DOI:
10.1039/C8NJ03847C
(Paper)
New J. Chem., 2019,
43, 444-453
α-Linolenic acid-modified pluronic 127-CS copolymeric micelles for the skin targeted delivery of amphotericin B
Received
1st August 2018
, Accepted 14th November 2018
First published on 20th November 2018
Abstract
In this study, an α-linolenic acid modified pluronic F127-block-chitosan (F127-(CS-LNA)2) copolymer was synthesized to prepare topical amphotericin B (AMB)-loaded micelles (AMB-M) via a dialysis technique. The drug encapsulation efficiency, particle size and zeta potential of AMB-M were 97.26 ± 2.85%, 140.00 ± 0.36 nm and 7.00 ± 0.54 mV, respectively. Characterization of AMB-M by Fourier transform infrared spectroscopy and powder X-ray diffraction revealed the presence of an interaction between AMB and the copolymer, and that AMB was present in AMB-M without any crystallization. AMB-M exhibited in vitro antifungal activity comparable to that of a commercial AMB injection (AMB-I), although it showed a slower AMB release than that of AMB-I. AMB-M showed a 310-fold increase in the drug skin deposition and better in vivo activity against a fungal infection of the skin as compared to commercial AMB liposomal gel, a topical formulation, which shows no AMB penetration through the skin. In addition, AMB-M showed inconspicuous skin irritation, and was stable at 4 °C for 30 days. In conclusion, F127-(CS-LNA)2 may be a promising and safe drug delivery vehicle for AMB in the treatment of topical skin fungal infections.
1. Introduction
Every year, many people are at risk of developing fungal infections. Fungal infections disturb various parts of the body, including the skin, eyes, nails or main organs. The warm and moist condition of the skin provides a suitable environment for fungal growth, and at least 20% of the population in the world is at risk from different types of superficial fungal infections. In addition, diseases such as cancer, diabetes, and HIV/AIDs increase the rate of superficial fungal infections (such as cutaneous albicans) in patients.1 However, owing to frequent treatment with antiretroviral and immunosuppressant drugs, the low immune systems of these patients makes it difficult to cure their superficial fungal infections with systemic antifungal administration. So, it is necessary to topically deliver antifungal drugs for the management of superficial fungal diseases and to improve their uptake in patients.
Amphotericin B (AMB), a highly hydrophobic drug with a broad antifungal spectrum, is used to treat systemic and local fungal infections.2,3 It is effective for treating burns-related fungemia caused by the Candida species as a first-line drug.4 It is also used as a drug of choice for treating cutaneous leishmaniasis or for impregnated mycosis treatment.5 However, clinical injection of AMB shows a low effect when topically administered because of its poor or negligible cutaneous deposition and permeation. Now, two formulations, including a cream and liposomal carrier-based gel, are clinically available to achieve topical AMB delivery based on their ability to enhance the permeation and deposition of AMB.1 In addition, microemulsion,6 nanoethosome,1 nanoemulsion,7–9 solid lipid nanoparticles10 and nanoemulsion gel11 formulations have also been studied for the topical delivery of AMB and shown to have increased skin deposition and permeation properties.
Amphiphilic block copolymers can self-assemble into nanoscale micelles in aqueous solution and be used to encapsulate hydrophobic drugs owing to their unique core–shell structure and high drug loading capacity.12 It has been confirmed that amphiphilic copolymer micelle carriers are capable of promoting the skin permeation and deposition of a drug due to their small particle size and good surface activity.13,14 We have previously prepared ketoconazole-loaded methoxy poly(ethylene glycol)-b-poly(δ-valerolactone) micelles that were shown to significantly enhance the skin deposition and permeation of ketoconazole without skin leakage,15 and in another of our studies, we also showed its ability to selectively deposit in skin with low skin leakage into the blood circulation and its obvious skin targeting abilities.16
Herein, we present the design and synthesis of an α-linolenic acid (LNA)-modified pluronic 127-chitosan (F127-(CS-LNA)2) copolymer. The copolymer was used in the preparation of AMB-loaded micelles (AMB-M) to achieve the transdermal delivery of AMB. Chitosan (CS) is a natural polysaccharide. Its amino and hydroxyl groups can be easily modified with other functional groups to attain different goals. It has been reported that CS changes the secondary structure of keratin in the stratum corneum, increasing the amount of water in the stratum corneum and enhancing the cell membrane fluidity of the epidermis to various degrees.17 LNA can promote drug permeation across the skin by means of stratum corneum disruption/fluidization and tight junction/desmosome expansion.18 So, it is hypothesized that the prepared copolymer can self-assemble into micelles to encapsulate AMB and promote its skin permeation and deposition. The physicochemical properties of AMB-M were investigated via critical micelle concentration (CMC), Fourier transform infrared spectroscopy (FT-IR) and powder X-ray diffraction (PXRD) measurements. To evaluate the micellar biocompatibility, the hemolysis and aggregation of AMB-M were tested. The in vitro drug release, antifungal activity and potential of AMB-M for topical delivery were also evaluated.
2. Materials and experiments
2.1 Materials
CS (Mw = 1000, 98% deacetylation) was purchased from Zhejiang Golden-shell Pharmaceutical Co., Ltd (Yuhuan, China). F127 (Mw = 13
000) was bought from BASF Corp. (Wyandotte, MI). p-Nitrophenyl chloroformate (NPC) was obtained from Sigma-Aldrich (St. Louis, MO). NPC-activated F127 (NPC-F127-NPC) was obtained according to the previous literature19N-(3-dimethylamino-propyl)-N-ethyl carbodiimide hydrochloride (EDC·HCl) and N-hydroxysuccinimide (NHS) were bought from Sinopharm Chemical Reagent Co., Ltd (Shanghai, China). Linolenic acid (LNA) was purchased from Linuo Biochemistry Co., Ltd (Anyang, China). Injectable AMB powder (AMB-I), composed of AMB, sodium deoxycholate and sodium hydroxide, was obtained from North China Pharmaceutical Co., Ltd (Shijiazhuang, China). Amfy gel (lipid based AMB gel, AMB-G, 0.1%) was purchased from Intas Pharmaceuticals Ltd (Ahmedabad, India). All other reagents were of analytical grade.
