DOI:
10.1039/C9NH00168A
(Communication)
Nanoscale Horiz., 2019,
4, 1153-1157
Nanoporous gold metamaterials for high sensitivity plasmonic sensing†
Received
14th March 2019
, Accepted 3rd May 2019
First published on 3rd May 2019
Abstract
Surface plasmon resonance sensors are a well-established class of sensors that includes a very large variety of materials and detection schemes. However, the development of portable devices is still challenging due to the intrinsic complexity of the optical excitation/detection schemes. This work shows that nanoporous gold (NPG) films can overcome the said limitations by providing an excellent sensitivity without the need for sophisticated fabrication approaches and/or optical setups. The sensing mechanism is related to the co-localization of optical energy and analytes in the pores fostering an enhanced light–matter coupling. As a result, when molecules are adsorbed in the pores, the NPG film shows a significant spectral shift of the effective plasma frequency and an abrupt change of the reflectivity. By monitoring the reflectivity in the spectral region close to the plasma frequency (namely the plasma edge), it is possible to detect the analyte. Through a series of experiments, the authors demonstrated a sensitivity exceeding 15
000 nm per RIU in the near infrared range comparable with the state of the art of plasmonic metamaterials.
New concepts
Most of the optical approaches applied in biosensing are based on sophisticated excitation/detection schemes that hinder the development of cheap and portable sensors. Here, we show that a simple nanoporous gold film can be exploited as a plasmonic metamaterial sensor exhibiting a sensitivity comparable to the state of the art but with no need of complex nanofabrication strategies and/or optical setups. The interaction of the analyte and a metallic porous matrix modulates the effective plasma frequency of the material, a parameter that can be simply measured in the near infrared range. Hence, a given analyte can be detected by monitoring the reflectivity of the nanoporous material. The key point enabling a sensitivity up to 15 000 nm per RIU is that the subwavelength porous matrix provides a very effective accumulation of the optical energy into the pores (plasmonic hot spots) which can be used to capture the analyte. In this way, an efficient co-localization of the molecules and optical energy can be achieved promoting a stronger light–matter interaction and a more effective energy transfer. Our work can enable a new pathway towards the development of a class of sensors with extreme sensitivity that can be prepared without any lithographic procedure.
|
Introduction
Biosensing technologies are emerging for quick real-time identification of biomarkers and to quantify several species of interest at the same time, i.e. multiplex detection.1–3 Most of these requirements can be satisfied by leveraging the optical confinement and enhancement produced by plasmonic resonances. Surface plasmon resonances (SPR) are extremely sensitive to the dielectric permittivity of the environment in contact with the metal surface.4–6 Therefore, when molecules are adsorbed in plasmonic nanostructures, the spectral positions of their resonances undergo significant shifts even for few molecule binding events. The sensitivity of these classes of sensors is therefore usually expressed as S = Δλ/Δn, i.e. the ratio between the spectral shift Δλ of the plasmon resonance and the change of the refractive index Δn surrounding the sensor, both induced by the presence of the analyte. Over the years, a wide range of optical excitation and detection schemes have been proposed together with the use of several kinds of nanostructures.4,7,8 In particular, two main classes can be identified: (i) those based on propagating plasmons, usually excited via grating coupling, allowing the implementation of a microarray format for a multiplexed and high-throughput biosensing platform;9,10 (ii) those based on localized resonances in individual nanostructures of different sizes and shapes, either dispersed in colloidal solution or fabricated on substrates through lithographic methods.11–14 Such a long-standing challenge recently culminated in plasmonic metamaterial based sensors showing a sensitivity approaching 30
