DOI:
10.1039/C8NJ04312D
(Paper)
New J. Chem., 2019,
43, 364-370
Improved catalytic performance of lipase under non-aqueous conditions by entrapment into alkyl-functionalized mesoporous silica†
Received
25th August 2018
, Accepted 19th November 2018
First published on 30th November 2018
Abstract
Three alkyl-functionalized mesoporous silica materials with different lengths of hydrocarbon chains (propyl, octyl and octadecyl) were synthesized by a one-pot condensation approach to enhance the catalytic performance of immobilized lipase r27RCL under non-aqueous conditions. The catalytic performance (esterification activity, thermal stability in n-hexane and organic solvent tolerance) of immobilized r27RCL greatly increased with the enhanced surface hydrophobicity of the mesoporous silica materials, mainly because of lipase activation via hydrophobic interactions between alkyl groups in the mesoporous silica materials and the surface loop (so-called “lid”) of lipase r27RCL. Furthermore, lipase r27RCL immobilized into mesoporous silica materials modified with octadecyl showed remarkably increased activity for the esterification reaction between ethanol and lauric acid (∼101 times higher than that of free lipase) and enantioselectivity in the kinetic resolution of (R, S)-1-phenylethanol by vinyl acetate in hexane (E ≥ 400, with eep ≥ 99%) compared with free r27RCL (E = 3.7, with eep = 56%) under non-aqueous conditions. Lipase r27RCL immobilized into mesoporous silica materials modified with octadecyl could be reused for at least 5 cycles without significant loss of activity and enantioselectivity. Our research suggests that lipase r27RCL immobilized into mesoporous silica materials modified with octadecyl is an attractive biocatalyst for catalysis under non-aqueous conditions.
1. Introduction
Lipases (triacylglycerol ester hydrolases EC 3.1.1.3) are ubiquitous hydrolases known to hydrolyze the ester bonds of water insoluble substrates in aqueous medium. Furthermore, in contrast to many other enzymes, lipases show remarkable levels of activity and stability under non-aqueous conditions, which facilitates the catalysis of reversible reactions (such as esterification and transesterification reactions). Due to these unique properties, lipases are one of the most attractive enzymes for catalysis under non-aqueous conditions.1 Enzymatic reactions under non-aqueous conditions exhibit numerous advantages, such as suppression of water-dependent side reactions, increased solubility of hydrophobic compounds, elimination of microbial contamination and alternation of substrate specificity, chemo-selectivity, stereo-selectivity and regio-selectivity.2,3
Rhizopus lipases are one important resource of industrial lipases, which are applied in the field of the edible oil and fat industry.4 In our previous study, a lipase gene from Rhizopus chinensis (CCTCC M201021) was cloned and expressed in Pichia pastoris as r27RCL.5 However, r27RCL cannot exhibit perfect catalytic performance under non-aqueous conditions as a result of its low activity, low stability and low enantioselectivity under non-aqueous conditions. Enzyme immobilization can overcome these problems.6,7 A range of materials have been applied for enzyme immobilization,6,8–16 and the structure and morphology of supports, such as yolk–shell, core–shell, etc., play an important role in the activity and capacity of the immobilized enzymes. Ordered mesoporous silica has drawn lots of attention owing to its distinct properties for enzyme immobilization, such as ordered mesostructure, high specific surface area and pore volume, and chemical and mechanical stability.17 More importantly, the internal surface of mesoporous silica materials surrounding the immobilized enzymes could be facilely functionalized with various organic groups, offering a great opportunity to impart desired micro-environmental properties to the immobilized enzymes.18,19 Among mesoporous materials used as immobilized enzyme supports, Santa Barbara Amorphous-15 (SBA-15) material is one of the most representative examples, as its mesoporous diameter (5–30 nm) is close to the dimensions of the enzyme molecule, which makes it a perfect candidate for the immobilization of enzymes.20,21 Zhang et al.22 demonstrated that the catalytic performance of immobilized enzymes is influenced by the structure and pore size of the support. A comparative study was carried out to immobilize enzymes between mesoporous molecular sieves MCM-41 and SBA-15 and mesostructured cellular foam (MCF) materials. Compared to other materials, SBA-15 shows the best capacity for enzymes. However, a systematic study on the immobilization of lipase into SBA-15 has not been seen.
In view of the “interfacial activation” of lipase, some studies indicate that supports functionalized with hydrophobic groups for lipase immobilization, such as hydrophobic octyl groups,23 dodecyl groups,24 and stearyl groups,25 can result in an increase in the activity retention of lipase. However, the correlation between the hydrophilicity/hydrophobicity of the support and the performance of the immobilized lipase is not clear. Some studies indicate that the moderate surface hydrophobicity of the support produces higher lipase activity,26,27 while some report that the greater hydrophobic interface of the support is preferable for the elevated activity of the immobilized lipase.22 So, supports with various surface hydrophilicities/hydrophobicities should be prepared to study the correlation between the performance of the immobilized lipase and the hydrophobicity of the support.
