is decoupled from the conjugated π system and essentially oriented in the same direction as the DNA bases, thus resulting in a positive LD signal.31 Such bisignate LD spectra have also been observed with bichromophoric bis-aminonaphthalimide-substituted Tröger bases and interpreted similarly.34 It is worth noting, however, that the intensity of CD and LD signals decreased significantly at higher LDR, presumably because at this higher ligand loading there are not sufficient binding sites available in the grooves, thus reducing the relative number of intercalated or groove-bound ligands.
The two ligands 3a and 3b do not carry special substituents that may be involved in specific interactions with the DNA, such as hydrogen bonding. Even the amino group is not available as it is sterically protected and the free lone pair of the amino group is highly delocalized. Therefore, the association of these ligands should be mainly governed by attractive dispersion interactions, such as π stacking and van der Waals interactions, with the binding site and by the thermodynamically favorable counter-ion release from the DNA upon association of the cationic ligand.35 Therefore, the different binding modes of ligands 3a and 3b are mainly based on their different size and shape. Both compounds are structurally flexible containing triphenylamine as a rather hydrophobic unit and the quinolizinium as the DNA-binding fragment. In the case of 3a, the bulky diaryl unit may point outside the binding site or even accommodate partly in the groove when the quinolizinium intercalates into DNA. Obviously, this binding mode is not favoured with 3b as only one of the two quinolizinium units can intercalate and the majority of the molecule would still protrude from the binding site so that it is still exposed to the solvent, thus reducing the role of the hydrophobic effect as a driving force for intercalation.35 As a result, ligand 3b binds to the grooves because in this binding mode this sterically demanding molecule fits better to the binding site as a whole, which may be driven by entropic factors.36
The association of 3a and 3b with G4-DNA 22AG was confirmed with CD spectroscopy. In the presence of 3a and 3b, the CD bands of the quadruplex 22AG change only marginally (Fig. 3, C1 and C3), which indicates the preservation of the quadruplex structure upon complex formation.37 The complex formation is also clearly indicated by the increased melting temperature, ΔTm, of the quadruplex in the presence of ligands 3a and 3b, which indicates significant thermodynamic stabilization of the quadruplex toward unfolding due to ligand association. At the same time, the disappearance of the weak shoulder around 250 nm usually denotes the disappearance of one minor form from the equilibrium of different quadruplex structures because of the stabilization of a basket-type or chair-type quadruplex structure by the ligand.38 In the case of ligand 3a, only a very weak ICD signal in the absorption range of the ligand developed, which is often observed for ligands that bind to the quadruplex by terminal π stacking.39 In contrast, the association of ligand 3b with 22AG leads to the formation of a much more pronounced ICD signal, which is proposed to be a characteristic feature of groove-binding G4-DNA ligands.40
In the absence of DNA, the styrylquinolizinium derivatives 3a and 3b are weakly fluorescent with very low quantum yields in aqueous buffer solution (Table 1) due to radiationless deactivation by conformational relaxation of the excited state (see Discussion above). Upon association with duplex and quadruplex DNA, however, the emission intensity of these ligands increases significantly (Fig. 2). As observed for several DNA-sensitive fluorescent light-up probes,14,15 this effect is most likely the result of the restricted conformational freedom of movement of the ligand within the binding site,41 which is comparable to the light-up effect observed in glycerol.22 Similar fluorescence light-up effects on DNA binding have been observed with annelated quinolizinium derivatives42 and diphenylamino-substituted derivatives of quinolinium, pyridinium and imidazolium.43
Most interesting is the observation that the fluorescence light-up effect of 3b on binding with ct DNA is 4 times stronger than that of 3a (Fig. 4A). Considering that the light-up effect is mainly caused by the restricted conformational flexibility of the ligand in the sterically constrained binding site, and not necessarily with the binding strength, this observation indicates that the groove-bound ligand 3b is accommodated more tightly in its binding site than the intercalated molecule 3a. Conversely, when bound to G4-DNA, the light-up factor of 3a is 17 times larger than that of 3b (Fig. 4B), so that in this case the conformational flexibility of 3a is more suppressed in the DNA binding site.