2.2 Synthesis of F127-CS
NPC-F127-NPC (10.0 g, 0.7 mmol) and CS (1.7 g, 1.7 mmol) were codissolved in dimethyl sulfoxide (DMSO) and stirred at 50 °C for 24 h. The resultant mixture was dialyzed against deionized water for 24 h and freeze-dried to obtain F127-CS.
2.3 Synthesis of F127-(CS-LNA)2
LNA (0.1 g, 0.4 mmol) in DMSO was activated with EDC·HCl (85 mg, 0.4 mmol) and NHS (70 mg, 0.4 mmol) overnight at room temperature. After the addition of F127-CS (3.0 g, 0.2 mmol), the resulting mixture was stirred at room temperature for 24 h. The mixture was dialyzed against deionized water for 24 h and freeze-dried to obtain F127-(CS-LNA)2.
2.4 Measurement of the copolymer critical micelle concentration (CMC)
The CMC of the copolymer was determined according to a previous reported procedure.20 A pyrene solution in acetone (4.2 × 10−2 mg mL−1) was evaporated to dryness in a test tube under a nitrogen flow. A copolymer solution (from 5.0 × 10−3 to 5.0 × 10−7 g mL−1) was added into the tube to obtain a final pyrene concentration of 4.2 × 10−4 mg mL−1. The obtained sample was shaken at a rate of 100 rpm at 60 °C for 1 h, maintained at room temperature overnight and then filtered through a Millipore filter (0.45 μm, Jinteng laboratory equipment Co., Ltd, China). Fluorescence excitation spectra were recorded from 300 to 360 nm with an emission wavelength of 390 nm using a fluorescence spectrometer (F-7000 FL, Hitachi Corp, Japan).
2.5 Preparation and characterization of AMB-M
The copolymer and AMB were codissolved in 2 mL of DMSO and dialyzed (dialysis bag, MWCO 2 kDa) for 24 h. The solution was filtered through a Millipore filter (0.22 μm, Jinteng laboratory equipment Co., Ltd, China) to remove the free drug or other substances. Blank micelles were prepared using the same process without any drug.
The particle size distribution and zeta potential of AMB-M were investigated using a Zetasizer ZS90 (Malvern Instruments Ltd, UK) at room temperature.
A carbon film-coated copper grid covered with AMB-M was negatively tinted with 2% phosphotungstic acid and dried at room temperature. Transmission electron microscopy (TEM) images of the sample were obtained using a JEM 1200EX transmission electron microscope.
To evaluate the stability of AMB-M, the content of AMB in micelles was determined after storage for 0, 1, 3, 7, 14, 21 and 30 days. All samples were stored in the dark at 4 °C.
2.6 Determination of the entrapment efficiency and study of the aggregation state of AMB-M
The AMB content in AMB-M was determined using a UV-vis spectrophotometer at 416 nm (UV-2600, Shimadzu Corporation, Japan). The drug loading (DL) and entrapment efficiency (EE) of AMB-M were calculated according to the following equations:
As a comparison, crude AMB was dissolved in DMSO to break up the reversible aggregation. AMB-I and AMB-M solutions were prepared, respectively. UV-visible spectra of the samples from 300 to 500 nm were recorded using a UV-visible spectrophotometer (UV-2600, Shimadzu Corporation, Japan).
2.7 FT-IR and PXRD
Using a pellet method, the FT-IR spectra of crude AMB, blank micelles and drug-loaded micelles were obtained using a FT-IR spectrophotometer (Spectrum One, PerkinElmer, USA) in the range of 4000–400 cm−1.
Wide angle X-ray diffraction measurements of crude AMB, blank micelles and drug-loaded micelles were performed using an X-ray diffractometer (Bruker D8 Focus, Bruker, Germany) with Cu Kα radiation (40 kV, 30 mA). The scattered angle 2θ was set at a range of 3°–50°.
2.8
In vitro drug release
The in vitro release of AMB from AMB-M and AMB-I was performed in an isotonic Palitzsch buffer medium (20 mM, pH 7.4) containing Tween 80 (0.5%, v/v) using a dialysis method (MWCO, 8k–14k Da).21 Using AMB-I (equivalent to 0.4 mg of AMB) as a control, dialysis bags containing 4 mL of AMB-M were sunk into 96 mL of a release medium and incubated at an oscillation rate of 100 rpm at 37 °C. At specified time intervals, 1 mL of the release medium was withdrawn for HPLC analysis at a wavelength of 416 nm. 1 mL of fresh release medium was used to replace the volume sampled. The mobile phase used was acetonitrile
:
10 mM sodium acetate buffer (pH 4.0, (40
:
60, v/v)) and the flow rate was 1 mL min−1. The AMB accumulative release percentage was calculated and described as the mean ± standard deviation (SD) of the four replicates, and its accumulative release curve was plotted.
2.9
In vitro antifungal efficacy
The antifungal activity of AMB-M against Candida albicans was performed using a microdilution method.22Candida albicans was cultivated with a SDA medium by shaking at 28 °C for 12 h and was then diluted to afford a bacteria sample at a concentration of 2–5 × 106 mL−1. AMB-M and AMB-I solutions were diluted with sterile water using the half dilution method over a concentration range of 0.0625–8 μg mL−1. Equivalent volumes of bacteria samples and AMB formulations were mixed in 96-well plates and cultivated at 28 °C for 24 h. Medium containing fungi and sterile medium were set as the positive and negative controls, respectively. The inhibition percentage of fungal growth in each pore was calculated after determination of the optical density (OD) at 600 nm in a microplate reader (1510, Thermo Fisher Scientific, Waltham, MA).
