DOI:
10.1039/C9NH00328B
(Minireview)
Nanoscale Horiz., 2019,
4, 1037-1045
Recent advances in functional nanomaterials for photoacoustic imaging of glioma
Received
20th May 2019
, Accepted 7th June 2019
First published on 7th June 2019
Abstract
Glioma is a malignant tumor in the central nervous system with poor prognosis, high risk of recurrence and mortality. As the primary choice of treatment for glioma, surgery cannot effectively remove glioma cells owing to the lack of advanced intraoperative navigation technology. Photoacoustic (PA) imaging, a hybrid imaging technique, integrates the merits of optical and ultrasound imaging, exhibiting great potential for cancer surgery navigation. To enhance the sensitivity and specificity for visualizing the tumor margin, abundant contrast agents have been developed for enhanced PA imaging. In this minireview, we expect to draw attention to the recent advances in constructing functional nanomaterials as PA contrast agents for glioma imaging. The design and preparation of organic and inorganic nanomaterials for glioma PA imaging are discussed in detail. Meanwhile, the current challenges and perspectives of contrast-enhanced PA imaging of glioma are presented.
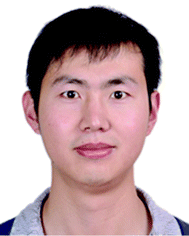
Duyang Gao
| Duyang Gao received his PhD degree from the University of Macau in 2018. Then he joined the Shenzhen Institutes of Advanced Technology, Chinese Academy of Sciences, as a postdoctoral fellow under the supervision of Prof. Hairong Zheng and Prof. Zonghai Sheng. His research interest focuses on developing functional nanomaterials for biomedical applications. |
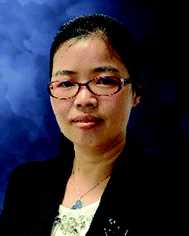
Dehong Hu
| Associate professor Dehong Hu received her MS degree in 2008 from Huazhong Agricultural University in chemistry and biological engineering. Then she worked at the Shenzhen Institutes of Advanced Technology, Chinese Academy of Sciences. Her research interests include functional nanomaterials for cancer imaging and therapy. |
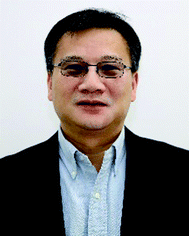
Xin Liu
| Professor Xin Liu received his MD (2006) degree in diagnostic radiology from Postgraduate Medical School of PLA General Hospital. He entered the Research Center for Cardiovascular MRI, Northwestern University, as a research associate. In 2008, he joined the Shenzhen Institutes of Advanced Technology, Chinese Academy of Sciences. His research interests include cardiovascular MRI and MRI-guided minimally invasive therapy. |
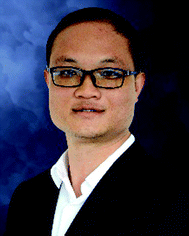
Zonghai Sheng
| Professor Zonghai Sheng received his PhD (2010) degree from Huazhong Agricultural University, China. Then, he worked as a postdoctoral fellow researcher from 2011 to 2013 at the Chinese Academy of Sciences. Since 2018, he has been a full professor at the Shenzhen Institutes of Advanced Technology, Chinese Academy of Sciences. His main research activities focus on the preparation and bioapplications of functional nanomaterials. |
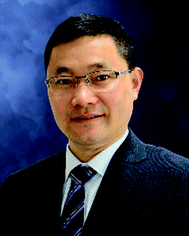
Hairong Zheng
| Professor Hairong Zheng is a deputy director of the Shenzhen Institutes of Advanced Technology, Chinese Academy of Sciences. He received his PhD from the University of Colorado at Boulder in 2006. Dr Zheng has published more than 160 peer-reviewed journal papers, and owned more than 110 ultrasound/MRI related patents in his research. Dr Zheng's research areas focus on multifunctional biomedical ultrasound and multimodality medical imaging systems. |
1. Introduction
Glioma is a typical type of malignant brain tumor that originates from glial cells. The World Health Organization (WHO) classifies gliomas into four different grades according to their growth potential and aggressiveness. Among them, glioblastoma (GBM) is the most aggressive malignant primary brain tumor which has poor prognosis, high risk of recurrence and mortality.1 Currently, clinical treatment of GBM generally includes surgery and postoperative adjuvant therapy, such as radiation therapy,2 chemotherapy,3 and immunotherapy.4 The infiltrative nature of GBM cells, however, presents a particular challenge for a neurosurgeon who is unable to precisely delineate the tumor–brain margin with experience, visual observation, and neuronavigation.5 Nowadays, various advanced intraoperative navigation technologies have been developed for glioma delineation, such as ultrasound (US),6 computed tomography (CT),7 magnetic resonance imaging (MRI),8 surface-enhanced Raman scattering (SERS),9 desorption electrospray ionization-mass spectrometry,10 and fluorescence imaging.11 However, maximizing tumor excision and minimizing postoperative neurological damage are significant challenges in glioma neurosurgery.
Photoacoustic (PA) imaging is an emerging imaging modality that demonstrates prominent potential for cancer surgery navigation.12 As a hybrid imaging technique, PA imaging is based on the PA effect, which capitalizes on acoustic wave detection instead of optical detection. Therefore, PA imaging generates high spatial resolution in the deep-tissue region.13–15 Moreover, endogenous chromophores, such as oxy-hemoglobin and deoxy-hemoglobin, can be applied as contrast agents for structural imaging of the cerebrovascular system and functional imaging of oxygen saturation.16 In recent years, PA image-guided surgery has evolved rapidly, leading to a variety of valuable discoveries.17–20 However, it is difficult to specifically visualize the tumor margin with endogenous contrast agents due to the ambiguous differences between normal and lesion tissues.21
Recently, with the development of nanobiotechnology, functional nanomaterials with strong optical absorbance and highly specific recognition elements have been widely adopted for PA molecular imaging.22–26 These functional nanomaterials typically consist of two kinds of nanomaterials, organic contrast agents, such as small-molecule-based nanomaterials (e.g., ICG-based and cy5.5-based) and conjugated polymer (CP) nanoparticles, and inorganic contrast agents, such as noble metal nanoparticles (e.g., gold nanorods, gold nanostars, and gold nanoshells), copper-based nanoparticles (e.g., CuS and Cu2−xSe), and other inorganic nanostructures (e.g., Fe@Fe3O4, carbon-based nanodots, and MoS2 nanosheets). They have played significant roles in improving the sensitivity and spatial resolution of glioma PA imaging.27 In this minireview, we summarize the recent exploration of functional nanomaterials for PA imaging of glioma from 2015 to 2019, and discuss the design, preparation and application of organic and inorganic functional nanomaterials for PA imaging of glioma in pre-clinical models (Table 1). Correspondingly, further challenges and perspectives in this field are proposed.
