DOI:
10.1039/C8NJ04421J
(Paper)
New J. Chem., 2019,
43, 175-181
Palladium nanoparticles decorated SnO2 wrapped MWCNT nanocomposites as a highly efficient H2O2 electrocatalyst
Received
30th August 2018
, Accepted 9th November 2018
First published on 14th November 2018
Abstract
Owing to the broad application of hydrogen peroxide (H2O2) in various fields, the significance of selective and sensitive detection of H2O2 were adequately realized. Therefore, a lower cost, environmentally friendly, and high catalytic activity sensing platform towards H2O2 needs to be exploited and studied. In this work, carboxyl-functionalized multi-walled carbon nanotubes were clad in tin dioxide (with thickness of ∼10 nm) and decorated with palladium nanoparticles (∼20 nm) via a simple solvothermal method. A H2O2 sensor-based Pd–SnO2@MWCNTs nanocomposite was fabricated and exhibited a wide linear range of 0.01–50 mM with a low detection limit of 2.8 μM and high sensitivity of 46.1 μA mM−1 cm−2 (S/N = 3). The sensor also presented a high anti-interference, good stability, and good performance detecting H2O2 in disinfectant samples. Our synthesis strategy perhaps may provide a novel idea for fabricating other electrochemical sensors.
Introduction
Hydrogen peroxide (H2O2) is found and applied in various fields such as food,1 environmental degradation,2 clinical analysis,3 and fuel cells4 due to its strong oxidizing and reducing properties. As an example, in a biological system H2O2 as an important reactive oxygen species (ROS) produced by most oxidase reactions was employed to reflect the state of health.5–7 Improper concentrations of H2O2 can cause some diseases (such as Parkinson's disease), DNA fragmentation, aging, neurodegeneration, etc.8–11 Therefore, an accurate, convenient, and rapid determination of H2O2 is essential and significant. Considering the available means of detecting H2O2,12–15 an enzyme-free electrochemical method attracts a lot of attention owing to its rapid response, low cost, good selectivity, and stability.16–19
A tin dioxide (SnO2)-based sensor has gained considerable attention due to its cheap, highly porous, abundant, high mechanical and chemical stability, and environmentally friendly properties.20,21 To date, SnO2 as an n-type semiconductor material with a wide band gap has been widely used in various fields such as catalysts, gas sensors, electrode materials, electronic devices,22–26etc. Although it can’t be ignored due to so many advantages, SnO2 also has low conductivity. However, a strategy has been adopted that combines SnO2 with carbon materials and metal nanomaterials to improve its conductivity and catalytic activity.27–29 Among carbon materials, multi-walled carbon nanotubes (MWCNTs) is one of the most popular candidates. MWCNTs have remarkable chemical stability, large specific area, high electrocatalytic activity, and brilliant electrical conductivity due to their unique geometry and amazing features.30,31 Besides, functionalized/modified MWCNTs with chemical groups, organic molecules, etc. have been extensively utilized for electrochemical sensing.32,33 A functionalized MWCNT (such as MWCNT-COOH) can improve stability, solubility, offer active sites, and facilitate chemical interactions.34,35 The surfaces of functionalized MWCNTs have abundant carboxylate groups and surface defects which is beneficial for adsorption of target ions (e.g., F−, Sn4+).
Herein, SnO2 nanocrystals anchored on MWCNTs were synthesized by a solvothermal method and decorated with palladium nanoparticles to further enhance catalytic ability. A sensor based on these nanocomposites was built and showed high sensing performance for enzyme-free electrochemical detection of H2O2 owing to the excellent catalytic behavior and electrochemical properties of palladium.
Experimental sections
Chemical reagents and materials
Multiwalled carbon nanotubes (MWCNT, ≥95%) were purchased from Sigma-Aldrich (Shanghai, China). Palladium chloride (PdCl2, Purity > 99%) was obtained from Shanghai Reagent Factory (Shanghai, China). Sodium fluoride (NaF, ≥98%), and tin(IV) chloride pentahydrate (SnCl4·5H2O, 98%) were obtained from Guangdong Guanghua Technology Co., Ltd (Guangdong, China). Hydrogen peroxide (H2O2, AR grade, 30%) and ascorbic acid (AA, C6H8O6, AR grade) were supplied by Beijing Chemical Works. KBr and polyvinyl pyrrolidone (PVP, K30) were purchased from Tianjin Tianli Chemistry Reagent Co., Ltd (Tianjin, China). A 0.1 M phosphate buffered saline (PBS) was used as the supporting electrolyte. Other chemicals all were of analytical grade and used as received without further purification. Deionized water was used for all experiments.