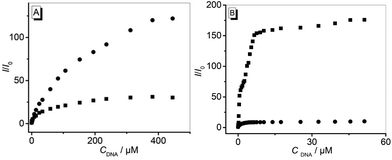 |
| Fig. 4 Plot of relative emission intensity I/I0 of 3a (squares) and 3b (circles) (c = 10 μM) versus concentration of ct DNA in BPE buffer (10 mM, pH 7.0; with 10% v/v DMSO) and 22AG in K-phosphate buffer; pH 7.0, T = at 20 °C. | |
Conclusion
In summary, it was demonstrated that the diphenylaminostyryl-substituted quinolizinium derivatives 3a and 3b bind to duplex and quadruplex DNA. Due to the significantly different size and steric demand of these ligands they exhibit different binding modes. Thus, 3a intercalates into ct DNA and binds through π stacking to G4-DNA 22AG, whereas 3b favours groove binding to both DNA forms. As the very low emission intensity of these compounds increases strongly upon association with DNA and the emission wavelength is close to the NIR range, which is favourable for biological applications, these compounds are promising platforms for the development of DNA-sensitive fluorescent probes. In this regard, the different effects of the actual DNA form and binding mode on the extent of the light-up effect may be used for selective DNA detection.
Conflicts of interest
There are no conflicts to declare.
Acknowledgements
We thank the University of Siegen and the Alexander von Humboldt Foundation (postdoctoral research fellowship for A. K. D.) for financial support. We thank Ms Jennifer Hermann and Ms Sandra Uebach for technical assistance.
Notes and references
-
(a) P. B. Dervan, Molecular recognition of DNA by small molecules, Bioorg. Med. Chem., 2001, 9, 2215–2235 CrossRef CAS;
(b) J. Sheng, J. Gan and Z. Huang, Structure-based DNA-targeting strategies with small molecule ligands for drug discovery, Med. Res. Rev., 2013, 33, 1119–1173 CrossRef CAS;
(c) J. Wang, Survey and summary: From DNA biosensors to gene chips, Nucleic Acids Res., 2000, 28, 3011–3016 CrossRef CAS;
(d) L. H. Hurley, DNA and its associated processes as targets for cancer therapy, Nat. Rev. Cancer, 2002, 2, 188–200 CrossRef CAS;
(e) P. G. Baraldi, A. Bovero, F. Fruttarolo, D. Preti, M. A. Tabrizi, M. G. Pavani and R. Romagnoli, DNA minor groove binders as potential antitumor and antimicrobial agents, Med. Res. Rev., 2004, 24, 475–528 CrossRef CAS.
-
(a) N. C. Seeman and H. F. Sleiman, DNA nanotechnology, Nat. Rev. Mater., 2017, 3, 17068 CrossRef;
(b) Y. Jung and S. J. Lippard, Direct Cellular Responses to Platinum-Induced DNA Damage, Chem. Rev., 2007, 107, 1387–1407 CrossRef CAS;
(c) J. Wu, Y. Zou, C. Li, W. Sicking, I. Piantanida, T. Yi and C. Schmuck, A Molecular Peptide Beacon for the Ratiometric Sensing of Nucleic Acids, J. Am. Chem. Soc., 2012, 134, 1958–1961 CrossRef CAS;
(d) H. Song, J. T. Kaiser and J. K. Barton, Crystal structure of Δ-[Ru(bpy)2dppz]2+ bound to mismatched DNA reveals side-by-side metalloinsertion and intercalation, Nat. Chem., 2012, 4, 615–620 CrossRef CAS.
-
(a) H. Kobayashi, M. Ogawa, R. Alford, P. L. Choyke and Y. Urano, New Strategies for Fluorescent Probe Design in Medical Diagnostic Imaging, Chem. Rev., 2010, 110, 2620–2640 CrossRef CAS;
(b) D.-L. Ma, H.-Z. He, K.-H. Leung, H.-J. Zhong, D. S.-H. Chan and C.-H. Leung, Label-free luminescent oligonucleotide-based probes, Chem. Soc. Rev., 2013, 42, 3427–3440 RSC.
-
(a) Y. V. Suseela, N. Narayanaswamy, S. Pratihar and T. Govindaraju, Far-red fluorescent probes for canonical and non-canonical nucleic acid structures: current progress and future implications, Chem. Soc. Rev., 2018, 47, 1098–1131 RSC;
(b) S. J. Smith, C. R. Nemr and S. O. Kelley, Chemistry-Driven Approaches for Ultrasensitive Nucleic Acid Detection, J. Am. Chem. Soc., 2017, 139, 1020–1028 CrossRef CAS;
(c) S. Manna and S. G. Srivatsan, Fluorescence-based tools to probe G-quadruplexes in cell-free and cellular environments, RSC Adv., 2018, 8, 25673–25694 RSC;
(d) D. Wu, A. C. Sedgwick, T. Gunnlaugsson, E. U. Akkaya, J. Yoon and T. D. James, Fluorescent chemosensors: the past, present and future, Chem. Soc. Rev., 2017, 46, 7105–7123 RSC.