Table 1 Functional nanomaterials as PA contrast agents for glioma imaging
Organic-based functional nanomaterials |
Small-molecule-based nanostructures |
Den-RGD/CGS/Cy5.5, RGD-CR780-PEG5K, MB-ICG–BSA, ICG-loaded liposome, holo-Tf–ICG, melittin-RADA32-ICG, lipo@HRP & ABTS, PDI-based NPs, TB1-RGD, MoS2–ICG, Fe3O4–ICG |
Polymer-based nanostructures |
P1 RGD, CP3-RGD, PDPPTBZ, CP, |
Inorganic-based functional nanomaterials |
Noble metal nanomaterials |
Au@MIL-88, Au NRs@SiO2, p53/Au@PDM/Fe3O4, gold nanostars, SPIO@Au |
Copper-based nanostructures |
BSA–CuS, Cu2−xSe, HCu |
Other inorganic nanostructures |
Fe@Fe3O4, carbon-based nanodots, MoS2 nanosheets |
2. Organic functional nanomaterials
Organic nanomaterials customarily retain good biocompatibility and metabolism.21,28,29 At present, plenty of organic nanomaterials as PA contrast agents have been developed.30–35 In this section, we introduce two kinds of nanomaterials, small-organic-molecule based nanomaterials and conjugated polymer nanoparticles, for PA imaging of glioma.
2.1 Small-molecule-based nanoparticles
Several types of organic dyes for PA imaging have attracted intensive attention due to their abundant sources, excellent biocompatibility, bright future for clinical translation, and designable carriers. Among them, indocyanine green (ICG) is a U.S. Food and Drug Administration (FDA)-approved dye for cancer localization, surgical margin evaluation, metastatic lymph node mapping, and many other applications in clinics.36–42 Recent reports show that ICG could be an impressive PA contrast agent because of its high optical absorption in the near-infrared (NIR) region. However, it is difficult to adopt ICG for in vivo PA imaging due to its inherent shortcomings, such as water instability, poor active targeting and short blood circulation.43,44 To overcome these drawbacks, various nanocarriers including hydrogels45 and inorganic46,47 and organic nanoparticles48–50 have been developed to improve its in vivo performance. For instance, Jin et al. designed a novel biocompatible hydrogel containing melittin in the peptide hydrogel backbone and ICG in the hydrogel matrix with nearly 100% loading efficiency.45 The ICG-based hydrogel demonstrated stronger PA signals in the tumor region than that of the free ICG-treated group, indicating the superiority of the ICG hydrogel for in vivo PA imaging. Our group has also developed several nanocarriers such as biomimetic liposomes,48 holo-transferrin,49 bovine serum albumin (BSA),50 and 2-dimensional MoS2 nanosheets,46 for efficiently enhanced PA imaging with ICG. The biomimetic ICG-liposomes demonstrated a remarkable PA contrast enhancement as compared to the conventional liposome nanomedicine and free ICG, due to their active homotypic targeting property and long circulation time.48 Similarly, we proposed a one-step strategy for the synthesis of active-targeting ICG-based nanoassemblies using the hydrophobic interaction and hydrogen bonds between holo-Tf and ICG (Fig. 1a and b).49 The holo-Tf–ICG with a diameter of about 10 nm could selectively bind with glioma cells, resulting in highly promoted PA signals with increasing time (Fig. 1c and d). Quantitative results indicated that the PA signal intensity in the glioma site of the holo-transferrin–ICG nanoassembly-treated group was 4.6-fold stronger than that of the free ICG-treated group at 24 h post-injection (Fig. 1e). The same tendency of PA imaging of glioma was also observed in MoS2–ICG nanostructures.46 This suggested that highly biocompatible and biodegradable nanocarriers in the ICG matrix have extraordinary advantages for in vivo PA imaging of glioma compared to free ICG. Other organic dyes were involved in designing organic nanoparticles for PA imaging of glioma. For instance, Liu and co-workers developed cy5.5-based functional nanoparticles, featuring strong NIR absorption and specific glioblastoma-targeting ability.51 In addition to commercialized dyes, rational design and preparation of organic molecule-loaded nanoparticles as PA contrast agents have attracted much attention in the field of PA imaging. For example, Fan et al. reported an efficient PA contrast agent via enveloping a small organic molecule (perylene-3,4,9,10-tetracarboxylic diimide, PDI) with amphiphilic molecules for deep brain tumor imaging.52 The PDI-based organic NPs with high biocompatibility and photostability revealed an outstanding PA imaging function. An obvious PA signal enhancement at the brain tumor tissue can be observed after 1 day tail-vein intravenous injection; in contrast, no signal was detected in the control group. The authors found that overly strong absorption of the PA nanoprobes may reduce the generation of PA signals in the deep tissue. Therefore, it is important to select contrast agents with appropriate absorption or control their concentrations in the lesion for obtaining optimal PA images of the deep tumor area. We designed novel aggregation-induced-emission molecule-based nanomaterials linked by a cyclic arginine–glycine–aspartic (c-RGD) peptide for in vivo PA imaging of brain tumor.53 It was found that the TB1-RGD nanoparticles possessed excellent cancer cell targeting ability. We observed that the PA signals were gradually enhanced after the administration of the TB1-RGD nanoparticles, achieving the maximum at 24 h post-injection. Furthermore, the tumor region was clearly visualized as deep as 2.0 mm, indicating that the as-synthesized TB1-RGD nanoparticles could be used as an excellent contrast agent for PA imaging with precise depth information. Smart molecules that can respond to the tumor microenvironment were applied for glioma PA imaging. Chen et al. designed H2O2-responsive liposomes composed of horseradish peroxidase (HRP) and its substrate, 2,2′-azino-bis(3-ethylbenzothiazoline-6-sulfonic acid) (ABTS).54 It produced strong NIR absorbance in the tumor microenvironment by HRP-mediated catalytic oxidation of ABTS for changing from colourless to dark blue, enabling the PA imaging of orthotopic brain gliomas.