Apparatus and electrochemical measurements
Scanning electron microscopy (SEM) and energy-dispersive X-ray spectroscopy (EDS) images were taken with a Carl Zeiss (SIGMA VP, Germany) microscope. Transmission electron microscopy (TEM) images were obtained from a Tecnai G2 F20 (FEI, USA) instrument. X-ray powder diffraction (XRD) was carried out on a Bruker D8 Advance (Bruker AXS, Germany) instrument.
Electrochemical measurements were run in a conventional three-electrode electroanalysis system controlled by a CHI 660D electrochemical workstation (Shanghai CH Instrument Co. Ltd, China). An Ag/AgCl (3 M KCl) electrode and a platinum foil was used as the reference and counter electrode, respectively, with a bare or modified glassy carbon electrode (GCE, 1.5 mm in semidiameter) as the working electrode. The experiments were carried out at room temperature (25 ± 2 °C).
Preparation of SnO2@MWCNTs nanocomposites
SnO2 over-layers grown on MWCNTs were synthesized by a solvothermal method. MWCNTs were functionalized by successively refluxing in HNO3 + H2SO4 solution (v/v = 1
:
3), washing with deionized water to pH 7.0, and then drying in an oven at 80 °C. The functionalized MWCNTs (60 mg) were dissolved in distilled water (30 mL) by ultra-sonication for one hour. Then, NaF (0.6 g) and SnCl4·5H2O (1 g) were sequentially added to the MWCNTs suspension with stirring for 30 min at room temperature. Lastly, the mixed solution was transferred to a Teflon-lined stainless steel autoclave and kept 24 h at 180 °C. The final product was collected by centrifugation, washed with deionized water, and dried at 80 °C.
Preparation of Pd–SnO2@MWCNTs nanocomposites
Pd nanoparticles were synthesized in water via a simple water bath method. In the first step, an H2PdCl4 solution (56.4 M) was obtained by dissolving PdCl2 power (50 mg) in 0.05 mL of concentrated HCl using a water bath at 60 °C. The solution was diluted to a volume of 5 mL when the PdCl2 power had disappeared. Secondly, the SnO2@MWCNTs were dispersed in 30 mL distilled water with 40 mg of PVP, and then 0.5 mL KBr (0.2 M) and 0.8 mL AA (0.1 M) was injected with continuous stirring. In the last step, the H2PdCl4 solution (0.3 mL) was added to the homogeneous solution at 85 °C in a water bath and kept for 3.5 h. The final product was obtained and dried in an oven at 60 °C.
Electrode modification
The working electrode was prepared by a typical casting method. A mirror like surface of GCE was obtained by using 1.0 and 0.3 μm Al2O3 paste polished several times, respectively, and washed ultrasonically in ethanol–water (1
:
1, v/v ratio) solution for 5 min. Then, the GCE was rinsed with doubly distilled water and allowed to dry in a stream of nitrogen. 1.0 mg of nanocomposites power was dispersed into chitosan (1 mL, 0.5%) and sonicated for 5 min. A suspension (8 μL) was dropped onto the pre-polished GCE and dried in air at room temperature. The synthesized and modified GCE process is shown in Scheme 1.
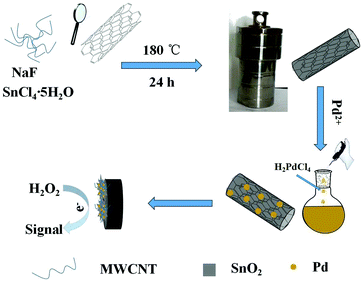 |
| Scheme 1 Schematic illustration of the formation process of Pd–SnO2@MWCNTs and modified GCE. | |
Discussion and results
Characterization of MWCNTs, SnO2@MWCNTs and Pd–SnO2@MWCNTs
The SEM and TEM images of samples are shown in Fig. 1. Some Pd nanoparticles in Fig. 1 are circled by a red dot line. The MWCNTs are exhibited in Fig. 1(A); the surface of MWCNTs was clean with an outer diameter about 25 nm. Fig. 1(B) shows the morphology of SnO2@MWCNTs; the MWCNTs are tightly surrounded by SnO2 and their surfaces are rough. It can be observed from Fig. 1(C) that the state of SnO2 was changed to a loose form and the solubility of nanocomposites improved when Pd nanoparticles were synthesized on SnO2@MWCNTs. The state of Pd–SnO2@MWCNTs was further examined by TEM. It can be observed from the pictures Fig. 1(D–F) that the MWCNTs were enclosed by a SnO2 over-layer with a thickness of ∼10 nm and the Pd nanoparticles dispersed with a size of about ∼20 nm.