-
(a) P. Prentø, A contribution to the theory of biological staining based on the principles for structural organization of biological macromolecules, Biotech. Histochem., 2001, 76, 137–161 CrossRef;
(b) J. B. LePecq and C. A. Paoletti, A fluorescent complex between ethidium bromide and nucleic acids. Physical-chemical characterization, J. Mol. Biol., 1967, 27, 87–106 CrossRef CAS;
(c) L. S. Lermann, Structural considerations in the interaction of DNA and acridines, J. Mol. Biol., 1961, 3, 18–30 CrossRef;
(d) A. Slama-Schwok, J. Jazwinski, A. Bere, T. Montenay-Garestier, M. Rougée, C. Hélène and J. M. Lehn, Interactions of the Dimethyldiazaperopyrenium Dication with Nucleic Acids. 1. Binding to Nucleic Acid Components and to Single-Stranded Polynucleotides and Photocleavage of Single-Stranded Oligonucleotides, Biochemistry, 1989, 28, 3227–3234 CrossRef CAS.
-
(a) S. Neidle, Quadruplex nucleic acids as targets for anticancer therapeutics, Nat. Rev. Chem., 2017, 1, UNSP 0041 Search PubMed;
(b) D.-L. Ma, Z. Zhang, M. Wang, L. Lu, H. J. Zhong and C.-H. Leung, Recent Developments in G-Quadruplex Probes, Chem. Biol., 2015, 22, 812–828 CrossRef CAS;
(c) S. Neidle, Quadruplex Nucleic Acids as Novel Therapeutic Targets, J. Med. Chem., 2016, 59, 5987–6011 CrossRef CAS.
-
(a) Y. Xu, Chemistry in human telomere biology: structure, function and targeting of telomere DNA/RNA, Chem. Soc. Rev., 2011, 40, 2719–2740 RSC;
(b) A. Ali and S. Bhattacharya, DNA binders in clinical trials and chemotherapy, Bioorg. Med. Chem., 2014, 22, 4506–4521 CrossRef CAS;
(c) P. Murat, Y. Singh and E. Defrancq, Methods for investigating G-quadruplex DNA/ligand interactions, Chem. Soc. Rev., 2011, 40, 5293–5307 RSC;
(d) E. Largy, A. Granzhan, F. Hamon, D. Verga and M. P. Teulade-Fichou, Visualizing the quadruplex: from fluorescent ligands to light-up probes, Top. Curr. Chem., 2013, 330, 111–177 CrossRef CAS.
-
(a) R. W. Sinkeldam, N. J. Greco and Y. Tor, Fluorescent Analogs of Biomolecular Building Blocks: Design, Properties, and Applications, Chem. Rev., 2010, 110, 2579–2619 CrossRef CAS;
(b) T. Deligeorgiev, A. Vasilev, S. Kaloyanova and J. J. Vaquero, Styryl dyes – synthesis and applications during the last 15 years, Color. Technol., 2010, 126, 55–80 CrossRef CAS;
(c) G. R. Rosania, J. W. Lee, L. Ding, H. S. Yoon and Y. T. Chang, Combinatorial Approach to Organelle-Targeted Fluorescent Library Based on the Styryl Scaffold, J. Am. Chem. Soc., 2003, 125, 1130–1131 CrossRef CAS.
-
(a) H. Özhalici-Ünal, C. L. Pow, S. A. Marks, L. D. Jesper, G. L. Silva, N. I. Shank, E. W. Jones, J. M. Burnette, P. B. Berget and B. A. Armitage, A Rainbow of Fluoromodules: A Promiscuous scFv Protein Binds to and Activates a Diverse Set of Fluorogenic Cyanine Dyes, J. Am. Chem. Soc., 2008, 130, 12620–12621 CrossRef;
(b) A. Ajayaghosh, E. Arunkumar and J. Daub, A Highly Specific Ca2+-Ion Sensor: Signaling by Exciton Interaction in a Rigid–Flexible–Rigid Bichromophoric “H” Foldamer, Angew. Chem., Int. Ed., 2002, 41, 1766–1769 CrossRef CAS.