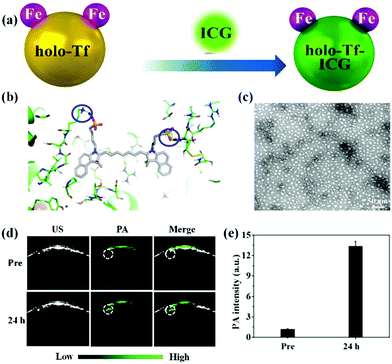 |
| Fig. 1 (a) Schematic illustration of the preparation of holo-Tf–ICG nanoassemblies; (b) three-dimensional pattern of the holo-Tf–ICG complex; (c) representative TEM image of the holo-Tf–ICG nanoassemblies; (d) PA images of orthotopic-U87 brain tumors pre- and post-holo-Tf–ICG injection; (e) quantitative analysis of PA imaging. Reproduced with permission from ref. 49. Copyright© 2017, American Chemical Society. | |
2.2 Conjugated polymer nanoparticles
Conjugated polymer (CP) nanoparticles, composed of CPs with delocalized π-electron backbones, have emerged as a promising class of functional nanomaterials for biomedical applications owing to their large absorption coefficient, excellent photostability, and distinct biocompatibility.23,30,31,55–61 Moreover, the absorption wavelength of nanoparticles can be tuned by adjusting the molecular structure of the CPs and their particle size.62–64 By virtue of these superior photoelectronic properties, different CP nanoparticles have been introduced as contrast agents for glioma PA imaging. For example, Yang and co-workers designed CP nanoparticles with a large mass extinction coefficient of 43 mL mg−1 cm−1 at 1064 nm.65 In a phantom experiment, the CP nanoparticles allowed achieving excellent PA contrast and deep imaging depth at a depth of 4 cm. In tumor-bearing mice models, they further demonstrated that CP nanoparticles enabled realizing the PA imaging of glioma at a depth of 3.8 mm below the skull. Recently, we explored a variety of CP nanoparticles obtained by the nanoprecipitation method for PA imaging of glioma at 1064 nm (Fig. 2a).66 These benzodithiophene (BDT)-based CP nanoparticles generally exhibited a spherical shape with diameters around 50 nm and a broad absorption spectrum (Fig. 2b and c). We first observed that CP nanoparticle-based PA imaging at 1064 nm was superior to that of the condition at 808 nm in penetration depth and signal-to-background ratio. In orthotopic tumor-bearing mice models, the PA signals increased with time continuously after administration of the BDT-based CP nanoparticles, reaching a maximum at 24 h post-injection (Fig. 2d–f). The PA signal intensity increased 94 times as compared to the initial PA signal (Fig. 2h). The imaging depth was measured to be 3.4 mm below the scalp and skull, which was well consistent with the MRI result (Fig. 2g). Further, a c-RGD peptide for integrin targeting was subsequently linked on the BDT-based CP nanoparticles, resulting in active targeting PA contrast agents.67 In the cell uptake experiment, c-RGD modified BDT-based CP nanoparticles exhibited a 3.5-fold higher PA signal than that of CP nanoparticles. The signal-to-noise ratio of glioma PA imaging in the c-RGD NPs-treated group was about 1.5-fold higher than that of the passive-targeting group in mice models, suggesting the advantage of the c-RGD-modified CP nanoparticles. Subsequently, we further investigated the effect of electron acceptor (A)–electron donor (D) strength on their absorption, emission, and extinction coefficient.68 It was found that increasing the D–A strength was essential to synthesize excellent CP-based PA contrast agents. The engineering concept is of great significance for the design and preparation of CP-based functional nanomaterials for PA imaging of glioma.
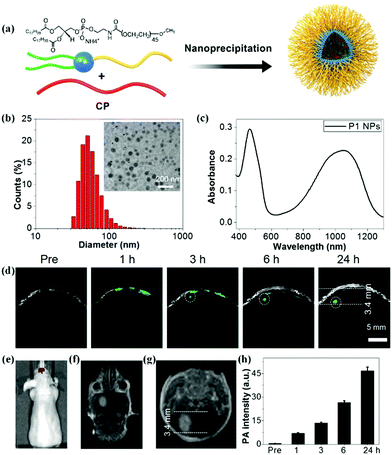 |
| Fig. 2 (a) Schematic of the construction of CP nanoparticles; (b) diameter of the BDT-based nanoparticles measured by dynamic light scattering and a TEM image; (c) UV-vis spectrum of BDT-based nanoparticles dispersed in water; (d) B-scan PA images of the brain tumor before and after nanoparticle injection; (e) in vivo bioluminescence image of a brain tumor; (f) coronal and (g) transverse section views of contrast-enhanced T1-weight MR imaging of a U87 tumor-bearing mouse; (h) PA signals over time in the tumor region. Reproduced with permission from ref. 66. Copyright© 2017, Royal Society of Chemistry. | |
3. Inorganic functional nanomaterials
Inorganic nanomaterials with different composition, morphology, and size have a significant effect on the absorption wavelength and intensity, leading to various PA performances. In this section, we mainly focus on several typical inorganic nanomaterials including noble metal nanomaterials (gold nanorods, gold nanostars, and gold nanoshells), semiconductor nanomaterials (Cu2−xSe and CuS), Fe-based nanoprobes (Fe@Fe3O4), and 2-dimensional transition metals (MoS2) as PA contrast agents for glioma imaging.
3.1 Noble metal nanomaterials
Noble metal nanomaterials including gold nanorods, palladium nanoplates, silver nanoplates, etc. exhibited tuneable surface plasmon resonance absorption in the NIR window.69–71 The unique performance combined with their excellent biocompatibility allows their use as PA contrast agents for biomedical imaging.72 In general, controllable synthesis of noble metal nanomaterials is accomplished by the cetyltrimethyl ammonium bromide (CTAB) ligand, a cationic surfactant with high cytotoxicity.73 Thus, ligand exchange or surface coating strategies are usually employed for improving their biocompatibility. Recently, Shang et al. reported a strategy to reduce the cytotoxicity of gold nanorods by ligand exchange and controllable growth of metal–organic-framework (MOF) shells (Fig. 3a).74 11-Mercaptoundecanoic acid (MUA) molecules were applied for the ligand exchange reaction to obtain carboxyl group-terminated gold nanorods for further growth of MOF shells. The biocompatible gold nanorod/MOF nanoparticles (Au@MIL-88) demonstrated an intense absorption in the NIR region (Fig. 3b). As shown in elemental mapping results (Fig. 3c), gold nanorods homogeneously located in the core region and the other elements distributed throughout the nanostructure. The authors evaluated the PA contrast ability of the Au@MIL-88 nanomaterials in orthoptic glioma models in mice (Fig. 3d). The PA signal in the glioma region dramatically increased after intravenous injection of Au@MIL-88, indicating its excellent PA imaging with high contrast and deep penetration depth (Fig. 3e). Additionally, a silica-coating approach for improving the biocompatibility of gold nanorods was proposed by Xu's group.75,76 They further assessed the PA properties of gold nanorods@SiO2 nanostructures and achieved contrast-enhanced PA imaging in a subcutaneous glioma-bearing mouse model.
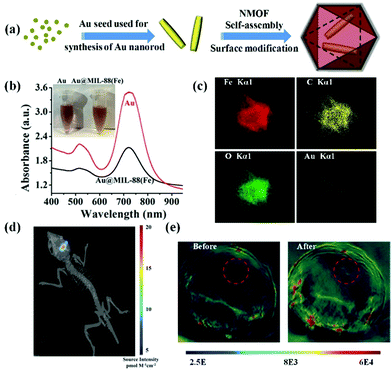 |
| Fig. 3 (a) Schematic illustration of the preparation of Au@MIL-88 nanoparticles; (b) UV-vis spectra and photograph (inset) of Au nanorods and Au@MIL-88; (c) STEM-EDS mapping of Au@MIL-88 nanoparticles. (d) Bioluminescent imaging of glioma; (e) in vivo PA imaging of tumors in mice before and 12 h after i.v. injection with Au@MIL-88(Fe) (2 mg mL−1, 200 μL). Reproduced with permission from ref. 74. Copyright© 2016 Wiley-VCH Verlag GmbH & Co. KGaA, Weinheim. | |
Gold nanostars with multibranched nanostructures exhibit tuneable surface plasmon resonance absorption in the NIR region, with potential as contrast agents for PA imaging. The size, morphology, and monodispersity of gold nanostars can be tailored by varying the concentration/ratio of the reactants, and the reaction temperature.77,78 Moritz F. Kircher et al. successfully prepared gold nanostars/SiO2 nanostructures as contrast agents for PA image-guided glioma surgery.79 They exhibited excellent penetration depth, covering the entire volume of the mouse brain with a sub-millimeter spatial resolution. A star-shaped gold nanostructure demonstrated better photostability than a rod-shaped one under pulsed laser irradiation. More interestingly, the gold nanostars exhibited high accumulation in the brain tumor region, leading to highly sensitive PA imaging with a desirable signal-to-background ratio.