In the present work, SBA-15 samples with various hydrophobic/hydrophilic internal surfaces were used to immobilize r27RCL to enhance the activity, stability and enantioselectivity of r27RCL under non-aqueous conditions.
2. Experimental
2.1 Materials
Lipase r27RCL was previously produced in our laboratory (for details, see the ESI†).5 Poly(ethylene glycol)-block-poly(propylene glycol)-block-poly(ethylene glycol) (Pluronic P123, EO20–PO70–EO20, Mw = 5800) and 4-nitrophenyl butyrate (pNPB) were purchased from Sigma-Aldrich. Tetraethoxysilane (TEOS), n-propyltrimethoxysilane (C3), n-octyltrimethoxysilane (C8), octadecyltrimethoxysilane (C18) and other reagents were purchased from Sinopharm Chemical Reagents Co., Ltd.
2.2 Preparation of alkyl-functionalized SBA-15
Mesoporous silica was synthesized according to a previously reported method with a slight modification.28 In a typical preparation, 2.0 g of Pluronic P123 was dissolved in 15 ml of water and 60 ml of 2 M HCl solution with vigorous stirring at 35 °C for 2 h. Then, 4.25 g of TEOS was added dropwise into the mixture with stirring at 35 °C for 20 h. The mixture was aged at 100 °C for 48 h without stirring. Subsequently, the solid product was recovered, washed abundantly with deionized water and air-dried at RT. Thereafter, Pluronic P123 was removed from the products by ethanol extraction for 5 h at 65 °C. This was repeated three times to ensure complete removal of Pluronic P123 and the product was air-dried at RT. The sample was denoted as pure MPS.
Alkyl-functionalized SBA-15 was synthesized according to Wang et al.29 with a slight modification. Pluronic P123 (2.0 g) was dissolved in 15 ml of water and 60 ml of 2 M HCl solution with vigorous stirring at 35 °C for 2 h. Then, 4.25 g of TEOS was added dropwise into the mixture under stirring at 35 °C, followed by the addition of organotrialkoxysilane 1 h later. The alkyl-functionalized SBA-15 was synthesized with organosilane/TEOS at a molar ratio of 1
:
9. After stirring for 20 h, the mixture was aged at 100 °C for 48 h without stirring. Subsequently, the solid product was recovered, washed abundantly with deionized water and air-dried at RT. Thereafter, Pluronic P123 was removed from the products by ethanol extraction for 5 h at 65 °C. This was repeated three times to ensure complete removal of Pluronic P123 and the product was air-dried at RT. The sample was denoted as MPS-R (R = C3, C8, and C18), where R represents the functional group of organosilane.
2.3 r27RCL immobilization
The support solid (15 mg; pure MPS, MPS-C3, MPS-C8 or MPS-C18) was added to 2 ml of phosphate buffer (50 mM, pH 8.0) solution containing the crude r27RCL (1 mg ml−1 protein). The mixture was incubated at 25 °C with a constant rotation of 200 rpm for 10 h. After incubation, the enzyme adsorbed nanoparticles were separated and the amount of enzyme immobilized was calculated using the Bradford assay method. The immobilized lipase was denoted as pure MPS-r27RCL or MPS-R-r27RCL (R = C3, C8, and C18), where R represents the functional group of organosilane. The immobilization yield (IY) and relative activity (RA) were calculated according to the following equations:30 | IY (%) = 100 × the amount of immobilized protein/the content of protein added in the immobilization solution | (1) |
| RA (%) = 100 × activity measured on the support/the total activity of free enzyme | (2) |
2.4 Hydrolytic reaction
The hydrolytic activities of free r27RCL and immobilized r27RCL in aqueous solution were assayed by pNPB as the substrate at 40 °C.31
2.5 Esterification reaction
The esterification activities of free r27RCL and immobilized r27RCL were assayed via an esterification reaction of ethanol and lauric acid. Two milligrams of free r27RCL and immobilized r27RCL was added to a screw capped vial containing a mixture of ethanol (0.72 M) and lauric acid (0.6 M) in 1 ml of dry n-heptane. The vial was placed in a controlled temperature shaker at 40 °C and shaken at 200 rpm. The reaction was stopped after 1 h and analyzed by gas chromatography. The esterification activity of r27RCL was defined as the amount of ester produced (micromole) per minute and milligram protein.