-
(a) R. W. Dirks and H. J. Tanke, Styryl Molecules Light-Up RNAs, Chem. Biol., 2006, 13, 559–561 CrossRef CAS;
(b) C. V. Kumar, R. S. Turner and E. H. Asuncion, Groove binding of a styryl cyanine dye to the DNA double helix: the salt effect, J. Photochem. Photobiol., A, 1993, 74, 231–238 CrossRef CAS;
(c) N. Akbay, M. Y. Losytskyy, V. B. Kovalska, A. O. Balanda and S. M. Yarmoluk, The Mechanism of Benzothiazole Styryl cyanine Dyes Binding with dsDNA: Studies by Spectral-Luminescent Methods, J. Fluoresc., 2008, 18, 139–147 CrossRef CAS;
(d) N. Nizomov, E. N. Kurtaliev, S. N. Nizamov and G. Khodjayev, Spectral-luminescent study of the interaction of some styrylcyanine dyes with bovine serum albumin and DNA in aqueous solutions, J. Mol. Struct., 2009, 936, 199–205 CrossRef CAS.
-
(a) V. B. Kovalska, D. V. Kryvorotenko, A. O. Balanda, M. Y. Losytskyy, V. P. Tokar and S. M. Yarmoluk, Fluorescent homodimer styrylcyanines: synthesis and spectral-luminescent studies in nucleic acids and protein complexes, Dyes Pigm., 2005, 67, 47–54 CrossRef CAS;
(b) J.-S. Lee, Y. K. Kim, M. Vendrel and Y.-T. Chang, Diversity-oriented fluorescence library approach for the discovery of sensors and probes, Mol. BioSyst., 2009, 5, 411–421 RSC;
(c) D. V. Berdnikova, O. A. Fedorova, E. V. Tulyakova, H. Li, S. Kölsch and H. Ihmels, Interaction of Crown Ether-Annelated Styryl Dyes with Double-Stranded DNA, Photochem. Photobiol., 2015, 91, 723–731 CrossRef CAS;
(d) A. Mazzoli, B. Carlotti, G. Consiglio, C. G. Fortuna, G. Miolo and A. Spalletti, Photobehaviour of methyl-pyridinium and quinolinium iodide derivatives, free and complexed with DNA. A case of bisintercalation, Photochem. Photobiol. Sci., 2014, 13, 939–950 RSC;
(e) M.-Q. Wang, J. Xu, L. Zhang, Y. Liao, H. Wei, Y.-Y. Yin, Q. Liu and Y. Zhang, Tuning the selectivity of N-alkylated styrylquinolinium dyes for sensing of G-quadruplex DNA, Bioorg. Med. Chem., 2019, 27, 552–559 CrossRef CAS.
-
(a) Q. Li, Y. Kim, J. Namm, A. Kulkarni, G. R. Rosania, Y. H. Ahn and Y. T. Chang, RNA-selective, live cell imaging probes for studying nuclear structure and function, Chem. Biol., 2006, 13, 615–623 CrossRef CAS;
(b) M. Q. Wang, S. Liu, C. P. Tang, A. Raza, S. Li, L. X. Gao, J. Sun and S. P. Guo, Flexible amine-functionalized triphenylamine derivative as a fluorescent “light-up” probe for G-quadruplex DNA, Dyes Pigm., 2017, 136, 78–84 CrossRef CAS;
(c) A. Manna and S. Chakravorti, Modification of a Styryl Dye Binding Mode with Calf Thymus DNA in Vesicular Medium: From Minor Groove to Intercalative, J. Phys. Chem. B, 2012, 116, 5226–5233 CrossRef CAS;
(d) Z.-Q. Liu, S.-T. Zhuo, J.-H. Tan, T.-M. Ou, D. Li, L.-Q. Gu and Z.-S. Huang, Facile syntheses of disubstituted bis(vinylquinolinium)benzene derivatives as G-quadruplex DNA binders, Tetrahedron, 2013, 69, 4922–4932 CrossRef CAS;
(e) Y.-J. Lu, D.-P. Hu, K. Zhang, W.-L. Wong and C.-F. Chow, New pyridinium-based fluorescent dyes: A comparison of symmetry and side-group effects on G-Quadruplex DNA binding selectivity and application in live cell imaging, Biosens. Bioelectron., 2016, 81, 373–381 CrossRef CAS.