Gold nanoshells are composed of a dielectric core and a gold shell.80 The absorption wavelength of the nanoshell can be tuned from visible to the NIR region by controlling the relative size of the inner core and the outer shell layer, making them excellent PA contrast agents for biomedical applications. Qiao and co-workers successfully synthesized a magnetic gold nanoshell for PA imaging of brain tumor via carotid artery injection.81 They utilized mesenchymal stem cells (MSC) as active-targeting carriers for efficient delivery of the gold nanoshell. It was found that the magnetic gold nanoshell exhibited no adverse effects on differentiation or migration of the MSC. Furthermore, the PA signal in the brain tumor region dramatically increased at 72 h post-injection of the MSC-magnetic gold nanoshell.
3.2 Copper-based nanomaterials
Copper-based nanomaterials have attracted intense attention ascribed to their tuneable physical, chemical, and/or optical properties.82–87 Like gold-based nanomaterials, plasmonic properties can also be observed in copper-based nanostructures, resulting in tunable optical absorption in the NIR windows.88 Moreover, compared to gold-based nanomaterials with a characteristic absorption peak in the first NIR region (NIR-I: 700–950 nm), copper-based nanomaterials usually show a strong absorption peak over 1000 nm, defined as the second NIR window (NIR-II: 1000–1700 nm), which has less tissue absorption and scattering.89–94 Therefore, recently, several copper-based nanomaterials were designed as PA contrast agents by our group and others for glioma imaging. For example, Gao et al. developed BSA-assisted synthesis of CuS nanoparticles with tuneable absorption in aqueous solution by adjusting the stoichiometric ratios of copper and sulfide precursors.95 The BSA-modified CuS nanoparticles exhibited a triangular shape with a diameter of about 15 nm, demonstrating excellent stability under diverse conditions including different pH values (pH = 4–10), strong ionic strength and high temperature. Further, the triangular CuS nanoparticles were successfully employed as PA contrast agents for glioma imaging in a subcutaneous tumor-bearing mouse model. And on this basis, we further constructed an analog of CuS nanoparticles, Cu2−xSe nanoparticles, with an ultra-small particle size of 3.3 ± 0.4 nm and strong absorption in the NIR-II window.96 The miniature nanoparticles were able to cross the blood–brain barrier (BBB)/blood–brain tumor barrier (BBTB) and specifically accumulate in the glioma region under the treatment of focused ultrasound (FUS), a physical strategy for opening the BBB temporarily. After the ultrasound treatment, the PA signal at the tumor location increased to be about 3 times higher than that of the control group, indicating the amazing effect of FUS for BBB opening. Moreover, we further demonstrated that Cu2−xSe nanoparticles could also be integrated on hollow mesoporous organosilica nanoparticles (HCu) as theranostic agents for PA image-guided glioma therapy (Fig. 4a).97 The absorbance and in vitro PA signals of HCu nanoparticles linearly increased with the Cu concentration, indicating their concentration-dependent properties (Fig. 4b and c). A significant PA contrast enhancement was observed in the glioma beneath the skull after intravenous injection of HCu plus FUS-induced BBB opening. However, the PA signals in the control without FUS treatment had no variation, confirming that FUS is a promising strategy to deliver PA contrast agents and/or drugs for orthotopic brain tumor imaging and/or therapy (Fig. 4d).
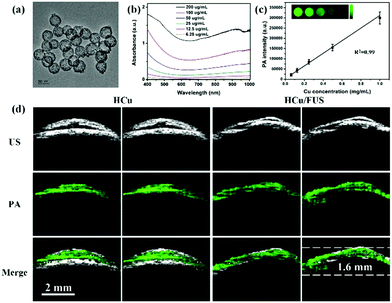 |
| Fig. 4 (a) TEM image of HCu; (b) UV-vis absorbance spectra of HCu aqueous solution at different concentrations; (c) the linear relationship between PA intensity and Cu concentration (inset: PA images of agar gel with different Cu concentrations); (d) US, PA, and the overlay images of orthotopic brain tumors acquired before and after administration of HCu without/with FUS-induced BBB opening. Reproduced with permission from ref. 97, an open-access article by the authors. Published by Wiley-VCH Verlag GmbH & Co. KGaA, Weinheim. | |
3.3 Other inorganic contrast agents for glioma PA imaging
Besides the above-mentioned nanomaterials, scientists have prepared other nanostructures as PA contrast agents for glioma PA imaging including Fe-based nanoprobes, 2-dimensional transition metal dichalcogenides, and carbon-based nanomaterials. Zhou and co-workers synthesized Fe@Fe3O4 nanoparticles with a moderate absorbance in the NIR region, exhibiting enhanced PA imaging ability.98 A c-RGD peptide was conjugated with the PEGylated Fe@Fe3O4 nanoparticles to achieve the active tumor targeting ability. The PA signal of the αvβ3-positive U87MG glioma area at 6 h post-injection was 2.2 times higher than that of the pre-injection group and increased by 2.2-fold compared to that of the blocking group, indicating their excellent active-targeting PA imaging ability. Carbon-based nanomaterials have been widely used in biological areas due to their rich surface functional groups, tunable optical properties, and good biocompatibility.99–104 Qian et al. fabricated biocompatible carbon-based nanodots with high crystallinity and uniform small size of 6–8 nm, which specifically accumulated in glioma for PA imaging.105 Meanwhile, 2-dimensional transition metal dichalcogenides, MoS2 nanosheets, were introduced as PA contrast agents by our group due to their distinctive physical and chemical properties.106 As demonstrated in Fig. 5a, MoS2 nanosheets with different layers including single-layer (S-MoS2), few-layer (F-MoS2), and multi-layer (M-MoS2) nanosheets were obtained by albumin-assisted exfoliation. It was proved that the layer number of the nanosheets had a great effect on the generation of PA signals. S-MoS2 nanosheets exhibited the strongest absorption and best PA imaging ability as compared to the F-MoS2 and M-MoS2 nanosheets, which were further applied for in vivo imaging (Fig. 5b and c). In an orthotopic brain tumor model, the tumor location could be clearly observed in the PA images of the whole brain after S-MoS2 administration, (Fig. 5d). More importantly, an enhanced PA signal was visualized in the brain tumor at 1 h after intravenous injection. At 24 h post-injection, the PA signal in the brain tumor area was about 36 times higher than that of the pre-injection group. The imaging depth was up to 1.8 mm below the skull, indicating an excellent PA imaging ability on brain tumor (Fig. 5e).