Ethyl laurate can be identified by gas chromatography (Agilent GC 6890) equipped with a flame ionization detector (FID) and a packed column DB-WAX (30 m × 0.25 mm × 0.25 μm). Nitrogen was used as a carrier gas and the temperature of the injector and detector was kept constant at 220 °C. The column was held at 60 °C, kept constant for 6 min and increased up to 200 °C at a rate of 15 °C min−1.
2.6 Transesterification reaction
A transesterification reaction of racemic 1-phenylethanol was selected to determine the transesterification activities of free r27RCL and immobilized r27RCL with a slight modification.32
Racemic 1-phenylethanol (0.25 mmol) and vinyl acetate (0.5 mmol) were dispersed in n-hexane (1 ml) at 40 °C. Immobilized lipase containing an identical protein content was added to the mixture to initiate the reaction. The samples were analyzed using high performance liquid chromatography (HPLC) with a chiral column, Chiralcel OD-H (4.6 mm × 250 mm). Enantioselectivity was expressed as the E value.
As for the reusability, the immobilized r27RCL was recovered by centrifugation after each batch, washed with dry n-hexane and subsequently used for the next cycle.
2.7 Thermal stability
The thermal stabilities of free r27RCL and immobilized r27RCL under non-aqueous conditions were assayed by incubating the enzyme in n-hexane at 70 °C for different time durations. Hexane was removed by centrifugation. The activities of free r27RCL and immobilized r27RCL were measured by pNPB hydrolysis at 40 °C.
2.8 Stability under different non-aqueous conditions
To study the r27RCL stability under non-aqueous conditions, free r27RCL and immobilized r27RCL were incubated with 50% (v/v) methanol, propanol and cyclohexane in 50 mM phosphate buffer at pH 8.0. After incubation for 2 h at room temperature, the activities of free r27RCL and immobilized r27RCL were measured by pNPB hydrolysis at 40 °C.
2.9 Material characterization
Transmission electron microscopy (TEM) images were obtained using a JEOL JEM-2100 at a voltage of 100 kV. Nitrogen adsorption and desorption isotherms were recorded at 77 K using a Micromeritics TriStar II 3020 system. The specific surface area was calculated by using the Brunauer–Emmett–Teller (BET) method based on adsorption isotherms. The pore diameter distribution was calculated from adsorption isotherms by using the Barrett–Joyner–Halenda (BJH) method. Infrared spectra were recorded on a Thermo Nicolet 6700 Fourier transform infrared spectrometer by using KBr pellets. X-ray powder diffraction (XRD) patterns were recorded on a Bruker AXS D8 Advance diffractometer by using Cu Kα radiation. A Waters DSC Q2000 was used for thermogravimetric (TG) analysis in a temperature range of 40–900 °C at a heating rate of 10 °C min−1. The adsorption of water and toluene vapors was measured at 0 °C using a Hiden Intelligent Gravimetric Analyzer IGA-100B after the sample was degassed for 8 h at 80 °C.
3. Results and discussion
3.1 Physicochemical properties of alkyl-functionalized mesoporous silica
There are two approaches to prepare alkyl-functionalized mesoporous silica materials, that is, the grafting approach and the one-pot condensation approach. The advantages of a one-pot condensation approach in the homogeneous distribution of functional groups have been reported by other publications.33,3429Si MAS NMR spectroscopy was performed to evaluate the homogeneous distribution of functional groups.35 Besides, some studies reported that mesoporous silica materials have been functionalized with alkyl groups for lipase immobilization to enhance the catalytic performance of immobilized lipase.34,36,37 Shakeri et al.38 found that the increase from 3 to 18 carbons in the length of alkyl chains incorporated on spherical silica-based mesocellular foam resulted in an increase from 110% to 7300% in the activity retention of lipase. So, in this study, three alkyl-functionalized mesoporous silica materials (MPS-C3, MPS-C8 and MPS-C18) were synthesized by a one-pot condensation approach for enzyme immobilization.
Nitrogen adsorption–desorption analysis was conducted to evaluate their mesoporous features. Fig. S1 (ESI†) and Table 1 show the nitrogen adsorption–desorption isotherms and pore structural parameters for pure MPS, MPS-C3, MPS-C8 and MPS-C18. The results show that all these materials exhibit IV type isotherms and have similar pore sizes of about 6 nm with a narrow size distribution. This value is large enough to accommodate lipase r27RCL (molecular weight of 37 kDa (Fig. S2, ESI†); diameter: about 4–5 nm).