2.10 Cellular uptake
NHS Rhodamine B ester (RB-NHS) was prepared according to a previously reported method.23 F127-(CS-LNA)2 (90 mg) was treated with RB-NHS (1 mg) in DMSO without light at room temperature for 48 h. The mixture was dialyzed against distilled water in the dark and lyophilized to afford RB-modified F127-(CS-LNA)2 (RB-F127-(CS-LNA)2). Candida albicans, at a concentration of 2–5 × 106 mL−1 in SDA medium, was mixed with RB-F127-(CS-LNA)2 solution and cultivated at 28 °C for 20 min, 1 h and 3 h, respectively. The resulting mixture was centrifuged and washed with PBS (pH 7.4) three times to remove any free RB-F127-(CS-LNA)2. The obtained fungal sample was then redistributed in PBS to observe its fluorescence intensity using a fluorescence inverted microscope (DMI8, Leica, Germany).
2.11
Ex vivo skin permeation and deposition
Male Kunming mice (25 ± 2 g), obtained from Shandong University, were housed under normal conditions and allowed free access to food and water. All procedures were performed after approval from the Animal Ethics Committee of University of Jinan and in accordance with the Guidelines for Care and Use of Laboratory Animals of University of Jinan (SYXK (Lu) 20160001).
Drug permeation and deposition studies were performed using a vertical Franz diffusion cell according to a previous report6 The back hair of the mice was removed with depilatory cream. A skin sample was surgically excised, washed with saline and stored at −20 °C prior to the experiments.
The receiver chamber of the Franz diffusion cell (diffusion area of 1.327 cm2) was filled with 14 mL of isotonic Palitzsch buffer medium (pH 7.4) containing Tween 80 (0.5%, v/v). The skin was mounted between the donor and receptor chamber with the epidermal surface facing up, to equilibrate it for 1 h. AMB-M, AMB-I, a mixture of AMB and LNA (AMB-LNA) and AMB-G (containing 56 μg of AMB) were added to the donor chamber at 32 °C, respectively. At predetermined times, 0.3 mL of the release solution was withdrawn and centrifuged prior to HPLC analysis. The receptor chamber was refilled with 0.3 mL of the fresh buffer medium after each sample was taken. At the end of the experiment, the skin was discharged, washed with 50% methanol and deionized water and cut into small pieces in turn. The pieces were immersed in 5 mL of methanol overnight in the dark and centrifuged for HPLC analysis. The reported results are based on four sets of measurements.
2.12 Visualization of the skin permeation and deposition of drug-loaded micelles
Fluorescein-loaded micelles were prepared according to the procedure in Section 2.5, and 1 mg of fluorescein was completely dispersed in 10 mL of water to prepare a saturated solution of it as a control after filtration through a Millipore filter (0.22 μm). The two solutions were treated according to the same procedure as in the ex vivo skin permeation and deposition experiments (Section 2.11). At each time point, the skin was removed and washed with 50% methanol solution and deionized water, and slice samples of the skin cross-section were prepared via a frozen section method to visualize the skin permeation of the formulation using inverted fluorescence microscopy (DMI8, Leica, Germany). The mean fluorescence intensities of the samples were calculated using the Image J 1.48v software.
2.13 Skin irritation study
The hair on the back of the mice was removed with depilatory cream and coated with AMB-M, AMB-G and AMB-I, respectively, according to a previously reported method.11 Saline and sodium sulfide solution was set as the negative and positive controls, respectively. To evaluate the skin irritation, the severity of erythema or edema at the treatment site of each formulation was observed, evaluated and photographed after treatment for 24 h. After that, paraffin sections were prepared for hematoxylin–eosin staining evaluation after the skin samples were cut off and sunk into neutral formalin, respectively. Paraffin section photographs were obtained using a microscope (DMI8, Leica, Germany).
2.14
In vivo antifungal evaluation against skin infection
Balb/c mice were intraperitoneally injected with dexamethasone sodium phosphate once daily at a dosage of 5 mg kg−1 for 3 days.21 Dorsal seta was removed with depilatory cream, slightly scratched with sandpaper and coated with a suspension of Candida albicans. The bacteria sample was supplied once a day for 3 days to establish a skin infection model. Then, topical treatment was administrated daily by applying AMB-M or AMB-G (equivalent to 8.5 μg of AMB)24 to the infected site for 7 days, respectively. Mice without any drug administered were used as positive controls. After drug administration, the infected site was photographed to trace its healing progression at 0, 1, 2 and 3 days. On the last day, a skin specimen from the infected site was taken and homogenized in saline. The homogenate was striped on a solid medium and incubated at 28 °C for 48 h. A number of colonies formed on the solid plate.
2.15 Statistical analysis
All data is expressed as the mean ± standard deviation (SD). Statistical analysis was performed using a Student's t-test with a level of significance at P < 0.05.
3. Results and discussion
3.1 Synthesis of F127-(CS-LNA)2
CS-F127 was synthesized through a reaction between NPC-F127-NPC and CS. LNA was activated with NHS and EDC and selectively reacted with the amino group of CS-F127 to afford the F127-(CS-LNA)2 copolymer (Fig. 1).
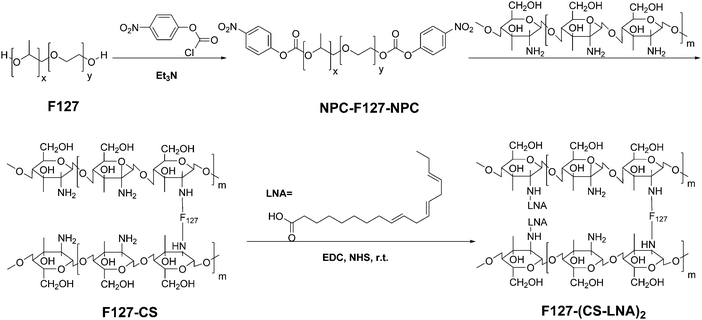 |
| Fig. 1 Synthetic route of F127-(CS-LNA)2. | |
The structure of F127-(CS-LNA)2 was confirmed by 1H-NMR spectroscopy (AVANCE III 600 MHz Digital NMR Spectrometer). The 1H-NMR spectrum of the F127-(CS-LNA)2 copolymer is shown in Fig. 2. Multiplet peaks at 3.48–3.55 ppm and doublet peaks at 1.04 and 1.05 ppm in the spectrum are characteristic of F127.19 Multiplet peaks at 3.42–3.46 ppm were assigned to the hydrogen signals of the CS backbone, and triplet peaks at 2.78 ppm were assigned to the amide proton of CS.19 Chemical shifts at 0.8–0.94 ppm, 5.3 ppm and 1.13 ppm revealed that there were characteristic signals of CH3, –CH
CH– and CH
CH–CH2–, respectively, of LNA in the prepared copolymer.25 Based on the integral area at 1.04, 5.3 and 2.78 ppm, the component ratios of F127, LNA and CS were found to be 1
:
1.9
:
2. In addition, gel chromatography of the copolymer was also performed with tetrahydrofuran as the mobile phase using a GPC system equipped with an isocratic pump (Waters 1525), an autosampler (Waters 717 plus), a column oven and a dual λ absorbance detector (Waters 2487) with an integrated temperature controller maintained at 35 °C. Its molecular weight (Mw) was 11
826 with a polydispersity of 1.02. All of the results confirmed that the F127-(CS-LNA)2 copolymer was successfully synthesized.