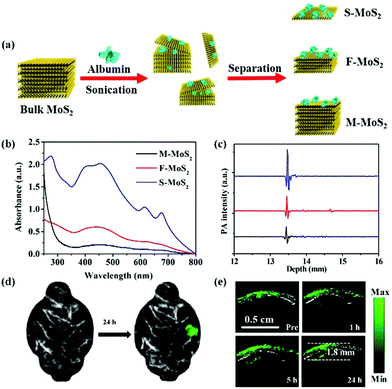 |
| Fig. 5 (a) Schematic illustration of the preparation of MoS2 nanosheets with various layered nanostructures by albumin-assisted exfoliation; (b) the UV-vis-NIR absorption spectra of MoS2 with different layers at the same concentration, (c) PA signals of S-MoS2, F-MoS2, and M-MoS2 with the same optical absorbance at a wavelength of 675 nm; (d) PA images of the glioma area before and after injection of S-MoS2 nanosheets exhibiting blood vessels in gray and glioma in green color; (e) B-scan PA images of the brain tumor region obtained before (Pre) and 1, 5, and 24 h after injection of S-MoS2 nanosheets. Reproduced with permission from ref. 106. Copyright© 2016 Wiley-VCH Verlag GmbH & Co. KGaA, Weinheim. | |
4. Summary and perspectives
PA imaging, a hybrid imaging technique, has shown great potential for image-guided glioma surgery in pre-clinics. Since the imaging resolution and depth are directly affected by the contrast agents, over recent years, tremendous efforts have been devoted to the development of PA contrast agents. In this minireview, we summarize the recent advances in functional nanomaterials as contrast agents for PA imaging of glioma. Different functional nanomaterials including organic (small-molecule-based nanomaterials and polymer nanoparticles) and inorganic (noble metal nanoparticles, copper-based nanoparticles, and other inorganic nanostructures) ones are discussed via presenting various typical examples. While the development of functional nanomaterials has been evidenced from the above progress, nevertheless, there are still certain challenges remaining to be addressed. The clinical application of functional nanomaterials for PA imaging of glioma is still premature. Here, we propose some issues of clinical concern which are indispensable to be addressed in future studies.
4.1 Biosafety
Although several functional nanomaterials have been explored to enhance the PA contrast, the in vivo biosafety of these nanomaterials, including biodistribution, metabolism, and acute/chronic toxicity, has rarely been systematically studied. Therefore, the biocompatibility and biosafety of nanomaterials in large animals and even non-human primates should be carefully investigated and tested before the clinical application of the functional nanomaterials. Given the fact that FDA-approved ICG has been applied in clinics, the development of ICG-based functional nanomaterials should be a promising direction.
4.2 Imaging window
It is difficult to obtain PA images through the scalp and skull, limiting the applications of PA imaging of glioma. In biological tissue, there are two distinct spectral windows, NIR-I (650–900 nm) and NIR-II (1000–1700 nm). Contrast agents with NIR absorptions have been developed to overcome this issue. Within NIR-II, the 1064 nm excitation using a stable nanosecond laser can generate the lowest PA background noise due to the low optical absorption of deoxygenated hemoglobin. Moreover, compared with NIR-I imaging, PA imaging in the NIR-II shows higher tissue penetration depth. Therefore, the development of NIR-II functional nanomaterials as PA contrast agents can significantly improve the sensitivity, resolution and imaging depth of PA molecular imaging.
4.3 Crossing the BBB/BBTB and targeting strategies for glioma
Different from other tumors, glioma has a unique physiological structure of BBB/BBTB, which greatly prevents functional nanomaterials from entering the brain tissue and targeting glioma cells. Therefore, actively targeted ligands including peptides, proteins, antibodies, and nucleic acids should be considered for modifying the functional nanomaterials. In particular, biomimetic nanostructures with appropriate size, charge, and surface modification should be developed to improve their performance of crossing the BBB/BBTB and accumulating in the glioma. Additionally, FUS, a physical method, can also efficiently deliver functional nanomaterials into glioma cells for enhancing the PA contrast.
4.4 Imaging system
Several commercially available PA imaging systems have been developed including the Vevo LAZR system, LOIS 3D pre-clinical mouse imaging system, multispectral optoacoustic tomography system, Nexus 128 3D imaging system, and LED-based photoacoustic imaging (PLED-PAI).107 Each of these imaging systems has advantages and exhibits great promise for functional nanomaterial-based PA imaging. Some groups attempted to set up their PA imaging system for brain imaging, which was generally thwarted due to the lack of nanomaterial knowledge and clinical background. Therefore, the development of PA systems for real-time, dynamic, high resolution and 3D brain imaging by interdisciplinary fusion is an important development direction in the near future.
Conflicts of interest
There are no conflicts to declare.
Acknowledgements
This work was supported by the Major State Basic Research Development Program of China (973 Program) (2015CB755500), the National Key Research and Development Program of China: Scientific and Technological Innovation Cooperation of Mainland and Macao (2017YFE0120000), the Natural Science Foundation of China (81571745, 91859117, 81771906), the Science and Technology Key Project of Shenzhen (JCYJ20160229200902680), Shenzhen Key Laboratory of Ultrasound Imaging and Therapy (ZDSYS201802061806314), Shenzhen Double Chain Grant [2018]256, the Natural Science Foundation of Guangdong Province (2014A030312006), and the China Postdoctoral Science Foundation (2019M653129).
Notes and references
- A. Omuro and L. M. DeAngelis, JAMA, 2013, 310, 1842–1850 CrossRef CAS PubMed.
- B. G. Baumert, M. E. Hegi, M. J. van den Bent, A. von Deimling, T. Gorlia, K. Hoang-Xuan, A. A. Brandes, G. Kantor, M. J. B. Taphoorn, M. B. Hassel, C. Hartmann, G. Ryan, D. Capper, J. M. Kros, S. Kurscheid, W. Wick, R. Enting, M. Reni, B. Thiessen, F. Dhermain, J. E. Bromberg, L. Feuvret, J. C. Reijneveld, O. Chinot, J. M. M. Gijtenbeek, J. P. Rossiter, N. Dif, C. Balana, J. Bravo-Marques, P. M. Clement, C. Marosi, T. Tzuk-Shina, R. A. Nordal, J. Rees, D. Lacombe, W. P. Mason and R. Stupp, Lancet Oncol., 2016, 17, 1521–1532 CrossRef CAS PubMed.
- L. A. Stewart, Lancet, 2002, 359, 1011–1018 CrossRef CAS.
- A. S. Achrol, R. C. Rennert, C. Anders, R. Soffietti, M. S. Ahluwalia, L. Nayak, S. Peters, N. D. Arvold, G. R. Harsh, P. S. Steeg and S. D. Chang, Nat. Rev. Dis. Primers, 2019, 5, 5 CrossRef PubMed.
- J. E. Villanueva-Meyer, M. C. Mabray and S. Cha, Neurosurgery, 2017, 81, 397–415 CrossRef PubMed.
- M. A. Pino, A. Imperato, I. Musca, R. Maugeri, G. R. Giammalva, G. Costantino, F. Graziano, F. Meli, N. Francaviglia, D. G. Iacopino and A. Villa, Brain Sci., 2018, 8, 202 CrossRef PubMed.
- E. Uhl, S. Zausinger, D. Morhard, T. Heigl, B. Scheder, W. Rachinger, C. Schichor and J.-C. Tonn, Oper. Neurosurg., 2009, 64, 231–239 CrossRef PubMed.
- C. Senft, A. Bink, K. Franz, H. Vatter, T. Gasser and V. Seifert, Lancet Oncol., 2011, 12, 997–1003 CrossRef PubMed.