Table 1 Pore structure parameters, organic group contents of supports and molar ratios of adsorbed water/toluene of mesoporous silica materials
Sample |
D
BJH (nm) |
S
BET (m2 g−1) |
V
P (cm3 g−1) |
Content of organic group (mmol g−1) |
Molar ratio of adsorbed water/toluene |
Pure MPS |
7.50 |
608.34 |
0.95 |
— |
1.16 |
MPS-C3 |
6.06 |
761.16 |
0.83 |
1.095 |
0.54 |
MPS-C8 |
6.51 |
648.84 |
0.77 |
0.884 |
0.13 |
MPS-C18 |
5.98 |
652.18 |
0.73 |
1.004 |
0.04 |
The pore structures of the mesoporous silica materials were characterized by using TEM images and XRD patterns. As shown in Fig. S3 (ESI†), the pure MPS material exhibits an XRD pattern with three peaks that can be indexed as the (100), (110) and (200) reflections of a two-dimensional hexagonal P6mm symmetry.27 For the one-pot modified mesoporous silica materials (MPS-3, MPS-8 and MPS-C18), the (110) and (200) reflections are not very apparent. But, in the TEM image shown in Fig. 1, well-ordered 1D mesopores arraying along the long axis, rather similar to pure MPS, could be clearly resolved for the one-pot alkyl-functionalized mesoporous silica materials.
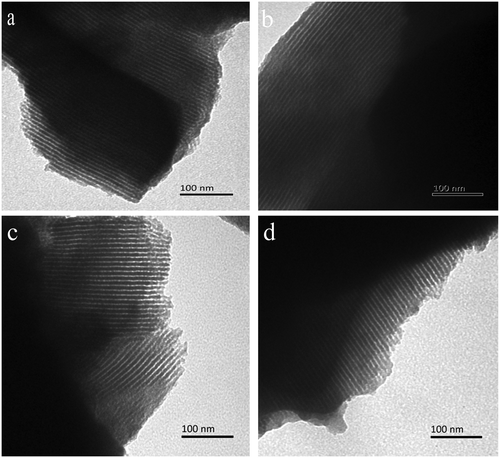 |
| Fig. 1 TEM images of (a) pure MPS, (b) MPS-C3, (c) MPS-C8 and (d) MPS-C18. | |
Fig. 2 displays the FT-IR spectra of the mesoporous silica materials. All the mesoporous silica materials show specific bands at around 1100, 810 and 480 cm−1 attributable to the typical vibration of Si–O–Si in the mesoporous silica materials. The spectra of the alkyl-functionalized mesoporous silica materials show peaks at ∼2880, ∼2885 and 2964 cm−1 attributable to the stretching vibration of C–H in alkyl chains, and the peaks at 1450 and 1500 cm−1 originate from the bending vibration of C–H. The intensity of the peaks at 1450 and 1500 cm−1 (bending vibration of C–H) in various alkyl-functionalized samples is not very apparent in other publications.10,39 The results indicate that the alkyl groups have been introduced into the framework of the mesoporous silica materials by using the one-pot condensation approach.
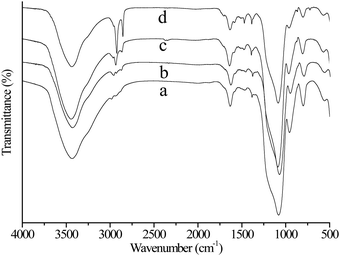 |
| Fig. 2 FT-IR spectra of (a) pure MPS, (b) MPS-C3, (c) MPS-C8 and (d) MPS-C18. | |
The amount of alkyl groups in the alkyl-functionalized mesoporous silica materials was determined by thermogravimetric (TG) analysis. As shown in Table 1, the content of alkyl groups in the alkyl-functionalized mesoporous silica materials was measured to be 1.095, 0.884, and 1.004 mmol g−1 for MPS-C3, MPS-C8 and MPS-C18, indicating that all the alkyl-functionalized mesoporous silica materials using the one-pot condensation approach had similar alkyl group contents, furthermore evidencing the successful incorporation of alkyl groups into the mesoporous silica materials.
The relative hydrophobicity/hydrophilicity of supports was assayed by water vapor and toluene vapor adsorption experiments (Table 1). At a P/P0 of 0.96, the molar ratio of adsorbed water/toluene is 1.16, 0.54, 0.13 and 0.04 for pure MPS, MPS-C3, MPS-C8 and MPS-C18, respectively. The results confirm a significant alteration from hydrophilicity to moderate hydrophobicity and finally strong hydrophobicity. Pure MPS had the highest hydrophilicity while MPS-C18 had the highest hydrophobicity. MPS-C3 and MPS-C8 are within the two boundaries.