- A. Granzhan and H. Ihmels, Playing Around with the Size and Shape of Quinolizinium-Derivatives: Versatile Ligands for Duplex, Triplex, Quadruplex and Abasic Site-Containing DNA, Synlett, 2016, 27, 1775–1793 CrossRef CAS.
- A. Granzhan, H. Ihmels and M. Tian, The benzo[b]quinolizinium ion as a water-soluble platform for the fluorimetric detection of biologically relevant analytes, ARKIVOC, 2015, vi, 494 Search PubMed.
-
(a) L. Chang, C. Liu, S. He, Y. Lu, S. Zhang, L. Zhao and X. Zeng, Novel styryldehydropyridocolinium derivative as turn-on fluorescent probe for DNA detection, Sens. Actuators, B, 2014, 202, 483–488 CrossRef CAS;
(b) H. Yao, L. Chang, C. Liu, X. Jiao, S. He, H. Liu and X. Zeng, A Novel Styryldehydropyridocolinium Homodimer: Synthesis and Fluorescence Properties Upon Interaction with DNA, J. Fluoresc., 2015, 25, 1637–1643 CrossRef CAS;
(c) E. Zacharioudakis, T. Cañeque, R. Custodio, S. Müller, A. M. Cuadro, J. J. Vaquero and R. Rodriguez, Quinolizinium as a new fluorescent lysosomotropic probe, Bioorg. Med. Chem. Lett., 2017, 27, 203–207 CrossRef CAS;
(d) M. A. Martín, B. del Castillo, J. Ezquerra and J. Alvarez-Builla, Quinolizinium Salts as Fluorescent Probes for N-Nucleophiles, Anal. Chim. Acta, 1985, 170, 89–94 CrossRef;
(e) P. Martin, M. A. Martin, B. del Castillo and I. Cayre, Polarity effect on fluorescence of styryl derivatives of quinolizinium salts in micellar media, Anal. Chim. Acta, 1988, 205, 129–137 CrossRef CAS.
-
(a) Z. J. Ning and H. Tian, Triarylamine: a promising core unit for efficient photovoltaic materials, Chem. Commun., 2009, 37, 5483–5495 RSC;
(b) R. Chennoufi, H. Bougherara, N. Gagey-Eilstein, B. Dumat, E. Henry, F. Subra, S. Bury-Moné, F. Mahuteau-Betzer, P. Tauc, M. P. Teulade-Fichou and E. Deprez, Mitochondria-targeted Triphenylamine Derivatives Activatable by Two-Photon Excitation for Triggering and Imaging Cell Apoptosis, Sci. Rep., 2016, 6, 21458 CrossRef CAS;
(c) M. Q. Wang, W.-X. Zhu, Z.-Z. Song, S. Li and Y.-Z. Zhang, A triphenylamine-based colorimetric and fluorescent probe with donor–bridge–acceptor structure for detection of G-quadruplex DNA, Bioorg. Med. Chem. Lett., 2015, 25, 5672–5676 CrossRef CAS.
-
(a) R. S. Juang, H. W. Wen, M. T. Chen and P. C. Yang, Enhanced sensing ability of fluorescent chemosensors with triphenylamine-functionalized conjugated polyfluorene, Sens. Actuators, B, 2016, 231, 399–411 CrossRef CAS;
(b) S. Koersten and G. J. Mohr, Star-Shaped Tripodal Chemosensors for the Detection of Aliphatic Amines, Chem. – Eur. J., 2011, 17, 969–975 CrossRef CAS;
(c) T. Liu, F. Huo, C. Yin, J. Li, J. Chao and Y. Zhang, A triphenylamine as a fluorophore and maleimide as a bonding group selective turn-on fluorescent imaging probe for thiols, Dyes Pigm., 2016, 128, 209–214 CrossRef CAS;
(d) M. Q. Wang, L. X. Gao, Y. F. Yang, X. N. Xiong, Z. Y. Zheng, S. Li, Y. Wu and L. L. Ma, A triphenylamine derivative as a naked-eye and light-up fluorescent probe for G-quadruplex DNA, Tetrahedron Lett., 2016, 57, 5042–5046 CrossRef CAS;
(e) I. Pont, J. González-García, M. Inclán, M. Reynolds, E. Delgado-Pinar, M. T. Albelda, R. Vilar and E. García-Espaňa, Aza-Macrocyclic Triphenylamine Ligands for G-Quadruplex Recognition, Chem. – Eur. J., 2018, 24, 10850–10858 CrossRef CAS.