- X. Gao, Q. Yue, Z. Liu, M. Ke, X. Zhou, S. Li, J. Zhang, R. Zhang, L. Chen, Y. Mao and C. Li, Adv. Mater., 2017, 29, 1603917 CrossRef PubMed.
- V. Pirro, C. M. Alfaro, A. K. Jarmusch, E. M. Hattab, A. A. Cohen-Gadol and R. G. Cooks, Proc. Natl. Acad. Sci. U. S. A., 2017, 114, 6700 CAS.
- W. Stummer, U. Pichlmeier, T. Meinel, O. D. Wiestler, F. Zanella and H.-J. Reulen, Lancet Oncol., 2006, 7, 392–401 CrossRef CAS PubMed.
- K. S. Valluru, K. E. Wilson and J. K. Willmann, Radiology, 2016, 280, 332–349 CrossRef PubMed.
- L. V. Wang and S. Hu, Science, 2012, 335, 1458–1462 CrossRef CAS PubMed.
- L. Nie and X. Chen, Chem. Soc. Rev., 2014, 43, 7132–7170 RSC.
- L. V. Wang and H. Song, Science, 2012, 335, 1458–1462 CrossRef CAS PubMed.
- X. Wang, Y. Pang, G. Ku, X. Xie, G. Stoica and L. V. Wang, Nat. Biotechnol., 2003, 21, 803–806 CrossRef CAS PubMed.
- S. Mallidi, G. P. Luke and S. Emelianov, Trends Biotechnol., 2011, 29, 213–221 CrossRef CAS PubMed.
- L. Xi, S. R. Grobmyer, L. Wu, R. Chen, G. Zhou, L. G. Gutwein, J. Sun, W. Liao, Q. Zhou and H. Xie, Opt. Express, 2012, 20, 8726–8731 CrossRef PubMed.
- M. T. Graham, J. Y. Guo and M. A. L. Bell, Proc. SPIE, 2019, 10868, 108680R Search PubMed.
- D. Lee, J. Kim, J. Y. Kim, J. W. Baik and C. Kim, Proc. SPIE, 2019, 10864, 108640C Search PubMed.
- J. Weber, P. C. Beard and S. E. Bohndiek, Nat. Methods, 2016, 13, 639 CrossRef CAS PubMed.
- A. De La Zerda, C. Zavaleta, S. Keren, S. Vaithilingam, S. Bodapati, Z. Liu, J. Levi, B. R. Smith, T.-J. Ma, O. Oralkan, Z. Cheng, X. Chen, H. Dai, B. T. Khuri-Yakub and S. S. Gambhir, Nat. Nanotechnol., 2008, 3, 557 CrossRef CAS PubMed.
- K. Pu, A. J. Shuhendler, J. V. Jokerst, J. Mei, S. S. Gambhir, Z. Bao and J. Rao, Nat. Nanotechnol., 2014, 9, 233 CrossRef CAS PubMed.
- Y.-S. Chen, Y. Zhao, S. J. Yoon, S. S. Gambhir and S. Emelianov, Nat. Nanotechnol., 2019, 14, 465–472 CrossRef CAS PubMed.
- P. Huang, J. Lin, W. Li, P. Rong, Z. Wang, S. Wang, X. Wang, X. Sun, M. Aronova, G. Niu, R. D. Leapman, Z. Nie and X. Chen, Angew. Chem., 2013, 125, 14208–14214 CrossRef.
- S. K. Maji, S. Sreejith, J. Joseph, M. Lin, T. He, Y. Tong, H. Sun, S. W.-K. Yu and Y. Zhao, Adv. Mater., 2014, 26, 5633–5638 CrossRef CAS PubMed.
- M. F. Kircher, A. de la Zerda, J. V. Jokerst, C. L. Zavaleta, P. J. Kempen, E. Mittra, K. Pitter, R. Huang, C. Campos, F. Habte, R. Sinclair, C. W. Brennan, I. K. Mellinghoff, E. C. Holland and S. S. Gambhir, Nat. Med., 2012, 18, 829 CrossRef CAS PubMed.
- D. Cui, C. Xie and K. Pu, Macromol. Rapid Commun., 2017, 38, 1700125 CrossRef PubMed.
- Q. Fu, R. Zhu, J. Song, H. Yang and X. Chen, Adv. Mater., 2019, 31, 1805875 Search PubMed.
- Y. Lyu, C. Xie, S. A. Chechetka, E. Miyako and K. Pu, J. Am. Chem. Soc., 2016, 138, 9049–9052 CrossRef CAS PubMed.
- K. Pu, A. J. Shuhendler and J. Rao, Angew. Chem., Int. Ed., 2013, 52, 10325–10329 CrossRef CAS PubMed.
- Z. Sheng, D. Hu, M. Zheng, P. Zhao, H. Liu, D. Gao, P. Gong, G. Gao, P. Zhang, Y. Ma and L. Cai, ACS Nano, 2014, 8, 12310–12322 CrossRef CAS PubMed.
- C. Yin, G. Wen, C. Liu, B. Yang, S. Lin, J. Huang, P. Zhao, S. H. D. Wong, K. Zhang, X. Chen, G. Li, X. Jiang, J. Huang, K. Pu, L. Wang and L. Bian, ACS Nano, 2018, 12, 12201–12211 CrossRef CAS PubMed.
- Y. Zhang, M. Jeon, L. J. Rich, H. Hong, J. Geng, Y. Zhang, S. Shi, T. E. Barnhart, P. Alexandridis, J. D. Huizinga, M. Seshadri, W. Cai, C. Kim and J. F. Lovell, Nat. Nanotechnol., 2014, 9, 631–638 CrossRef CAS PubMed.
- Y. Liu, S. Wang, Y. Ma, J. Lin, H. Y. Wang, Y. Gu, X. Chen and P. Huang, Adv. Mater., 2017, 29, 1606129 CrossRef PubMed.
- J. T. Alander, I. Kaartinen, A. Laakso, T. Pätilä, T. Spillmann, V. V. Tuchin, M. Venermo and P. Välisuo, Int. J. Biomed. Imaging, 2012, 940585 Search PubMed.
- A. L. Vahrmeijer, M. Hutteman, J. R. van der Vorst, C. J. H. van de Velde and J. V. Frangioni, Nat. Rev. Clin. Oncol., 2013, 10, 507 CrossRef CAS PubMed.
- M. V. Marshall, J. C. Rasmussen, I. C. Tan, M. B. Aldrich, K. E. Adams, X. Wang, C. E. Fife, E. A. Maus, L. A. Smith and E. M. Sevick-Muraca, Open Surg. Oncol. J., 2010, 2, 12 CrossRef.
- M. Koch and V. Ntziachristos, Annu. Rev. Med., 2016, 67, 153–164 CrossRef CAS PubMed.
- P. Karol, M. Dawid, R. Young-Soo, N. Piotr, H. Michael and M. Pawel, Cancer, 2011, 117, 4812–4822 CrossRef PubMed.
- E. A. Te Velde, T. Veerman, V. Subramaniam and T. Ruers, Eur. J. Surg. Oncol., 2010, 36, 6–15 CrossRef CAS PubMed.
- J. C. Rasmussen, I. C. Tan, M. V. Marshall, C. E. Fife and E. M. Sevick-Muraca, Curr. Opin. Biotechnol., 2009, 20, 74–82 CrossRef CAS PubMed.