3.2 Catalytic activities of immobilized r27RCL under non-aqueous conditions
Fig. S4 (ESI†) shows the spectra of MPS-C18 and MPS-C18-r27RCL. The spectra of MPS-C18-r27RCL exhibit a weak band at 1660 cm−1 assigned to the C
O stretching vibration of amide, which can roughly indicate the presence of enzyme.32 The lipase loading of the mesoporous silica materials is listed in Table S1 (ESI†). The alkyl-functionalization increased the r27RCL loading. Moreover, r27RCL uptake was increased with enhanced surface hydrophobicity of the supports. The difference in r27RCL loading for different alkyl-functionalized mesoporous silica materials can be explained by the difference in the surface hydrophobicity of the supports.27
The esterification reaction between ethanol and lauric acid was conducted to assess the catalytic activity of immobilized r27RCL under non-aqueous conditions. As shown in Fig. 3 and Table S1 (ESI†), all of the immobilized r27RCL samples showed increased catalytic activities, and MPS-C18-r27RCL gave the best performance, with about 102 times the activity obtained with free r27RCL (0.6 μmol min−1 mg−1). The increased activity of immobilized r27RCL can be attributed to the interfacial activation of lipase by the hydrophobic surface.27,34 In addition, the mesoporous silica materials favored the effective dispersion of r27RCL and enabled r27RCL to be more accessible to the substrate, while free r27RCL aggregated in the organic solvent to limit mass transfer and retard the reaction. The results agreed well with several previous reports.40,41 Shakeri et al.38 reported that lipase from Rhizopus oryzae (ROL) was immobilized onto C18-MCF, with 73 times the transesterification activity of free ROL. In addition, hydrolytic reactions of pNPB were conducted. As shown in Fig. S5 and Table S1 (ESI†), the activity of immobilized r27RCL was increased with enhanced surface hydrophobicity of the support, but the activity of immobilized r27RCL was lower than that of free r27RCL. This may be due to the enhanced diffusion restriction caused by the high r27RCL loading.27
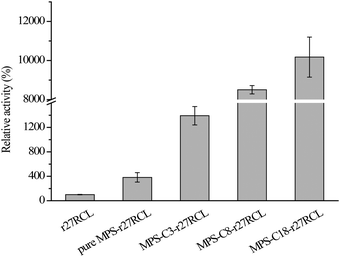 |
| Fig. 3 Comparison of the relative activities of r27RCL immobilized into mesoporous silica materials for the esterification reaction between ethanol and lauric acid, assuming the relative activity of free lipase to be 100%. | |
3.3 Enantioselectivity of immobilized r27RCL
A transesterification reaction of racemic 1-phenylethanol with vinyl acetate was selected as the model reaction to investigate the enantioselectivity of free r27RCL and immobilized r27RCL. As shown in Fig. 4 and Table 2, the conversion and enantiomeric excess of the product (eep) of free r27RCL increased slowly with the reaction time, and they reached about 5.9% and 56% at 5 h, respectively. However, an excellent enantioselectivity (E ≥ 200, with eep ≥ 99%) was obtained for all immobilized r27RCL samples (E = 3.7 for free r27RCL). Especially, MPS-C18-r27RCL showed the highest initial rate and conversion (51%) in comparison with other immobilized r27RCL samples. Some literature studies23,36,42 have also reported this phenomenon. For example, Yang et al.43 reported that diatomite was modified by different functional groups (including methacryloxypropyl, octyl, dodecyl, vinyl and aminopropyl groups) to immobilize lipase KM. All the prepared lipases showed improved activity and enantioselectivity towards HMPC compared with free lipase KM. The improved enantioselectivity of immobilized lipase KM might be due to some distortion in the enzyme conformation, reducing the flexibility of the enzyme generated from the interaction between supports and enzyme during the immobilization process.
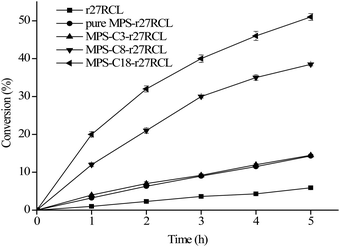 |
| Fig. 4 Resolution process of 1-phenylethanol of r27RCL immobilized into mesoporous silica materials. | |
Table 2 Kinetic resolution of (R, S)-1-phenylethanol catalyzed by r27RCL
Sample |
Conversion (%) |
eep value (%) |
E value |
r27RCL |
5.9 |
56 |
3.7 |
Pure MPS-r27RCL |
14.3 |
99 |
234 |
MPS-C3-r27RCL |
14.5 |
99 |
234.6 |
MPS-C8-r27RCL |
38.5 |
99 |
376.7 |
MPS-C18-r27RCL |
51 |
99 |
>400 |
3.4 Stability of immobilized r27RCL
Most lipase catalyzed reactions are carried out under non-aqueous conditions since many substrates are water insoluble. Hence, evaluating the stability of r27RCL under non-aqueous conditions is important for further reaction design. First, the effects of different organic solvents on enzyme stability were studied, and the results are presented in Table 3. Methanol completely inhibits the activities of both free r27RCL and immobilized r27RCL. This is due to the fact that polar solvents can disrupt the water layers of lipase, leading to the inactivation of enzyme.44 In addition, the stability of immobilized r27RCL in propanol and cyclohexane is higher than that of free r27RCL. This can be explained by the fact that the immobilized r27RCL can avoid water layer stripping and retains its enzyme structure with catalytic activity.45
Table 3 Stability of r27RCL and immobilized r27RCL under non-aqueous conditions
|
Log P |
r27RCL |
Pure MPS-r27RCL |
MPS-C3-r27RCL |
MPS-C8-r27RCL |
MPS-C18-r27RCL |
Relative activitya (%) |
100% activity in water and no activity observed in methanol.