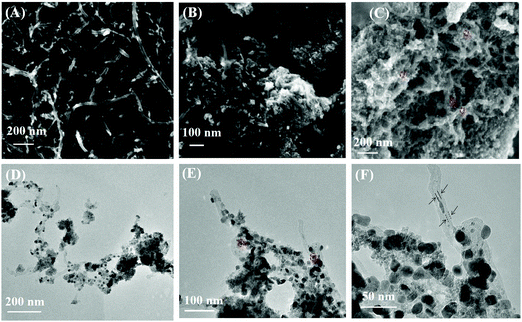 |
| Fig. 1 SEM images of MWCNTs (A), SnO2@MWCNTs (B) and Pd–SnO2@MWCNTs (C), TEM images of Pd–SnO2@MWCNTs (D–F) at low and high resolution. | |
Fig. 2(A) and (B) show the EDS spectrum and elemental mapping images of Pd–SnO2@MWCNTs, which confirmed the existence and distribution of C, O, Sn, and Pd elements in the nanocomposites. The crystalline nature of the prepared nanocomposite was researched using XRD patterns. The patterns of Pd–SnO2@MWCNTs, SnO2@MWCNTs, and MWCNTs were compared in Fig. 2(C). The pattern of MWCNTs has two peaks around 26° and 44° which correspond to the (002) and (101) reflections of hexagonal graphite, respectively.36 The diffraction peaks of the SnO2@MWCNTs nanocomposites were consistent with bare SnO2 (JCPDS no. 41-1445).37 The main intense peak of SnO2(110) phase around 26° overlaps with the peak originating from the MWCNT(002) phase. The other peaks at about 2θ angles of 34, 38.3, 52.0, 54.6, 61.9, 66.8, and 78.7° can be indexed to (101), (200), (211), (220), (310), (301), and (321) reflections. Diminished peaks belonging to MWCNTs, imply a high content of SnO2 in the nanocomposites. Diffraction peaks of Pd nanoparticles at 2θ values of 38.6°, 47.8°, and 66.8° correspond to the crystallographic planes (111), (200), and (220), respectively (JCPDS 46-1043).38 It can be observed that some characteristic diffraction peaks of SnO2 were reduced and disappeared. This may be due to the low wt% of SnO2 in the nanocomposites and SnO2 being present in the amorphous form. To sum up, analytical results suggested that the Pd–SnO2@MWCNTs nanocomposites were successfully synthesized.