000 nm per RIU,8,15–17 in the near infrared region. However, high sensitivity is very often associated with expensive fabrication methods or sophisticated detection schemes. In this respect, nanoporous gold (NPG) offers a valid alternative providing low-cost fabrication methods and simple excitation schemes of strong plasmonic resonances.18,19 Among the several newly investigated plasmonic materials,20 NPG presents significant advantages mainly due to the large surface/volume ratio.21 The latter ensures more binding sites not achievable with bulk metals.22 However, in previous work, the authors also demonstrated that NPG behaves like a metamaterial whose effective dielectric response can be tailored by changing the pore size and porosity, or equivalently by changing the fractal dimension.23 In particular, the plasma frequency can be shifted over a wide spectral range of infrared wavelengths. Indeed, the porous metamaterial exhibits greater plasmonic properties compared to its bulk counterpart. These properties, combined with a higher skin depth on the order of 100–200 nm, enable the penetration of light deep into the nanopores where the analyte can be accumulated. Such an efficient co-localization of analytes and optical energy promotes a robust light–matter coupling and a high detection sensitivity.24
This work shows that the spectral position of the effective plasma frequency (or the plasma edge) of the nanoporous plasmonic metamaterial is extremely sensitive to the index of refraction of the surrounding environment. Therefore, it can be used as a high-performance SPR sensor. Through a series of experiments, the authors showed a sensitivity exceeding 15
000 nm per RIU at λ = 1550 nm. This result can be achieved with a very easy excitation/detection scheme that can consist of a reflectance measurement at a fixed angle and wavelength. It does not require complex optical setups and/or advanced nanostructuring, thus opening the route to portable SPR nanosensors with extreme sensitivity and at low cost.
Results and discussion
During the last decade, nanostructured porous gold films have been prepared following different methods.21,25,26 Here, the preparation of NPG films followed a simple well-established procedure (already reported in detail23,24) in which a 3-hour optimized dealloying process was carried out. Noteworthily, as recently reported,23 tuning the dealloying time makes it possible to modify the morphology of the obtained NPG film, and consequently the plasma frequency. Here, the fabrication parameters were adjusted in order to obtain the plasma frequency in the NIR band (around 1550 nm – typical wavelength of low-cost IR sources commercially available). The concept of plasma frequency or plasma edge sensing is illustrated in Fig. 1, together with a representative SEM image of the fabricated samples. The plasma edge is the spectral band, near the plasma frequency, in which the material switches from reflective to transparent.23 As a result of the enhanced light–matter coupling occurring in the nanopores, the plasma edge of the NPG is extremely sensitive to the index of refraction of the material filling the nanopores. By measuring the reflectivity, it is then possible to measure the plasma edge shifts and monitor the amount of analyte present in the nanopores.
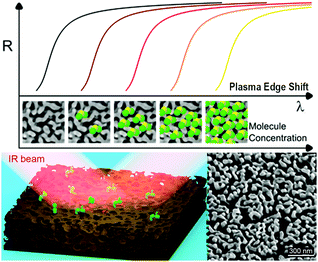 |
| Fig. 1 Sensing approach – a near infrared light beam illuminates the nanoporous gold, and the reflectance is measured around the plasma edge region where a significant spectral shift can be detected as a function of the number of molecules deposited on top of the material surface. | |
This first work focuses on the correct estimation of the sensitivity. Indeed, the latter is not trivial since the very large surface area provided by the nanoporous materials can lead to a misleading interpretation. This problem was approached in three different ways, giving comparable results.
First of all, the plasma edge shifts of NPG were monitored after successive depositions of very thin layers of SiO2 by atomic layer deposition (ALD) allowing a conformal coating of uniform thickness all over the NPG surface. This allows exact control of the amount of material deposited, which is why this method was chosen.27,28 After each deposition of 1.5 nm in thickness of SiO2 (final thickness 9 nm), the reflectance was measured via Fourier Transform InfraRed (FTIR) spectroscopy in the spectral range spanning from 1400 to 2800 nm.