-
L. F. Tietze and U. Beifuss, in Comprehensive Organic Synthesis, ed. B. M. Trost, Pergamon, Oxford, 1991 Search PubMed.
-
(a) A. Richards and T. S. Stevens, Synthesis and properties of dehydropyridocolinium salts, J. Chem. Soc., 1958, 3067–3073 RSC;
(b) O. F. Beumel Jr., W. N. Smith and B. Rybalka, Preparation of 2- and 4-Picolyllithium, Synthesis, 1974, 43–45 CrossRef.
- G. Marcelo, S. Pinto, T. Cañeque, I. F. A. Mariz, A. M. Cuadro, J. J. Vaquero, J. M. G. Martinho and E. M. S. Maçôas, Nonlinear Emission of Quinolizinium-Based Dyes with Application in Fluorescence Lifetime Imaging, J. Phys. Chem. A, 2015, 119, 2351–2362 CrossRef CAS.
- F. Arbeloa, P. R. Ojeda and I. Arbeloa, Flourescence self-quenching of the molecular forms of Rhodamine B in aqueous and ethanolic solutions, J. Lumin., 1989, 44, 105–112 CrossRef.
- M. A. Martin, M. Ballesteros and B. D. Castillo, The influence of solvent polarity and viscosity on fluorescence of quinolizinium salts, Anal. Chim. Acta, 1985, 170, 95–100 CrossRef CAS.
-
(a) M. Shortreed, R. Kopelman, M. Kuhn and B. Hoyland, Fluorescent Fiber-Optic Calcium Sensor for Physiological Measurements, Anal. Chem., 1996, 68, 1414–1418 CrossRef CAS;
(b) W. Lin, L. Yuan, Z. Cao, Y. Feng and L. Long, A Sensitive and Selective Fluorescent Thiol Probe in Water Based on the Conjugate 1,4-Addition of Thiols to α, β-Unsaturated Ketones, Chem. – Eur. J., 2009, 15, 5096–5103 CrossRef CAS.
- F. H. Stootman, D. M. Fisher, A. Rodger and J. R. Aldrich-Wright, Analyst, 2006, 131, 1145 RSC.
- E. M. Rezler, J. Seenisamy, S. Bashyam, M. Y. Kim, E. White, D. Wilson and L. H. Hurley, Telomestatin and Diseleno Sapphyrin Bind Selectively to Two Different Forms of the Human Telomeric G-Quadruplex Structure, J. Am. Chem. Soc., 2005, 127, 9439–9447 CrossRef CAS.
-
(a) O. van den Berg, W. F. Jager and S. J. Picken, 7-Dialkylamino-1-alkylquinolinium Salts: Highly Versatile and Stable Fluorescent Probes, J. Org. Chem., 2006, 71, 2666–2676 CrossRef CAS;
(b) A. Granzhan, H. Ihmels and G. Viola, 9-Donor-Substituted Acridizinium Salts: Versatile Environment-Sensitive Fluorophores for the Detection of Biomacromolecules, J. Am. Chem. Soc., 2007, 129, 1254–1267 CrossRef CAS.
- B. Heyne, Self-assembly of organic dyes in supramolecular aggregates, Photochem. Photobiol. Sci., 2016, 15, 1103–1114 RSC.
-
(a) M. A. Haidekker and E. A. Theodorakis, Molecular rotors—fluorescent biosensors for viscosity and flow, Org. Biomol. Chem., 2007, 5, 1669–1678 RSC;
(b) A. K. Chibisov, G. V. Zakharova and H. Görner, Effects of substituents in the polymethine chain on the photoprocesses in indodicarbocyanine dyes, J. Chem. Soc., Faraday Trans., 1996, 92, 4917–4925 RSC;
(c) M. S. A. Abdel-Mottaleb, R. O. Loutfy and R. Lapouyade, Non-radiative deactivation channels of molecular rotors, J. Photochem. Photobiol., A, 1989, 48, 87–93 CrossRef CAS.