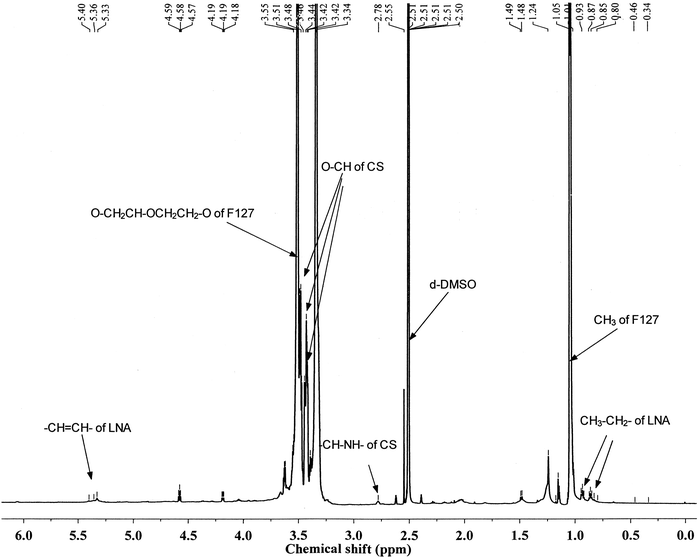 |
| Fig. 2
1H-NMR spectrum of F127-(CS-LNA)2. | |
3.2 CMC
Like other amphiphilic copolymers, the amphiphilic F127-(CS-LNA)2 copolymer can self-assemble into micelles with a hydrophobic inner core and hydrophilic outer shell. Pyrene is highly hydrophobic and preferentially migrates into the lipophilic micellar core in aqueous solution.26 When the environment surrounding the pyrene changes from polar (water) to non-polar (inner core of the micelles), the pyrene excitation peak red shifts from 335 to 337 nm. Therefore, the change in the intensity ratio of 337 to 335 nm in the fluorescence excitation graph indicates whether the copolymer forms micelles. In this study, we determined the CMC value of the copolymer with pyrene as a test subject using fluorescence excitation spectroscopy. The ratio of the I337/I335-copolymer concentration curve is shown in Fig. 3. The CMC was calculated through Sigmoidal-Boltzmann fitting in the Origin 8.5 software. The CMC value of F127-(CS-LNA)2 was 2.2 × 10−4 g mL−1, lower than that of F127 itself due to the introduction of hydrophobic LNA.27 The low CMC made the copolymer form stable micelles.
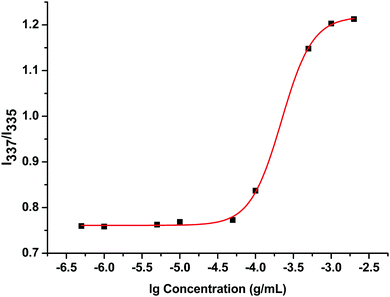 |
| Fig. 3 Intensity ratio of I337/I335vs. lg C for F127-(CS-LNA)2. | |
3.3 Characterization of micelles
F127-(CS-LNA)2 was used to prepare AMB-M via a dialysis method. The drug encapsulation efficiency and loading capacity of drug-loaded micelles were found to be 97.26 ± 2.85% and 12.51 ± 0.39%, respectively. The water-solubility was enhanced to 1.68 mg mL−1, 1.68 × 103 times higher than that of crude AMB in water.28 Its particle size and zeta potential were measured as 140.00 ± 0.36 nm and 7.00 ± 0.54 mV, respectively. The TEM image of AMB-M shows that it has spherical shaped particles (Fig. 4A). The EE of AMB-M at each time point was determined to investigate its stability at 4 °C. From the results shown in Fig. 4B, the EE was found to be between 93 and 97% over the whole storage period, showing negligible change. This indicates the good stability of AMB-M.