|
Propanol |
0.28 |
11 |
17 |
18 |
24 |
27 |
Cyclohexane |
3.44 |
42 |
56 |
58 |
69 |
74 |
Next, the thermal stability of free r27RCL and immobilized r27RCL was evaluated by determining their residual activities after incubation in n-heptane at 70 °C for different time durations. The results are presented in Fig. 5; all the immobilized r27RCL samples showed improved thermal stability compared with free r27RCL. MPS-C18-r27RCL exhibits the best thermal stability and still maintains around 56% of its initial activity after 20 h incubation in hexane at 70 °C, whereas free r27RCL loses nearly 85% of its initial activity. This can be ascribed to the fact that mesoporous silica materials provide an appropriate microenvironment to prevent lipase molecules from thermal inactivation and increase the activity of lipase due to the surface hydrophobicity of the supports.46
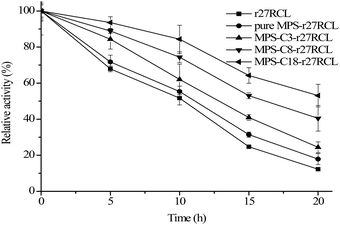 |
| Fig. 5 Thermal stability of free and immobilized r27RCL in n-heptane at 70 °C. | |
The reusability of immobilized enzyme systems is very important for industrial applications. In this study, the operational stability of immobilized r27RCL on MPS-C18 was evaluated in the kinetic resolution of 1-phenylethanol at 40 °C (5 h for each bath). As shown in Fig. 6, MPS-C18-r27RCL can still retain a stable conversion (from 50% to 45.3%) with more than 99% enantioselectivity even after 5 reuse cycles. However, the conversion of MPS-r27RCL presented an apparent decrease from 14.3% to 7.1% after 5 recycles. The reason for the excellent reusability of MPS-C18-r27RCL can be explained by the fact that mesoporous silica materials with narrow pores can inhibit r27RCL leakage and protect the r27RCL from inactivation. In addition, the hydrophobic interaction between r27RCL and supports can stabilize the catalytic conformation of lipase.36
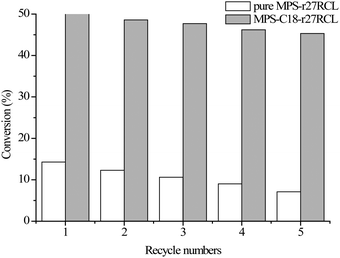 |
| Fig. 6 Reuse of pure MPS-r27RCL and MPS-C18-r27RCL in the kinetic resolution of (R, S)-1-phenylethanol. | |
4. Conclusions
In summary, three alkyl-functionalized mesoporous silica materials (MPS-C3, MPS-C8 and MPS-C18) were synthesized by a one-pot condensation approach to immobilize lipase r27RCL. All the immobilized r27RCL samples showed improved catalytic activity under non-aqueous conditions compared with free r27RCL. MPS-C18-r27RCL exhibited the best performance, with about 102 times the activity obtained with free r27RCL for the esterification reaction between ethanol and lauric acid. Moreover, an excellent enantioselectivity (E ≥ 200, with eep ≥ 99%) was obtained for all immobilized r27RCL samples in the kinetic resolution of (R, S)-1-phenylethanol by vinyl acetate in isooctane (E = 3.7 for free r27RCL). Especially, MPS-C18-r27RCL displayed the highest initial rate and conversion (51%) in comparison with the other immobilized r27RCL samples. The investigation of the intrinsic stability of r27RCL shows that immobilized r27RCL into mesoporous silica materials makes r27RCL more resistant to polar solvents and thermal exposure. MPS-C18-r27RCL could be reused for at least 5 cycles without significant loss of activity and enantioselectivity. Based on these desirable results, MPS-C18-r27RCL is a satisfying biocatalyst for catalysis under non-aqueous conditions.