- A.-K. Kirchherr, A. Briel and K. Mäder, Mol. Pharmaceutics, 2009, 6, 480–491 CrossRef CAS PubMed.
- Z. Sheng, D. Hu, M. Xue, M. He, P. Gong and L. Cai, Nano-Micro Lett., 2013, 5, 145–150 CrossRef.
- H. Jin, G. Zhao, J. Hu, Q. Ren, K. Yang, C. Wan, A. Huang, P. Li, J.-P. Feng, J. Chen and Z. Zou, ACS Appl. Mater. Interfaces, 2017, 9, 25755–25766 CrossRef CAS PubMed.
- C. Liu, J. Chen, Y. Zhu, X. Gong, R. Zheng, N. Chen, D. Chen, H. Yan, P. Zhang, H. Zheng, Z. Sheng and L. Song, Nano-Micro Lett., 2018, 10, 48 CrossRef PubMed.
- J. P. Thawani, A. Amirshaghaghi, L. Yan, J. M. Stein, J. Liu and A. Tsourkas, Small, 2017, 13, 1701300 CrossRef PubMed.
- Y. Jia, Z. Sheng, D. Hu, F. Yan, M. Zhu, G. Gao, P. Wang, X. Liu, X. Wang and H. Zheng, Biomater. Sci., 2018, 6, 1546–1555 RSC.
- M. Zhu, Z. Sheng, Y. Jia, D. Hu, X. Liu, X. Xia, C. Liu, P. Wang, X. Wang and H. Zheng, ACS Appl. Mater. Interfaces, 2017, 9, 39249–39258 CrossRef CAS PubMed.
- D. Hu, Z. Sheng, M. Zhu, X. Wang, F. Yan, C. Liu, L. Song, M. Qian, X. Liu and H. Zheng, Theranostics, 2018, 8, 410–422 CrossRef CAS PubMed.
- L. Liu, Q. Chen, L. Wen, C. Li, H. Qin and D. Xing, Adv. Funct. Mater., 2019, 29, 1808601 CrossRef.
- Q. Fan, K. Cheng, Z. Yang, R. Zhang, M. Yang, X. Hu, X. Ma, L. Bu, X. Lu, X. Xiong, W. Huang, H. Zhao and Z. Cheng, Adv. Mater., 2015, 27, 843–847 CrossRef CAS PubMed.
- Z. Sheng, B. Guo, D. Hu, S. Xu, W. Wu, W. H. Liew, K. Yao, J. Jiang, C. Liu, H. Zheng and B. Liu, Adv. Mater., 2018, 30, 1800766 CrossRef PubMed.
- Q. Chen, C. Liang, X. Sun, J. Chen, Z. Yang, H. Zhao, L. Feng and Z. Liu, Proc. Natl. Acad. Sci. U. S. A., 2017, 114, 5343–5348 CrossRef CAS PubMed.
- K. Li and B. Liu, Chem. Soc. Rev., 2014, 43, 6570–6597 RSC.
- Q. Miao, Y. Lyu, D. Ding and K. Pu, Adv. Mater., 2016, 28, 3662–3668 CrossRef CAS PubMed.
- J. Liu, J. Geng, L.-D. Liao, N. Thakor, X. Gao and B. Liu, Polym. Chem., 2014, 5, 2854–2862 RSC.
- L. Cui and J. Rao, Wiley Interdiscip. Rev.: Nanomed. Nanobiotechnol., 2017, 9, e148 Search PubMed.
- Y. Jiang and K. Pu, Small, 2017, 13, 1700710 CrossRef PubMed.
- T. Yang, L. Liu, Y. Deng, Z. Guo, G. Zhang, Z. Ge, H. Ke and H. Chen, Adv. Mater., 2017, 29, 1700487 CrossRef PubMed.
- K. Pu, J. Mei, J. V. Jokerst, G. Hong, A. L. Antaris, N. Chattopadhyay, A. J. Shuhendler, T. Kurosawa, Y. Zhou, S. S. Gambhir, Z. Bao and J. Rao, Adv. Mater., 2015, 27, 5184–5190 CrossRef CAS PubMed.
- J. Zhang, C. Yang, R. Zhang, R. Chen, Z. Zhang, W. Zhang, S.-H. Peng, X. Chen, G. Liu, C.-S. Hsu and C.-S. Lee, Adv. Funct. Mater., 2017, 27, 1605094 CrossRef PubMed.
- H. Chen, J. Zhang, K. Chang, X. Men, X. Fang, L. Zhou, D. Li, D. Gao, S. Yin, X. Zhang, Z. Yuan and C. Wu, Biomaterials, 2017, 144, 42–52 CrossRef CAS PubMed.
- K. Chang, D. Gao, Q. Qi, Y. Liu and Z. Yuan, Biomater. Sci., 2019, 7, 1486–1492 RSC.
- Y. Yang, J. Chen, Y. Yang, Z. Xie, L. Song, P. Zhang, C. Liu and J. Liu, Nanoscale, 2019, 11, 7754–7760 RSC.
- B. Guo, Z. Sheng, K. Kenry, D. Hu, X. Lin, S. Xu, C. Liu, H. Zheng and B. Liu, Mater. Horiz., 2017, 4, 1151–1156 RSC.
- B. Guo, Z. Sheng, D. Hu, C. Liu, H. Zheng and B. Liu, Adv. Mater., 2018, 30, 1802591 CrossRef PubMed.
- B. Guo, Z. Sheng, D. Hu, A. Li, S. Xu, P. N. Manghnani, C. Liu, L. Guo, H. Zheng and B. Liu, ACS Nano, 2017, 11, 10124–10134 CrossRef CAS PubMed.
- M. Chen, S. Tang, Z. Guo, X. Wang, S. Mo, X. Huang, G. Liu and N. Zheng, Adv. Mater., 2014, 26, 8210–8216 CrossRef CAS PubMed.
- X. Huang, S. Tang, X. Mu, Y. Dai, G. Chen, Z. Zhou, F. Ruan, Z. Yang and N. Zheng, Nat. Nanotechnol., 2010, 6, 28 CrossRef PubMed.
- K. A. Homan, S. Michael, T. Ryan, G. P. Luke, G. Christopher, V. Erika and E. Stanislav, ACS Nano, 2012, 6, 641–650 CrossRef CAS PubMed.
- Y. Liu, P. Bhattarai, Z. Dai and X. Chen, Chem. Soc. Rev., 2019, 48, 2053–2108 RSC.
- E. Ito, K. W. Yip, D. Katz, S. B. Fonseca, D. W. Hedley, S. Chow, G. W. Xu, T. E. Wood, C. Bastianutto, A. D. Schimmer, S. O. Kelley and F.-F. Liu, Mol. Pharmacol., 2009, 76, 969–983 CrossRef CAS PubMed.
- W. Shang, C. Zeng, Y. Du, H. Hui, X. Liang, C. Chi, K. Wang, Z. Wang and J. Tian, Adv. Mater., 2017, 29, 1604381 CrossRef PubMed.
- S. Duan, Y. Yang, C. Zhang, N. Zhao and F.-J. Xu, Small, 2017, 13, 1603133 CrossRef PubMed.