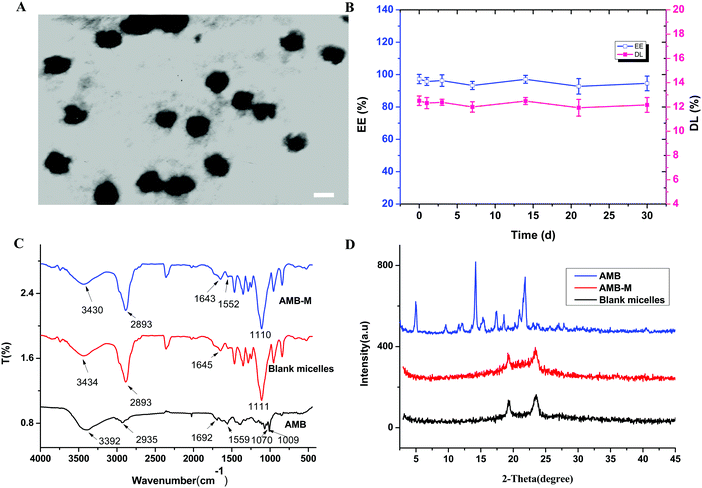 |
| Fig. 4 (A) TEM image of AMB-M (the bar represents 100 nm, ×53 000), (B) stability of AMB-M, (C) FT-IR of crude AMB, blank micelles and AMB-M, and (D) PXRD patterns of crude AMB, blank micelles and AMB-M. | |
The FT-IR spectra of crude AMB, blank micelles and AMB-M are shown in Fig. 4C. Crude AMB showed characteristic signals, such as a carbonyl stretching vibration peak at 1692 cm−1, a C
C stretching vibration band at 1559 cm−1, strong C–O stretching vibration bands of the CH–OH group at 1070 and 1009 cm−1 and a strong –OH or –NH stretching vibration peak at 3392 cm−1. After being encapsulated into the copolymeric micelles, crude AMB underwent peak shifts from 1692 and 1559 cm−1 to 1552 and 1643 cm−1, respectively, and the peak at 3392 cm−1 was covered by the peak at 3430 cm−1. At the same time, the peaks at 1070 and 1009 cm−1 were covered by the strong peak of the blank micelles at 1110 cm−1. In the spectrum of the blank micelles, characteristic peaks for –OH and –NH of CS were present at 3434 cm−1. The carbonyl stretching vibration peak of the amide appeared at 1645 cm−1. These vibration peaks shifted to 3430 and 1643 cm−1, respectively, after encapsulation of AMB. Meanwhile, the peak at 3430 cm−1 became stronger when compared to that for the blank micelles. The aforementioned peak position shift suggested that there might be hydrogen bond interactions among the amino, hydroxyl or carboxyl groups of AMB and the copolymer. The interactions assisted in the encapsulation of AMB into the copolymeric micelles and affected the release of AMB from AMB-M.
The PXRD profiles of crude AMB, blank micelles and AMB-M were measured to investigate the physical state of AMB in the micelles. As shown in Fig. 4D, the characteristic peaks of crude AMB appeared at 4.96°, 14.20°, 17.44°, 18.56° and 21.84°,29 suggesting the high crystallinity of the crude AMB. However, these peaks disappeared when the crude AMB was loaded into the copolymeric micelles. This result suggests that AMB was present in an amorphous form or solid solution. The amorphous state of AMB originated from its homogeneous molecular interaction with the copolymer, which was confirmed in the aforementioned FT-IR analysis. The interaction is helpful in the slow and sustained release of AMB from the copolymeric matrix and its dispersion.
3.4
In vitro release
Due to its low water-solubility and amphiphilicity, AMB self-assembles into aggregates in neutral or weak alkaline aqueous media.28 The aggregates reduce the rate of diffusion of AMB from the dialysis bags to the release medium. Hence, Tween 80 was applied as a solubilizer in the release medium to maintain sink conditions. The in vitro release profile is shown in Fig. 5A. The release of AMB from AMB-M displayed a similar release procedure to AMB-I formulations with an initial burst release in the first 6 h. This phenomenon can be attributed to the fact that a certain amount of AMB is located on/near to the outer surface of the micelles. About 24% of AMB was released from AMB-M with a steady and slow rate over 48 h. 44% of AMB was released, exhibiting quick release for AMB-I within the same period. These results demonstrated that AMB-M showed slow, controlled and sustained release when compared to AMB-I. The slow and sustained release of AMB from AMB-M is due to its hydrogen bond interactions with the copolymer (discussed in Section 3.3). AMB-M could be used as an AMB reservoir to maintain the sustained release of AMB when topically applied in the treatment of fungal skin infections.
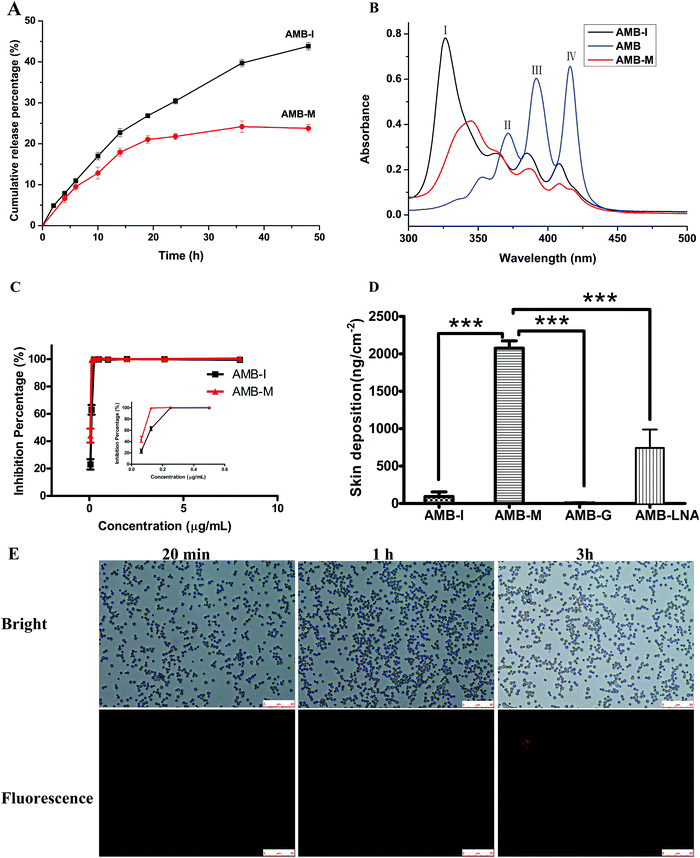 |
| Fig. 5 (A) In vitro release of AMB from AMB-M and AMB-I in isotonic Palitzsch buffer containing 0.5% Tween 80 at 37 °C (n = 4), (B) UV-visible spectra of crude AMB, AMB-I and AMB-M, (C) in vitro inhibition percentage of the fungal growth for Candida albicans treatment with AMB-I and AMB-M at different concentrations at 28 °C (n = 6), and (D) skin deposition of the drug after ex vivo permeation studies of AMB-M compared against AMB-I, AMB-LNA and AMB-G (n = 4, *** p ≤ 0.005), and (E) fluorescence and bright field images of the cellular uptake after treatment of Candida albicans with rhodamine B-modified F127-(CS-LNA)2 for different times. | |
3.5 Aggregation assessment
AMB operates through the disturbing cell membrane of fungi. As an amphiphilic compound, AMB exists in aggregate and monomer form, and it tends to self-assemble into aggregates in water due to its poor water solubility. Monomeric AMB selectively interacts with ergosterol in the fungal cell membrane. In contrast, its aggregate is nonspecific and induces channel formation in both fungal and mammalian cell membranes, resulting in host toxicity.30 In this study, the aggregations of AMB-M, crude AMB and AMB-I were analyzed using UV-vis spectroscopy. AMB dissolved in DMSO showed peaks at 416 nm (band IV), 391 nm (band III) and 371 nm (band II) (Fig. 5B). The presence of band IV shows that AMB was completely dissolved in the form of its monomer. As for AMB-I, its absorbance spectrum showed a strong peak at 326 nm (band I) (Fig. 5B), indicating its aggregation in aqueous medium.31 The absorbance ratio of band IV to band I is 0.175. In terms of AMB-M, the absorption peak at 345 nm showed a red shift in comparison to that of band I of AMB-I, partly overlapping with band II. The absorbance ratio of band IV to bond I is 0.485, higher than that for AMB-I. The increased absorbance ratio suggests that the amount of monomeric AMB in AMB-M was much higher than that in AMB-I, indicating its low toxicity against mammalian cells.30
3.6
In vitro antifungal activity