Conflicts of interest
There are no conflicts to declare.
Acknowledgements
Financial support from NSFC (31671799), the Six Talent Peaks Project in Jiangsu Province (NY-010), the 333 Project in Jiangsu Province (BRA2015316), the National High Technology Research and Development Program of China (863 Program) (2012AA022207), the Postgraduate Research & Practice Innovation Program of Jiangsu Province (KYCX17_1425) and National First-Class Discipline Program of Light Industry Technology and Engineering (LITE2018-09) are greatly appreciated.
References
- X.-W. Yu, L.-L. Wang and Y. Xu, J. Mol. Catal. B: Enzym., 2009, 57, 304–311 CrossRef CAS.
- A. Kumar, K. Dhar, S. S. Kanwar and P. K. Arora, Biol. Proced. Online, 2016, 18, 2 CrossRef PubMed.
- A. Salihu and M. Z. Alam, Process Biochem., 2015, 50, 86–96 CrossRef CAS.
- X. W. Yu, Y. Xu and R. Xiao, Prog. Lipid Res., 2016, 64, 57–68 CrossRef CAS PubMed.
- X.-W. Yu, R. Wang, M. Zhang, Y. Xu and R. Xiao, Microb. Cell Fact., 2012, 11, 102 CrossRef CAS PubMed.
- D. Turati, W. Morais Júnior, C. Terrasan, S. Moreno-Perez, B. Pessela, G. Fernandez-Lorente, J. Guisan and E. Carmona, Molecules, 2017, 22, 339 CrossRef PubMed.
- R. C. Rodrigues, C. Ortiz, Á. Berenguer-Murcia, R. Torres and R. Fernández-Lafuente, Chem. Soc. Rev., 2013, 42, 6290–6307 RSC.
- S. P. de Souza, R. A. D. de Almeida, G. G. Garcia, R. A. C. Leão, J. Bassut, R. O. M. A. de Souza and I. Itabaiana, J. Chem. Technol. Biotechnol., 2018, 93, 105–111 CrossRef CAS.
- A. Gricajeva, S. Kazlauskas, L. Kalediene and V. Bendikiene, Int. J. Biol. Macromol., 2018, 108, 1165–1175 CrossRef CAS PubMed.
- P. Urrutia, R. Arrieta, L. Alvarez, C. Cardenas, M. Mesa and L. Wilson, Int. J. Biol. Macromol., 2018, 108, 674–686 CrossRef CAS PubMed.
- S. K. Patel, V. C. Kalia, J. H. Choi, J. R. Haw, I. W. Kim and J. K. Lee, J. Microbiol. Biotechnol., 2014, 24, 639–647 CrossRef CAS PubMed.
- T. S. Kim, S. K. Patel, C. Selvaraj, W. S. Jung, C. H. Pan, Y. C. Kang and J. K. Lee, Sci. Rep., 2016, 6, 33438 CrossRef CAS PubMed.
- S. K. Patel, S. H. Choi, Y. C. Kang and J. K. Lee, Nanoscale, 2016, 8, 6728–6738 RSC.
- M. Z. Anwar, D. J. Kim, A. Kumar, S. K. S. Patel, S. Otari, P. Mardina, J. H. Jeong, J. H. Sohn, J. H. Kim, J. T. Park and J. K. Lee, Sci. Rep., 2017, 7, 15333 CrossRef PubMed.
- S. K. Patel, S. H. Choi, Y. C. Kang and J. K. Lee, ACS Appl. Mater. Interfaces, 2017, 9, 2213–2222 CrossRef CAS PubMed.
- S. K. S. Patel, M. Z. Anwar, A. Kumar, S. V. Otari, R. T. Pagolu, S.-Y. Kim, I.-W. Kim and J.-K. Lee, Biochem. Eng. J., 2018, 132, 1–8 CrossRef CAS.
- Z. Zhou and M. Hartmann, Chem. Soc. Rev., 2013, 42, 3894–3912 RSC.
- C.-H. Lee, T.-S. Lin and C.-Y. Mou, Nano Today, 2009, 4, 165–179 CrossRef CAS.
- J. Liu, Q. Yang and C. Li, Chem. Commun., 2015, 51, 13731–13739 RSC.
- A. Salis, D. Meloni, S. Ligas, M. F. Casula, M. Monduzzi, V. Solinas and E. Dumitriu, Langmuir, 2005, 21, 5511–5516 CrossRef CAS PubMed.
- Y. Li, G. Zhou, C. Li, D. Qin, W. Qiao and B. Chu, Colloids Surf., A, 2009, 341, 79–85 CrossRef CAS.