- Y. Hong, J. W. Y. Lam and B. Z. Tang, Aggregation-induced emission, Chem. Soc. Rev., 2011, 40, 5361–5388 RSC.
- W. Sbliwa, G. Matusiak and B. Bachowska, An Overview of the Optical and Electrochemical Methods for Detection of DNA – Drug Interactions, Croat. Chem. Acta, 2006, 79, 513 Search PubMed.
-
(a)
B. Norden, A. Rodger and T. Dafforn, Linear Dichroism and Circular Dichroism, RSC Publishing, Cambridge, 2010 Search PubMed;
(b) T. Šmidlehner, I. Piantanida and G. Pescitelli, Polarization spectroscopy methods in the determination of interactions of small molecules with nucleic acids – tutorial, Beilstein J. Org. Chem., 2018, 14, 84–105 CrossRef.
- B. Norden and T. Kurucsev, Analysing DNA complexes by circular and linear dichroism, J. Mol. Recognit., 1994, 7, 141–156 CrossRef CAS.
-
(a) N. Narayanaswamy, S. Das, P. K. Samanta, K. Banu, G. P. Sharma, N. Mondal, S. K. Dhar, S. K. Pati and T. Govindaraju, Sequence-specific recognition of DNA minor groove by an NIR-fluorescence switch-on probe and its potential applications, Nucleic Acids Res., 2015, 43, 8651–8663 CrossRef CAS;
(b) X. B. Fu, D. D. Liu, Y. Lin, W. Hu, Z. W. Mao and X. Y. Le, Water-soluble DNA minor groove binders as potential chemotherapeutic agents: synthesis, characterization, DNA binding and cleavage, antioxidation, cytotoxicity and HSA interactions, Dalton Trans., 2014, 43, 8721–8737 RSC.
- S. Murphy, S. A. Bright, F. E. Poynton, T. McCabe, J. A. Kitchen, E. B. Veale, D. C. Williams and T. Gunnlaugsson, Synthesis, photophysical and cytotoxicity evaluations of DNA targeting agents based on 3-amino-1,8-naphthalimide derived Tröger's bases, Org. Biomol. Chem., 2014, 12, 6610–6623 RSC.
-
(a)
H. Ihmels and L. Thomas, in Materials Science of DNA Chemistry, ed. J. I. Jin, CRC Press, Boca Raton, 2011, p. 49 Search PubMed;
(b)
Y. Xie, V. K. Tam and Y. Tor, in The chemical biology of nucleic acids, ed. G. Mayer, John Wiley & Sons, Chichester, 2010, p. 115 Search PubMed;
(c)
I. Haq, in Nucleic acids in chemistry and biology, ed. G. M. Blackburn, M. J. Gait, D. Loakes and D. M. Williams, Royal Society of Chemistry, Cambridge, 2006, p. 341 Search PubMed.
-
(a) S. Neidle, DNA minor-groove recognition by small molecules, Nat. Prod. Rep., 2001, 18, 291–309 RSC;
(b) Y. Liu, A. Kumar, S. Depauw, R. Nhili, M. H. David-Cordonnier, M. P. Lee, M. A. Ismail, A. A. Farahat, M. Say, S. Chackal-Catoen, A. Batista-Parra, S. Neidle, D. W. Boykin and W. D. Wilson, Water-Mediated Binding of Agents that Target the DNA Minor Groove, J. Am. Chem. Soc., 2011, 133, 10171–10183 CrossRef CAS.
-
(a) A. Tawani, S. K. Mishra and A. Kumar, Structural insight for the recognition of G-quadruplex structure at human c-myc promoter sequence by flavonoid Quercetin, Sci. Rep., 2017, 7, 3600 CrossRef;
(b) N. Ranjan, K. F. Andreasen, S. Kumar, D. H. Volpe and D. P. Arya, Aminoglycoside binding to Oxytricha nova telomeric DNA, Biochemistry, 2010, 49, 9891–9903 CrossRef CAS.