The antifungal activities of AMB-M and AMB-I were evaluated by means of a microdilution method. Fig. 5D shows that AMB-M and AMB-I inhibited fungal growth with concentration-dependent characteristics, and that their minimum inhibitory concentration, MIC90, values are 0.125 μg mL−1 and 0.25 μg mL−1 respectively. The MIC value of AMB-I was found to be similar to that reported previously.32 Compared to AMB-I, the slow release of AMB from AMB-M (discussed in Section 3.3) should make it difficult to reach an effective concentration, resulting in low activity. The in vitro antifungal experimental results indicated their similar activity. Moreover, we investigated the cellular uptake of RB-F127-(CS-LNA)2 by Candida albicans at different time points, and the results are shown in Fig. 5E. At 20 min, there was no red fluorescence across all fields. After cultivation for 1 h, a few bright fluorescent points were observed. At 3 h, a great number of fungal cells displayed red fluorescence, and some brighter fluorescent points were present when compared with the results at 1 h. The increase in the fluorescence intensity confirmed the promoting effect of the F127-(CS-LNA)2 on cellular uptake of AMB-M.
On the basis of the abovementioned results, the comparable activity might be associated with the surfactivity and small particle size of AMB-M instead of the slow AMB release from AMB-M.33 The small particle size (less than 150 nm) made the micelle diffusion and flow easy, causing easy penetration of AMB-M into fungi cells through fungi membrane pores within a short period (Fig. 5E). Its good surfactivity and electropositivity might strengthen its interaction with the negative fungi cell membrane composed of hydrophilic/hydrophobic components. All of these factors jointly contributed to the good cellular uptake of Candida albicans in comparison with AMB-I (Fig. 5E). At the same time, the AMB-M around or in the cell was able to play a role for a longer time due to its continuous drug release.
3.7
Ex in vivo skin permeation and deposition
In vitro skin permeation and deposition experiments were performed using Franz diffusion cell technology using mice skin. In terms of AMB-I, AMB-G, AMB-LNA and AMB-M, it was found that no AMB was detected in the receiver chamber during the whole experiment. The AMB content in the receptor was undetectable because its concentration was perhaps lower than the detection limit of the analytical method.34
Next, we measured the deposition of AMB in the skin treated with the formulations at the end of the abovementioned experiment and the results are listed in Fig. 5D. The accumulative skin deposition of the drug was found to be 6.68 ± 2.69 ng cm−2 and 2076 ± 97 ng cm−2 for AMB-G and AMB-M, respectively. The AMB-M achieved good skin deposition of AMB, 310-fold higher than that of AMB-G, respectively. We conjecture that the poor diffusion of pasty AMB-G might be the main reason for its low skin deposition, although it was able to firmly coat the surface of the skin. In order to confirm the conjecture, AMB-I solution with good AMB diffusion was employed to perform skin deposition ability tests. It was found that AMB-I increased the skin deposition of AMB to 94 ± 61 ng cm−2 as compared to AMB-G, showing the promotive effect of the diffusion. However, AMB-I is a negatively charged formulation that repels negatively charged skin cells to some extent, resulting in a reduced skin deposition when compared with AMB-M. It has been reported that the follicular pathway is the preferred penetration route for amphiphilic micelles.14 So, as for AMB-M, its compact structure with a small hydrodynamic radius and good diffusion should ensure its easy skin permeation and deposition through the skin follicular pathway.35 The interaction of cationic CS in the copolymer with the negatively charged skin cells also resulted in the improved skin permeation of AMB-M.34,36 It has been confirmed that LNA is a skin permeation enhancer that weakens the barrier properties of the stratum corneum via the loosening of the packing of the lipid bilayer, thus enhancing its fluidity.37 The effect of conjugation of LNA to F127-CS on the skin deposition of AMB was also investigated. In the AMB-LNA treatment group, the skin deposition was 742 ± 123 ng cm−2, higher than that for AMB-I or AMB-G. This result indicated the enhancing effect that LNA has on the skin deposition in comparison with AMB-I. However, AMB-M was found to obviously improve the skin deposition of AMB when compared to AMB-LNA. Therefore, the combination of LNA and CS chains was also helpful for improving the skin deposition. The above results suggest that AMB-M is suitable for the treatment of fungal skin infections. The reduced aggregation rate of AMB in AMB-M lowers the toxicity of AMB to the skin and its surrounding tissues. The continuous release of AMB was favorable in delivering a therapeutic effect for a long period time, thus reducing the drug administration frequency.
The finer permeation of the micelle delivery vehicle was further confirmed using fluorescence microscopy. The fluorescence intensity was observed to be nearly negligible for the fluorescein solution (control) at 3 h (shown in Fig. 6A), with a value of only 0.007. At the same time, lower fluorescence intensity arose at a density value of 0.033 in the skin stratum corneum for fluorescein-loaded micelles and the fluorescence intensity gradually increased over time. Weak fluorescence was observed in the stratum corneum for the fluorescein solution at 12 h, and the mean fluorescence density increased to 0.019. In contrast, the fluorescein-loaded micelle group showed high fluorescence intensity in the dermis layer of the skin at 12 h, and many strong fluorescent spots appeared in the deeper skin. The total fluorescence density in the sample was about 0.204. In other words, like the ex vivo skin permeation and deposition experiments, the fluorescence microscopy results also indicated that the micelles significantly promoted the deposition of drug in the skin when compared to the AMB-I solution.