- H. Zhang, Y. Zou, Y. Shen, X. Gao, X. Zheng, X. Zhang, Y. Chen and J. Guo, BioEnergy Res., 2014, 7, 1541–1549 CrossRef CAS.
- Y. Zhang, J. Li, D. Han, H. Zhang, P. Liu and C. Li, Biochem. Biophys. Res. Commun., 2008, 365, 609–613 CrossRef CAS PubMed.
- C. A. Palla, C. Pacheco and M. E. Carrín, Biochem. Eng. J., 2011, 55, 199–207 CrossRef CAS.
- H. T. Deng, J. J. Wang, M. Ma, Z. Y. Liu and F. Zheng, Chin. Chem. Lett., 2009, 20, 995–999 CrossRef CAS.
- M. G. Aucoin, F. A. Erhardt and R. L. Legge, Biotechnol. Bioeng., 2004, 85, 647–655 CrossRef CAS PubMed.
- S. Lu, J. He and X. Guo, AIChE J., 2010, 56, 506–514 CAS.
- D. Zhao, Q. Huo, J. Feng, B. F. Chmelka and G. D. Stucky, J. Am. Chem. Soc., 1998, 120, 6024–6036 CrossRef CAS.
- X.-m. Wang, X.-z. Du, C.-l. Li and X. Cao, Appl. Surf. Sci., 2008, 254, 3753–3757 CrossRef CAS.
- S. K. S. Patel, S. V. Otari, Y. Chan Kang and J.-K. Lee, RSC Adv., 2017, 7, 3488–3494 RSC.
- J. Zhu, Y. Zhang, D. Lu, R. N. Zare, J. Ge and Z. Liu, Chem. Commun., 2013, 49, 6090–6092 RSC.
- Q. Jin, G. Jia, Y. Zhang, Q. Yang and C. Li, Langmuir, 2011, 27, 12016–12024 CrossRef CAS PubMed.
- N. A. Vodolazkaya, C. Despas, B. Lebeau, C. Marichal and A. Walcarius, J. Sol-Gel Sci. Technol., 2012, 63, 587–594 CrossRef CAS.
- Q. Jin, G. Jia, Y. Zhang, Q. Yang and C. Li, Langmuir, 2011, 27, 12016–12024 CrossRef CAS PubMed.
- S. L. Burkett, S. D. Sims and S. Mann, Chem. Commun., 1996, 1367–1368 RSC.
- M. Zheng, X. Xiang, S. Wang, J. Shi, Q. Deng, F. Huang and R. Cong, Process Biochem., 2017, 53, 102–108 CrossRef CAS.
- J. Liu, S. Bai, Q. Jin, H. Zhong, C. Li and Q. Yang, Langmuir, 2012, 28, 9788–9796 CrossRef CAS PubMed.
- M. Shakeri and K. Kawakami, Microporous Mesoporous Mater., 2009, 118, 115–120 CrossRef CAS.
- J. Wang, G. Meng, K. Tao, M. Feng, X. Zhao, Z. Li, H. Xu, D. Xia and J. R. Lu, PLoS One, 2012, 7, e43478 CrossRef CAS PubMed.
- M. N. Tahir, A. Adnan, E. Stromberg and P. Mischnick, Bioprocess Biosyst. Eng., 2012, 35, 535–544 CrossRef CAS PubMed.
- B. Chen, C. Yin, Y. Cheng, W. Li, Z.-A. Cao and T. Tan, Biomass Bioenergy, 2012, 39, 59–66 CrossRef CAS.
- Y. Z. Chen, C. B. Ching and R. Xu, Process Biochem., 2009, 44, 1245–1251 CrossRef CAS.
- G. Yang, J. Wu, G. Xu and L. Yang, J. Mol. Catal. B: Enzym., 2009, 57, 96–103 CrossRef CAS.
- A. Illanes, A. Cauerhff, L. Wilson and G. R. Castro, Bioresour. Technol., 2012, 115, 48–57 CrossRef CAS PubMed.
- S. G. Wang, W. D. Zhang, Z. Li, Z. Q. Ren and H. X. Liu, Appl. Biochem. Biotechnol., 2010, 162, 2015–2026 CrossRef CAS PubMed.
- Y. Jiang, L. Shi, Y. Huang, J. Gao, X. Zhang and L. Zhou, ACS Appl. Mater. Interfaces, 2014, 6, 2622–2628 CrossRef CAS PubMed.
Footnote |
† Electronic supplementary information (ESI) available. See DOI: 10.1039/c8nj04312d |
|
This journal is © The Royal Society of Chemistry and the Centre National de la Recherche Scientifique 2019 |