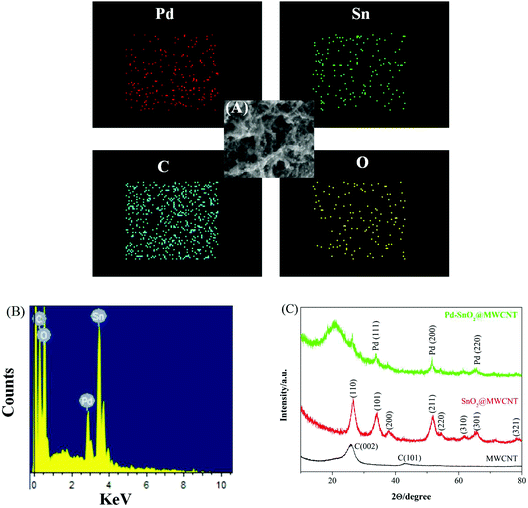 |
| Fig. 2 (A) Elemental mapping of C, O, Pd, and Sn in nanocomposites. (B) EDS spectrum of Pd–SnO2@MWCNTs nanocomposites. (C) XRD patterns of Pd–SnO2@MWCNTs, SnO2@MWCNTs, and MWCNTs. | |
Electrochemical properties of modified GCEs
Influence of the pH value (6.0, 6.5, 7.0, 7.2, 7.5, and 8.0) on the reduction peak current density of H2O2 (3.0 mM) was investigated and is shown in Fig. 3(A). In the present study, bare GCE and modified GCEs were utilized as the working electrode and thus a geometric electrode area of 0.07 cm2 was used to calculate the current densities. It can be observed that the maximum response current density was obtained at pH 7.2. Hence, the pH value of 7.2 was chosen as the support electrolyte for H2O2 sensing. The performances of different modified GCEs detection of H2O2 in 0.1 M PBS were examined by cyclic voltammetry (CV) and are shown in Fig. 3(B) and (C). Comparing the CV curves (CVs) in Fig. 3(B), the MWCNTs/GCE and Pd/GCE have higher current densities than bare GCE, but both modified electrodes display no obvious reduction peak. However, the MWCNTs and Pd nanoparticles would be beneficial to improve the electroconductibility and catalytic activity of the nanocomposites. In Fig. 3(C), the reduction current density of H2O2 was further improved with the modified GCE of SnO2@MWCNTs/GCE and Pd–SnO2@MWCNTs/GCE for sensing H2O2 (3 mM) in 0.1 M PBS. Among the modified GCEs, the CVs of Pd–SnO2@MWCNTs/GCE reached the highest reduction peak current density and has a remarkable reduction peak perhaps due to the good catalytic ability and electrical conductivity of the Pd nanoparticles.
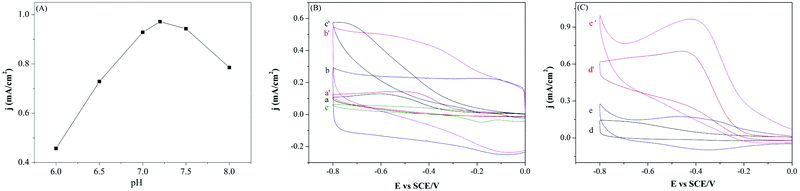 |
| Fig. 3 (A) effect of pH value of 0.1 M PBS on the reduction peak current densities of H2O2 (3.0 mM), (B) CVs of bare GCE (a, a′), MWCNTs/GCE (b, b′) and Pd/GCE (c, c′) as well as (C) CVs of SnO2@MWCNTs/GCE (d, d′), Pd–SnO2@MWCNTs/GCE (e, e′) in absence (a–e) and presence (a′–e′) of 3.0 mM H2O2 in N2-saturated 0.1 M PBS (pH 7.2) at a scan rate of 100 mV s−1. | |
The electrocatalytic behavior of Pd–SnO2@MWCNTs/GCE was further examined by sensing various concentrations of H2O2 in N2-saturated 0.1 M PBS as shown in Fig. 4(A). It can be seen that the reduction current densities were equably increased with the increased concentration of H2O2, indicating Pd–SnO2@MWCNTs has good electrocatalytic activity toward H2O2. A good linear relationship was found between the reduction current densities and H2O2 concentration (from 1 mM to 6 mM) with a correlation coefficient of 0.9999 as shown in Fig. 4(B). As shown in Fig. 4(C), the effect of scan rate was also evaluated to analyze catalytic behavior of the Pd–SnO2@MWCNTs. It was concluded that the reduction peak in current density increased with the increase of scan rate from 20 to 180 mV s−1. A good linear relationship exists between reduction peak current densities and square root of the scan rate with a correlation coefficient of 0.9987, implying that the catalysis process is diffusion controlled.