- Y. Hu, Y. Zhou, N. Zhao, F. Liu and F.-J. Xu, Small, 2016, 12, 2459–2468 CrossRef CAS PubMed.
- S. Barbosa, A. Agrawal, L. Rodríguez-Lorenzo, I. Pastoriza-Santos, R. A. Alvarez-Puebla, A. Kornowski, H. Weller and L. M. Liz-Marzán, Langmuir, 2010, 26, 14943–14950 CrossRef CAS PubMed.
- F. Hao, C. L. Nehl, J. H. Hafner and P. Nordlander, Nano Lett., 2007, 7, 729–732 CrossRef CAS PubMed.
- V. Neuschmelting, S. Harmsen, N. Beziere, H. Lockau, H.-T. Hsu, R. Huang, D. Razansky, V. Ntziachristos and M. F. Kircher, Small, 2018, 14, 1800740 CrossRef PubMed.
- S. Lal, S. E. Clare and N. J. Halas, Acc. Chem. Res., 2008, 41, 1842–1851 CrossRef CAS PubMed.
- Y. Qiao, J. Gumin, C. J. MacLellan, F. Gao, R. Bouchard, F. F. Lang, R. J. Stafford and M. P. Melancon, Nanotechnology, 2018, 29, 165101 CrossRef PubMed.
- Q. Tian, M. Tang, Y. Sun, R. Zou, Z. Chen, M. Zhu, S. Yang, J. Wang, J. Wang and J. Hu, Adv. Mater., 2011, 23, 3542–3547 CrossRef CAS PubMed.
- M. Zhou, R. Zhang, M. Huang, W. Lu, S. Song, M. P. Melancon, M. Tian, D. Liang and C. Li, J. Am. Chem. Soc., 2010, 132, 15351–15358 CrossRef CAS PubMed.
- M. Zhou, J. Li, S. Liang, A. K. Sood, D. Liang and C. Li, ACS Nano, 2015, 9, 7085–7096 CrossRef CAS PubMed.
- W. Yang, W. Guo, W. Le, G. Lv, F. Zhang, L. Shi, X. Wang, J. Wang, S. Wang, J. Chang and B. Zhang, ACS Nano, 2016, 10, 10245–10257 CrossRef CAS PubMed.
- Q. Tian, F. Jiang, R. Zou, Q. Liu, Z. Chen, M. Zhu, S. Yang, J. Wang, J. Wang and J. Hu, ACS Nano, 2011, 5, 9761–9771 CrossRef CAS PubMed.
- K. Ke, W. Yang, X. Xie, R. Liu, L.-L. Wang, W.-W. Lin, G. Huang, C.-H. Lu and H.-H. Yang, Theranostics, 2017, 7, 4763–4776 CrossRef CAS PubMed.
- D. Gao, P. Zhang, C. Liu, C. Chen, G. Gao, Y. Wu, Z. Sheng, L. Song and L. Cai, Nanoscale, 2015, 7, 17631–17636 RSC.
- G. Hong, A. L. Antaris and H. Dai, Nat. Biomed. Eng., 2017, 1, 0010 CrossRef.
- A. M. Smith, M. C. Mancini and S. Nie, Nat. Nanotechnol., 2009, 4, 710 CrossRef CAS PubMed.
- P. Wang, Y. Fan, L. Lu, L. Liu, L. Fan, M. Zhao, Y. Xie, C. Xu and F. Zhang, Nat. Commun., 2018, 9, 2898 CrossRef PubMed.
- C. Li and Q. Wang, ACS Nano, 2018, 12, 9654–9659 CrossRef CAS PubMed.
- Kenry, Y. Duan and B. Liu, Adv. Mater., 2018, 30, 1802394 CrossRef PubMed.
- K. Huang, Y. Zhang, J. Lin and P. Huang, Biomater. Sci., 2019, 7, 472–479 RSC.
- D. Gao, Z. Sheng, Y. Liu, D. Hu, J. Zhang, X. Zhang, H. Zheng and Z. Yuan, Adv. Healthcare Mater., 2017, 6, 1601094 CrossRef PubMed.
- H. Zhang, T. Wang, H. Liu, F. Ren, W. Qiu, Q. Sun, F. Yan, H. Zheng, Z. Li and M. Gao, Nanoscale, 2019, 7600–7608 RSC.
- M. Wu, W. Chen, Y. Chen, H. Zhang, C. Liu, Z. Deng, Z. Sheng, J. Chen, X. Liu, F. Yan and H. Zheng, Adv. Sci., 2018, 5, 1700474 CrossRef PubMed.
- P. Zhou, H. Zhao, Q. Wang, Z. Zhou, J. Wang, G. Deng, X. Wang, Q. Liu, H. Yang and S. Yang, Adv. Healthcare Mater., 2018, 7, 1701201 CrossRef.
- Z. Peng, X. Han, S. Li, A. O. Al-Youbi, A. S. Bashammakh, M. S. El-Shahawi and R. M. Leblanc, Coord. Chem. Rev., 2017, 343, 256–277 CrossRef CAS.
- Y. Zhou, Z. Peng, E. S. Seven and R. M. Leblanc, J. Controlled Release, 2018, 270, 290–303 CrossRef CAS PubMed.
- M. Zheng, S. Ruan, S. Liu, T. Sun, D. Qu, H. Zhao, Z. Xie, H. Gao, X. Jing and Z. Sun, ACS Nano, 2015, 9, 11455–11461 CrossRef CAS PubMed.
- S. Wang, C. Li, M. Qian, H. Jiang, W. Shi, J. Chen, U. Lächelt, E. Wagner, W. Lu, Y. Wang and R. Huang, Biomaterials, 2017, 141, 29–39 CrossRef CAS PubMed.
- A. d. l. Zerda, Z. Liu, S. Bodapati, R. Teed, S. Vaithilingam, B. T. Khuri-Yakub, X. Chen, H. Dai and S. S. Gambhir, Nano Lett., 2010, 10, 2168–2172 CrossRef PubMed.
- A. De la Zerda, C. Zavaleta, S. Keren, S. Vaithilingam, S. Bodapati, Z. Liu, J. Levi, B. R. Smith, T.-J. Ma, O. Oralkan, Z. Cheng, X. Chen, H. Dai, B. T. Khuri-Yakub and S. S. Gambhir, Nat. Nanotechnol., 2008, 3, 557–562 CrossRef CAS.
- M. Qian, Y. Du, S. Wang, C. Li, H. Jiang, W. Shi, J. Chen, Y. Wang, E. Wagner and R. Huang, ACS Appl. Mater. Interfaces, 2018, 10, 4031–4040 CrossRef CAS PubMed.
- J. Chen, C. Liu, D. Hu, F. Wang, H. Wu, X. Gong, X. Liu, L. Song, Z. Sheng and H. Zheng, Adv. Funct. Mater., 2016, 26, 8715–8725 CrossRef CAS.
- A. Hariri, J. Lemaster, J. Wang, A. S. Jeevarathinam, D. L. Chao and J. V. Jokerst, Photoacoustics, 2018, 9, 10–20 CrossRef PubMed.
Footnote |
† These authors contributed equally to this work. |
|
This journal is © The Royal Society of Chemistry 2019 |
Click here to see how this site uses Cookies. View our privacy policy here.