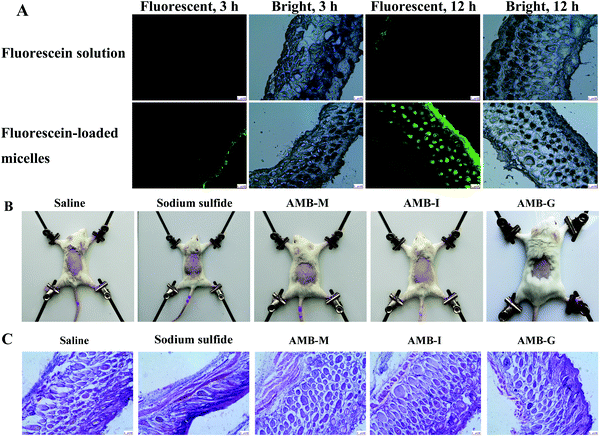 |
| Fig. 6 (A) Fluorescence images of frozen skin sections after treatment with fluorescein solution and fluorescein-loaded micelles for 3 and 12 h in a Franz diffusion cell, respectively, (B) photographs of the skin irritation study on the back of mice after treatment with saline, sodium sulfide, AMB-M and AMB-I, and (C) photographs of skin histological sections in the skin irritation study (the bars represent 100 μm). | |
3.8 Skin irritation study
The skin irritation results are shown in Fig. 6B. Only inconspicuous erythema or edema occurred in the saline, AMB-M, AMB-G and AMB-I groups. However, sodium sulfide treated skin demonstrated significant erythema, indicating serious skin irritation. Furthermore, histological section photographs were obtained after hematoxylin–eosin staining and the corresponding results are shown in Fig. 6C. Sodium sulfide treatment resulted in obvious skin damage: inflammatory cell infiltration, epidermis desquamation and dermal cell vacuolation. The other formulations did not induce any pathological change of the treated skin. These results show that AMB-M is safe to use on skin for the treatment of fungal skin infections.
3.9
In vivo activity
The antifungal activity of AMB-M was investigated in vivo using a Candida albicans-induced skin infection model in mice with AMB-G as a control. The logarithmic value of the colony forming unit (CFU) of the positive group was 5.37 ± 0.22 g−1, and AMB-G treatment group displayed a value of 4.62 ± 0.18 g−1. In contrast, the logarithmic value was negligible in the AMB treatment group. Photographs of the infected sites are shown in Fig. 7 at 0, 1, 2 and 3 days post drug administration. On day 0, there was obvious inflammation in the positive, AMB-G and AMB-M groups. The positive group showed no sign of skin recovery except for scab formation within 3 days. As for the AMB-G group, the wound size gradually decreased, and a scab formed without skin regrowth over time. However, in the AMB-M treatment group, slight skin recovery appeared on day 1, and the infected skin site completely healed. These results demonstrate that the in vivo activity of AMB against a fungi-induced skin infection was significantly improved when it was encapsulated into F127-(CS-LNA)2 copolymeric micelles.
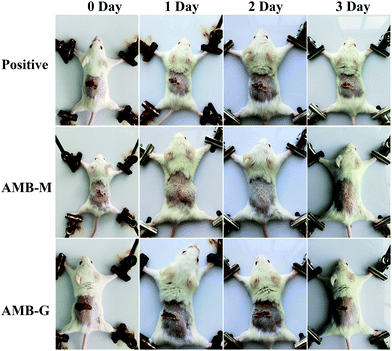 |
| Fig. 7 Photographs of mice skin after treatment without or with AMB-G and AMB-M at 0, 1, 2 and 3 days. | |
4. Conclusions
In this study, an α-linolenic acid (LNA)-modified pluronic 127-chitosan (F127-(CS-LNA)2) copolymer was synthesized and used to formulate AMB-loaded micelles. It was obvious that the drug-loaded micelles were effective for the topical delivery of AMB. When compared to a commercial AMB injection or gel, the prepared AMB-loaded micelles showed higher skin deposition, negligible skin irritation and better in vitro/in vivo anti-fungal activity with improved AMB solubility. Overall, it is apparent that the developed micelle formulation should have potential for the treatment of fungal skin infections. Further evaluation is needed to illuminate its clinical therapeutic effect.
Conflicts of interest
All authors confirm that there is no conflict of interest. All institutional and national guidelines for the care and use of laboratory animals were followed.
Acknowledgements
The work was supported by the Natural Science Foundation of Shandong Province (grant number ZR2016BL15) and the Science and Technology Project of University of Jinan (grant number XKY1732).
References
- L. Kaur, S. K. Jain, R. K. Manhas and D. Sharma, J. Liposome Res., 2015, 25, 294–307 CrossRef CAS.
- J. F. Ha, C. M. Italiano, C. H. Heath, S. Shih, S. Rea and F. M. Wood, Burns, 2011, 37, 181–195 CrossRef.
- M. Kleinberg, Int. J. Antimicrob. Agents, 2006, 27(suppl 1), 12–16 CrossRef.
- A. L. Martinez Marin, P. Gomez-Cortes, D. Carrion Pardo, N. Nunez Sanchez, G. Gomez Castro, M. Juarez, L. Perez Alba, M. Perez Hernandez and M. A. de la Fuente, J. Dairy Sci., 2013, 96, 7532–7537 CrossRef CAS.
- V. Yardley and S. L. Croft, Int. J. Antimicrob. Agents, 2000, 13, 243–248 CrossRef CAS.
- D. Butani, C. Yewale and A. Misra, Colloids Surf., B, 2014, 116, 351–358 CrossRef CAS.