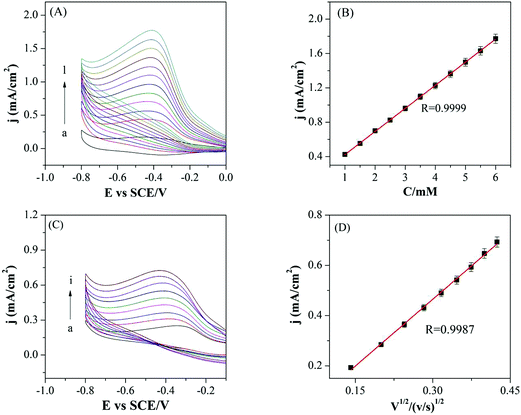 |
| Fig. 4 (A) CVs of Pd–SnO2@MWCNTs/GCE in N2-saturated 0.1 M PBS (pH 7.2) in the absence and presence of H2O2 with various concentrations (from a to l: 0, 1.0, 1.5, 2, 2.5, 3, 3.5, 4, 4.5, 5, 5.5, and 6.0 mM) at a scan rate of 100 mV s−1. (B) Calibration curve of H2O2 reduction current densities versus its concentrations. (C) CVs of Pd–SnO2@MWCNTs/GCE in N2-saturated 0.1 M PBS (pH 7.2) containing 2.0 mM H2O2 at different scan rates (from a to i: 20, 40, 60, 80, 100, 120, 140, 160, and 180 mV s−1). (D) Plot of reduction current densities versus square root of scan rate. | |
According to the relevant literature, the electrocatalytic mechanism of H2O2 could be expressed as follows:39,40
| Pd–OH + H2O2ads ↔ Pd–OH–H2O2ads | (2) |
| Pd–OH–H2O2ads + Pd–OH–H2O2ads → 2Pd–O + 3H2O + ½O2 | (3) |
| Pd–O + H2O + 2e− → Pd + 2OH− | (4) |
The oxidized and reduced states of palladium could transfer rapidly, and thereby decrease the adherence of oxidized ascorbic acid or uric acid on the electrode surface.
Fig. 5(A) shows amperometric response of Pd–SnO2@MWCNTs nanocomposites modified GCE in N2-saturated 0.1 M PBS (pH 7.2) for various concentrations of H2O2. The working potential can ensure high catalytic ability, less interference, and the reduction peak current density reached a stable value within 3 s. The calibration curve of the modified GCE is shown in Fig. 5(B). The linear regression equation was Ip (μA) = 1.836 + 3.225C (mM) with a correlation coefficient of 0.9998 (from 10 μM to 50 mM). The low limit of detection (LOD) was calculated by the equation of LOD = 3SB/b at a signal-to-noise ratio of 3, where SB is the standard deviation of the blank solution and b is the slope of the calibration curve. The LOD and sensitivity for this non-enzymatic H2O2 sensor were estimated from calculation to be 2.8 μM and 46.1 μA mM−1 cm−2, respectively. A comparison of the electrochemical parameter with other H2O2 sensors is shown in Table 1. As illustrated in Table 1, the sensor Pd–SnO2@MWCNTs/GCE showed a wider linear range and lower detection limit. These good performances may be due to the good electrocatalytic behavior of Pd–SnO2@MWCNT nanocomposites towards sensing H2O2.
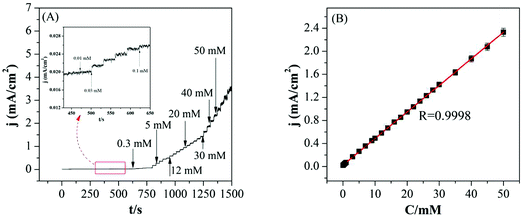 |
| Fig. 5 (A) Typical amperometric response curve of the Pd–SnO2@MWCNTs/GCE for successive injections of H2O2 into a constantly stirred N2-saturated 0.1 M PBS (pH 7.2) at a −0.2 V work potential. (B) Calibration curve of H2O2versus its concentration. | |
Table 1 Comparison of the electrocatalytic parameters with other H2O2 sensors
Sensors |
Linear range (mM) |
Sensitivity |
Detection limit (μM) |
Ref. |
Octahedral Cu2O/Nafion/GCE |
0.01–4.9 |
87 μA mM−1 cm−2 |
6.4 |
41
|
Copper nanoclusters |
0.01–1.0 |
— |
10.0 |
42
|
Cu2O–rGOpa |
0.03–12.8 |
20.0 μA mM−1 cm−1 |
21.7 |
43
|
Graphene–MWCNT/GC |
0.02–2.1 |
15 μA mM−1 cm−2 |
9.4 |
44
|
Ag nanowire array |
0.1–3.1 |
26.6 μA mM−1 cm−2 |
29.2 |
45
|
Pd–SnO2@MWCNTs/GCE |
0.01–50 |
46.1 μA mM−1 cm−2 |
2.8 |
This work |
Interference study
As shown in Fig. 6, the anti-interference ability of Pd–SnO2@MWCNTs/GCE was also examined. Ascorbic acid (AA), glucose (glu), dopamine (DA), and uric acid (UA) were studied as interfering substances. A large response appeared when 0.2 mM H2O2 was injected while almost no change occurred when 0.1 mM AA, glu, DA, or UA was added. These results indicated that Pd–SnO2@MWCNTs/GCE has a good selectivity to H2O2.