-
(a) I. Manet, F. Manoli, B. Zambelli, G. Andreano, A. Masi, L. Cellai and S. Monti, Affinity of the anthracycline antitumor drugs Doxorubicin and Sabarubicin for human telomeric G-quadruplex structures, Phys. Chem. Chem. Phys., 2011, 13, 540–551 RSC;
(b) W.-B. Wu, S.-H. Chen, J.-Q. Hou, J.-H. Tan, T.-M. Ou, S.-L. Huang, D. Li, L.-Q. Gu and Z.-S. Huang, Disubstituted 2-phenyl-benzopyranopyrimidine derivatives as a new type of highly selective ligands for telomeric G-quadruplex DNA, Org. Biomol. Chem., 2011, 9, 2975–2986 RSC;
(c) Y. J. Lu, T. M. Ou, J. H. Tan, J. Q. Hou, W. Y. Shao, D. Peng, N. Sun, X. D. Wang, W. B. Wu, X. Z. Bu, Z. S. Huang, D. L. Ma, K. Y. Wong and L. Q. Gu, 5-N-Methylated Quindoline Derivatives as Telomeric G-Quadruplex Stabilizing Ligands: Effects of 5-N Positive Charge on Quadruplex Binding Affinity and Cell Proliferation, J. Med. Chem., 2008, 51, 6381–6392 CrossRef CAS.
-
(a) H. Sun, Y. Tang, J. Xiang, G. Xu, Y. Zhang, H. Zhang and L. Xu, Spectroscopic studies of the interaction between quercetin and G-quadruplex DNA, Bioorg. Med. Chem. Lett., 2006, 16, 3586–3589 CrossRef CAS;
(b) T. Yamashita, T. Uno and Y. Ishikawa, Stabilization of guanine quadruplex DNA by the binding of porphyrins with cationic side arms, Bioorg. Med. Chem., 2005, 13, 2423–2430 CrossRef CAS.
- A. K. Jain and S. Bhattacharya, Interaction of G-Quadruplexes with Nonintercalating Duplex-DNA Minor Groove Binding Ligands, Bioconjugate Chem., 2011, 22, 2355–2368 CrossRef CAS.
- R. N. Dsouza, U. Pischel and W. M. Nau, Fluorescent Dyes and Their Supramolecular Host/Guest Complexes with Macrocycles in Aqueous Solution, Chem. Rev., 2011, 111, 7941–7980 CrossRef CAS.
-
(a) K. Faulhaber, A. Granzhan, H. Ihmels, D. Otto, L. Thomas and S. Wells, Studies of the fluorescence light-up effect of amino-substituted benzo[b]quinolizinium derivatives in the presence of biomacromolecules, Photochem. Photobiol. Sci., 2011, 10, 1535–1545 RSC;
(b) R. Bortolozzi, H. Ihmels, L. Thomas, M. Tian and G. Viola, 9-(4 Dimethylaminophenyl) benzo[b]quinolizinium: A Near-Infrared Fluorophore for the Multicolor Analysis of Proteins and Nucleic Acids in Living Cells, Chem. – Eur. J., 2013, 19, 8736–8741 CrossRef CAS.
-
(a) T. Cañeque, A. M. Cuadro, J. Alvarez-Builla, J. Pérez-Moreno, K. Clays, O. Castaño, J. L. Andrés and J. J. Vaquero, Novel charged NLO chromophores based on quinolizinium acceptor units, Dyes Pigm., 2014, 101, 116–121 CrossRef;
(b) B. Dumat, G. Bordeau, E. Faurel-Paul, F. Mahuteau-Betzer, N. Saettel, G. Metge, C. Fiorini-Debuisschert, F. Charra and M. P. Teulade-Fichou, DNA Switches on the Two-Photon Efficiency of an Ultrabright Triphenylamine Fluorescent Probe Specific of AT Regions, J. Am. Chem. Soc., 2013, 135, 12697–12706 CrossRef CAS;
(c) G. Saielli, G. Scorrano, A. Bagno and A. Wakisaka, Solvation of Tetraalkylammonium Chlorides in Acetonitrile–Water Mixtures: Mass Spectrometry and Molecular Dynamics Simulations, ChemPhysChem, 2005, 6, 1307–1315 CrossRef CAS;
(d) J. Li, K. Guo, J. Shen, W. Yang and M. Yin, A Difunctional Squarylium Indocyanine Dye Distinguishes Dead Cells through Diverse Staining of the Cell Nuclei/Membranes, Small, 2014, 10, 1351–1360 CrossRef CAS.
Footnote |
† Electronic supplementary information (ESI) available: Experimental procedures, additional spectroscopic data, and 1H and 13C NMR spectra of 3a and 3b. See DOI: 10.1039/c9pp00096h |
|
This journal is © The Royal Society of Chemistry and Owner Societies 2019 |