- A. Hussain, V. K. Singh, O. P. Singh, K. Shafaat, S. Kumar and F. J. Ahmad, Drug Delivery, 2016, 23, 3101–3110 CrossRef CAS.
- A. Hussain, S. Singh, T. J. Webster and F. J. Ahmad, Nanomedicine, 2017, 13, 1117–1126 CrossRef CAS.
- L. Sosa, B. Clares, H. L. Alvarado, N. Bozal, O. Domenech and A. C. Calpena, Nanomedicine, 2017, 13, 2303–2312 CrossRef CAS.
- D. Butani, C. Yewale and A. Misra, Colloids Surf., B, 2016, 139, 17–24 CrossRef CAS.
- A. Hussain, A. Samad, S. K. Singh, M. N. Ahsan, M. W. Haque, A. Faruk and F. J. Ahmed, Drug Delivery, 2016, 23, 642–647 CrossRef CAS.
- K. Kataoka, A. Harada and Y. Nagasaki, Adv. Drug Delivery Rev., 2001, 47, 113–131 CrossRef CAS.
- Y. G. Bachhav, K. Mondon, Y. N. Kalia, R. Gurny and M. Moller, J. Controlled Release, 2011, 153, 126–132 CrossRef CAS.
- M. Lapteva, K. Mondon, M. Moller, R. Gurny and Y. N. Kalia, Mol. Pharmaceutics, 2014, 11, 2989–3001 CrossRef CAS PubMed.
- P. Z. Deng, F. F. Teng, F. L. Zhou, Z. M. Song, N. Meng and R. L. Feng, J. Biomater. Sci., Polym. Ed., 2017, 28, 63–78 CrossRef CAS.
- P. Deng, F. Teng, F. Zhou, Z. Song, N. Meng, N. Liu and R. Feng, Mater. Sci. Eng., C, 2017, 78, 296–304 CrossRef CAS.
- W. He, X. Guo, L. Xiao and M. Feng, Int. J. Pharm., 2009, 382, 234–243 CrossRef CAS PubMed.
- S. Rambharose, R. S. Kalhapure, K. G. Akamanchi and T. Govender, J. Mater. Chem. B, 2015, 3, 6662–6675 RSC.
- W. Rao, H. Wang, J. Han, S. Zhao, J. Dumbleton, P. Agarwal, W. Zhang, G. Zhao, J. Yu, D. L. Zynger, X. Lu and X. He, ACS Nano, 2015, 9, 5725–5740 CrossRef CAS.
- Y. Zhong, K. Goltsche, L. Cheng, F. Xie, F. Meng, C. Deng, Z. Zhong and R. Haag, Biomaterials, 2016, 84, 250–261 CrossRef CAS.
- L. Kaur, K. Singh, S. Paul, S. Singh, S. Singh and S. K. Jain, AAPS PharmSciTech, 2018, 19, 1606–1624 CrossRef CAS.
- Y. Wang, X. Ke, Z. X. Voo, S. S. Yap, C. Yang, S. Gao, S. Liu, S. Venkataraman, S. A. Obuobi, J. S. Khara, Y. Y. Yang and P. L. Ee, Acta Biomater., 2016, 46, 211–220 CrossRef CAS.
- J. R. G. Navarro and L. Bergström, RSC Adv., 2014, 4, 60757–60761 RSC.
- D. A. Sanchez, D. Schairer, C. Tuckman-Vernon, J. Chouake, A. Kutner, J. Makdisi, J. M. Friedman, J. D. Nosanchuk and A. J. Friedman, Nanomedicine, 2014, 10, 269–277 CrossRef CAS.
- Z. M. Song, W. X. Zhu, N. Liu, F. Y. Yang and R. L. Feng, Int. J. Pharm., 2014, 471, 312–321 CrossRef CAS PubMed.
- S. K. Han, K. Na and Y. H. Bae, Colloids Surf., A, 2003, 214, 49–59 CrossRef CAS.
- Y.-Y. Li, L. Li, H.-Q. Dong, X.-J. Cai and T.-B. Ren, Mater. Sci. Eng., C, 2013, 33, 2698–2707 CrossRef CAS.
- B. G. Yu, T. Okano, K. Kataoka, S. Sardari and G. S. Kwon, J. Controlled Release, 1998, 56, 285–291 CrossRef CAS.
- M. Rolon, D. R. Serrano, A. Lalatsa, E. de Pablo, J. J. Torrado, M. P. Ballesteros, A. M. Healy, C. Vega, C. Coronel, F. Bolas-Fernandez and M. A. Dea-Ayuela, Mol. Pharmaceutics, 2017, 14, 1095–1106 CrossRef CAS.
- C. Alvarez, D. R. Andes, J. Y. Kang, C. Krug and G. S. Kwon, Pharm. Res., 2017, 34, 1115–1124 CrossRef CAS.
- W. Tiyaboonchai and N. Limpeanchob, Int. J. Pharm., 2007, 329, 142–149 CrossRef CAS.
- K. Gilani, E. Moazeni, T. Ramezanli, M. Amini, M. R. Fazeli and H. Jamalifar, J. Pharm. Sci., 2011, 100, 252–259 CrossRef CAS.
- G. M. Soliman, M. A. Attia and R. A. Mohamed, Curr. Drug Delivery, 2014, 11, 753–762 CrossRef CAS.
- C. M. Santos, R. B. de Oliveira, V. T. Arantes, L. R. Caldeira, M. C. de Oliveira, E. S. Egito and L. A. Ferreira, J. Biomed. Nanotechnol., 2012, 8, 322–329 CrossRef CAS.
- R. Jijie, A. Barras, R. Boukherroub and S. Szunerits, J. Mater. Chem. B, 2017, 5, 8653–8675 RSC.
- Y. Yang, J. Bugno and S. Hong, Polym. Chem., 2013, 4, 2651–2657 RSC.
- S. Rambharose, R. S. Kalhapure, K. G. Akamanchi and T. Govender, J. Mater. Chem. B, 2015, 3, 6662–6675 RSC.
|
This journal is © The Royal Society of Chemistry and the Centre National de la Recherche Scientifique 2019 |
Click here to see how this site uses Cookies. View our privacy policy here.