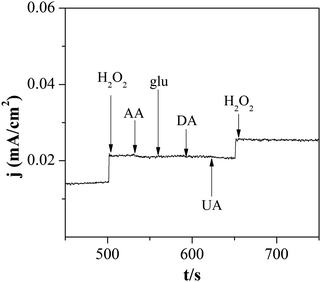 |
| Fig. 6 Amperometric responses of Pd–SnO2@MWCNTs/GCE after injecting 0.2 mM H2O2, 0.1 mM AA, glu, DA, and UA in N2-saturated 0.1 M PBS. | |
Stability and repeatability
The stability and repeatability of Pd–SnO2@MWCNTs/GCE was also assessed as exhibited in Fig. 7. Stability of the modified GCE was investigated in 0.1 M PBS containing 0.2 mM H2O2 up to 1400 s. As shown in Fig. 7(A), the current density remained stable during the experiment which proved a good stability of Pd–SnO2@MWCNTs/GCE. Furthermore, the current densities of five modified electrodes toward 3.0 mM H2O2 were recorded in Fig. 7(B). The RSD for reduction peak current densities sensing H2O2 was less than 3% for five modified GCEs. The peak current density of Pd–SnO2@MWCNTs/GCE kept 96% of its initial current density response after five weeks. Therefore, the Pd–SnO2@MWCNTs/GCE has demonstrated repeatability and stability.
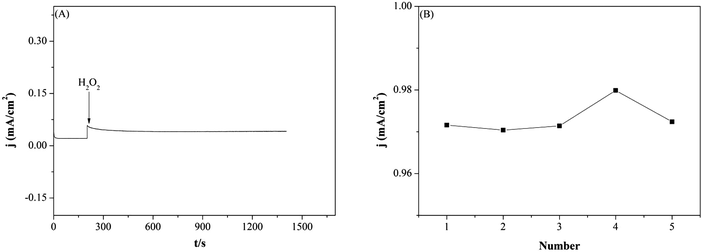 |
| Fig. 7 (A) Amperometric responses of Pd–SnO2@MWCNTs/GCE in N2-saturated 0.1 M PBS with stirring. (B) Current densities of 3.0 mM H2O2 at five equally constructed Pd–SnO2@MWCNTs/GCE under the same conditions. | |
Real samples analysis
The ability to detect H2O2 in real samples was verified by a standard addition method. Diluted H2O2 disinfectant was used as real samples and the analytical results are shown in Table 2. Recoveries in this experiment reached 98.8% and RSDs within 2.3% were calculated. The data suggests that the sensor fabricated in this study could be used for real sample analysis.
Table 2 Results of determination of H2O2 in real samples
Sample number (no.) |
Added (μM) |
Found (μM) |
Recovery (%) |
RSD (%) |
RSD (%) calculated from six measurements. |
Disinfectant sample |
0 |
14.5 ± 0.03 |
— |
— |
No. 1 |
20 |
33.6 ± 0.02 |
97.4 |
2.3 |
No. 2 |
30 |
44 ± 0.05 |
98.8 |
1.7 |
No. 3 |
40 |
53.8 ± 0.02 |
98.7 |
1.5 |
Conclusions
To sum up, a novel Pd–SnO2@MWCNT nanocomposite was successfully synthesized and an electrochemical sensor for H2O2 based on this nanomaterial was fabricated. The sensor exhibited good catalytic ability with H2O2, including a low detection limit, wide linear range, fast response, and good selectivity. Considering the excellent properties and facile generation route of Pd–SnO2@MWCNT nanocomposites, this study may provide a new concept and nanomaterials with which to construct future electrochemical sensors.
Conflicts of interest
There are no conflicts to declare.
Acknowledgements
The authors gratefully acknowledge the financial support of this project by the National Science Foundation of China (No. 21575113), Specialized Research Fund for the Doctoral Program of Higher Education (No. 20126101110013), Northwest University Graduate Innovation and Creativity Funds (No. YZZ17125), the Natural Science Foundation of Shaanxi Province in China (2013KJXX-25, 2018JQ2029), and the Scientific Research Foundation of Shaanxi Provincial Key Laboratory (No. 15JS100, 16JS099).
References
- M. E. Abbas, W. Luo, L. H. Zhu, J. Zou and H. Q. Tang, Food Chem., 2010, 120, 327–331 CrossRef CAS.
- K. A. Al-Maqdi, S. Hisaindee, M. A. Rauf and S. S. Ashraf, Chem. Eng. J., 2018, 352, 450–458 CrossRef CAS.
- R. Shen, P. P. Liu, Y. Q. Zhang, Z. Yu, X. Y. Chen, L. Zhou, B. Q. Nie, A. Żaczek, J. Chen and J. Liu, Anal. Chem., 2018, 90, 4478–4484 CrossRef CAS PubMed.
- L. H. Yi, B. Yu, W. Yi, Y. Q. Zhou, R. Ding and X. Y. Wang, ACS Sustainable Chem. Eng., 2018, 6, 8142–8149 CrossRef CAS.
- X. Li and X. Du, Sens. Actuators, B, 2017, 239, 536–543 CrossRef CAS.
- Y. D. Xue, G. Maduraiveeran, M. Y. Wang, S. L. Zheng, Y. Zhang and W. Jin, Talanta, 2018, 176, 397–405 CrossRef CAS PubMed.
- L. B. Shi, M. Layani, X. Cai, H. L. Zhao, S. Magdassi and M. B. Lan, Sens. Actuators, B, 2018, 256, 938–945 CrossRef CAS.
- S.-J. Kim, P. Cheresh, E. Malsin, R. Jablonski, A. Yeldandi, K. M. Ridge and D. W. Kamp, Am. Thorac. Soc., 2018, A4642 Search PubMed.
- J. Fruehauf and F. Meysken, Clin. Cancer Res., 2007, 13, 789–794 CrossRef CAS PubMed.
- Z. Li, Y. Xin, W. Wu, B. Fu and Z. Zhang, Anal. Chem., 2016, 88, 7724–7729 CrossRef CAS PubMed.
- Y. Wei and M. Guo, Angew. Chem., Int. Ed., 2007, 46, 4722–4725 CrossRef CAS PubMed.
- J. Penga, X. Houb, F. Zenga and S. Wu, Biosens. Bioelectron., 2017, 94, 278–285 CrossRef PubMed.
- Y. Y. Sheng, H. L. Yang, Y. Wang, L. Han, Y. J. Zhao and A. P. Fan, Talanta, 2017, 166, 268–274 CrossRef CAS PubMed.
- M. Guler, V. Turkoglu, A. Bulut and M. Zahmakiran, Electrochim. Acta, 2018, 263, 118–126 CrossRef CAS.
- R. Ravani Ananda, M. Kempegowda and M. Guin, Anal. Chem. Lett., 2017, 7, 779–791 CrossRef CAS.
- K. J. Huang, D. J. Niu, X. Liu, Z. W. Wu, Y. Fan, Y. F. Chang and Y. Y. Wu, Electrochim. Acta, 2011, 56, 2947–2953 CrossRef CAS.
- Y. Su, B. Luo and J. Zhang, Anal. Chem., 2016, 88, 1617–1624 CrossRef CAS PubMed.
- N. Sitnikova, M. Komkova, I. Khomyakova, E. Karyakina and A. Karyakin, Anal. Chem., 2014, 86, 4131–4134 CrossRef CAS PubMed.
- C. Hao, Y. Shen, Z. Wang, X. Wang, F. Feng, C. Ge, Y. Zhao and K. Wang, ACS Sustainable Chem. Eng., 2016, 4, 1069–1077 CrossRef CAS.
- M. H. Jiang, P. Lu, Y. M. Lei, Y. Q. Chai, R. Yuan and Y. Zhuo, Electrochim. Acta, 2018, 271, 464–471 CrossRef CAS.
- S. Navazani, A. Shokuhfar, M. Hassanisadi, M. Askarieh, A. Di Carlo and A. Agresti, Talanta, 2018, 181, 422–430 CrossRef CAS PubMed.
- W. Göpel and K. D. Schierbaum, Sens. Actuators, B, 1995, 26, 1–12 CrossRef.
- E. V. Sokovykh, L. P. Oleksenko, N. P. Maksymovych and I. P. Matushko, Nanoscale Res. Lett., 2017, 12, 383 CrossRef CAS PubMed.
- A. M. Al-Hamdi, U. Rinner and M. Sillanpää, Process Saf. Environ. Prot., 2017, 107, 190–205 CrossRef CAS.
- J. H. Kim, S. W. Cho, D. W. Kang, K. M. Lee, C. Y. Baek, H. M. Lee and C. K. Kim, Sci. Adv. Mater., 2016, 8, 117–121 CrossRef CAS.
- G. Ferraresi, C. Villevieille, I. Czekaj, M. Horisberger, P. Novák and M. El Kazzi, ACS Appl. Mater. Interfaces, 2018, 10, 8712–8720 CrossRef CAS PubMed.
- N. Van Hieu, L. T. B. Thuy and N. D. Chien, Sens. Actuators, B, 2008, 129, 888–895 CrossRef CAS.
- Q. Zhou, L. Xu, A. Umar, W. Chen and R. Kumar, Sens. Actuators, B, 2018, 256, 656–664 CrossRef CAS.
- T. Lu, Y. P. Zhang, H. B. Li, L. K. Pan, Y. L. Li and Z. Sun, Electrochim. Acta, 2010, 55, 4170–4173 CrossRef CAS.
- P. M. Ajayan, Chem. Rev., 1999, 99, 1787–1800 CrossRef CAS PubMed.
- P. J. Britto, K. S. Santhanam, A. Rubio, J. A. Alonso and P. M. Ajayan, Adv. Mater., 1999, 11, 154–157 CrossRef CAS.
- R. Kumar, M. Kumar and K. Awasthi, Int. J. Hydrogen Energy, 2016, 41, 23057–23066 CrossRef CAS.
- Z. A. Alothman, N. Bukhari, S. M. Wabaidur and S. Haider, Sens. Actuators, B, 2010, 146, 314–320 CrossRef CAS.
- Z. Liu, M. Jin, J. Cao, J. Wang, X. Wang and G. Zhou, Sens. Actuators, B, 2018, 257, 1065–1075 CrossRef CAS.
- Y. H. Jin, K. M. Min, S. D. Seo, H. W. Shim and D. W. Kim, J. Phys. Chem. C, 2011, 115, 22062–22067 CrossRef CAS.
- H. Zhang, H. Song, X. Chen, J. Zhou and H. Zhang, Electrochim. Acta, 2012, 59, 160–167 CrossRef CAS.
- Z. Z. Lu and H. K. Wang, CrystEngComm, 2014, 16, 550–555 RSC.
- Z. Xi, D. P. Erdosy, A. Mendoza-Garcia, P. N. Duchesne, J. Li and M. Muzzio, Nano Lett., 2017, 17, 2727–2731 CrossRef CAS PubMed.
- M. Jamal, M. Hasan, A. Mathewson and K. M. Razeeb, J. Electrochem. Soc., 2012, 159, B825–B829 CrossRef CAS.
- D. A. Johnston, M. F. Cardosi and D. H. Vaughan, Electroanalysis, 1995, 7, 520–526 CrossRef CAS.
- Y. Li, Y. Zhong, Y. Zhang, W. Weng and S. Li, Sens. Actuators, B, 2015, 206, 735–743 CrossRef CAS.
- L. Z. Hu, Y. L. Yuan, L. Zhang, J. M. Zhao, S. Majeed and G. B. Xu, Anal. Chim. Acta, 2013, 762, 83–86 CrossRef CAS PubMed.
- F. Xu, M. Deng, G. Li, S. Chen and L. Wang, Electrochim. Acta, 2013, 88, 59–65 CrossRef CAS.
- S. Woo, Y. R. Kim, T. D. Chung, Y. Piao and H. Kim, Electrochim. Acta, 2012, 59, 509–514 CrossRef CAS.
- E. Kurowska, A. Brzózka, M. Jarosz, G. D. Sulka and M. Jaskuł, Electrochim. Acta, 2013, 104, 439–447 CrossRef CAS.
|
This journal is © The Royal Society of Chemistry and the Centre National de la Recherche Scientifique 2019 |