The results are reported in Fig. 2: while the thickness of the SiO2 layer increases, the reflectivity significantly drops and the plasma edge redshifts even for vanishingly small quantities of SiO2. The spectral shifts extracted from Fig. 2a are reported in Fig. 2b. For comparison, the shifts achievable with a conventional approach were computed, such as the grating coupled SPR29 (GC-SPR) working in the same spectral range (see also ESI† – Supporting note #1). As evident, the NPG exhibits spectral shifts 100 times larger than those of a GC-SPR under the same conditions. In agreement with well-established literature, the conducted simulations estimated a GC-SPR sensitivity of about 1500 nm per RIU.29 This suggests an empiric sensitivity of 1500 × 100 = 150
000 nm per RIU for the NPG sensor. However, this value must be corrected by a factor due to the higher surface area of NPG with respect to the bulk grating. Although an accurate measurement of this parameter is not simple, a factor between 5 and 10 has been reported in several papers.30 Therefore, a conservative estimation of the sensitivity of the NPG sensor is in the order of 15
000 nm per RIU, which is within the same order of magnitude as the state of the art metamaterials.8,15–17,31
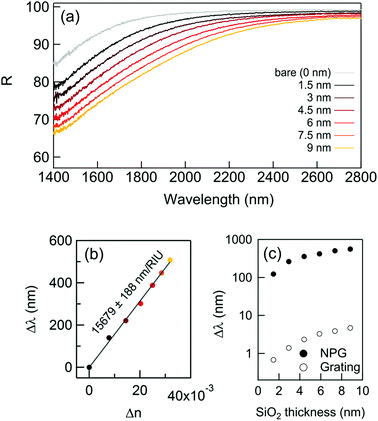 |
| Fig. 2 The NPG sensing performance with a thin dielectric coating deposition. (a) The reflectance curves after successive SiO2 layer depositions; (b) spectral shift measured at R(0.85%); (c) calculated sensitivity using the formula (1): comparison with the spectral shift obtained with the same dielectric coatings on a standard bulk gold grating.22 | |
The second approach for evaluating the sensitivity consisted of treating the NPG-SiO2 material as an insulator–metal multilayered metamaterial, whose overall effective dielectric function was dominated by the component longitudinal to the film plane, which can be easily calculated applying the interface conditions for the electric field vector (see Methods for details).32,33 By using this approximation, it was possible to calculate the refractive index shift Δn(ω,t) induced by the depositions of SiO2. Given Δn(ω,t), the sensitivity expressed in nm per RIU follows from the well-known figure of merit:
| S(ω,t) = (λt(R) − λ0(R))/(Δn(ω,t)) | (1) |
where
λt(
R) −
λ0(
R) is the spectral shift of the reflectivity at a fixed value
R as a function of the thickness variation Δ
t of the deposited SiO
2. The results reported in
Fig. 2b show an average sensitivity of 15
![[thin space (1/6-em)]](https://www.rsc.org/images/entities/char_2009.gif)
700 nm per RIU in good agreement with the empiric evaluation achieved by comparing the NPG with a conventional grating.
The third assessment of the sensitivity was performed by measuring the spectral shifts of the NPG plasma edge in the liquid phase using a solution of glycerol in water.
This procedure has already been reported in several papers17,31 and it can be considered a standard method. Since water presents significant absorbance lines in the NIR band, a dedicated microfluidic chamber34 (see ESI† – Supporting note #2) was exploited, enabling reproducible measurements to be achieved. The NPG film was integrated into a 10 microns thick chamber with an IR transparent CaF2 top window. Before each experiment, the NPG was treated in O2 plasma (100 W, 100% O2 for 60 seconds) in order to guarantee the wettability of the porous matrix. Another critical aspect observed during these “in-liquid” measurements was the washing step after every data collection for a particular concentration of glycerol. It was observed that the organic molecules tend to deposit on the NPG surface after several minutes. This created a non-uniform layer of “dirty” metal with a non-reproducible optical response (see ESI† – Supporting note #3 for an example). The microfluidic system developed for this experiment was optimized in order to be extendable, i.e. it can be opened and closed to access the substrate for the cleaning or washing procedure. This allowed washing of the NPG film in ethanol after each measurement. The washing step was verified by checking the NPG surface in SEM and by measuring the optical response in H2O at the end of the experiment.
Fig. 3 illustrates the shift of the plasma edge obtained for glycerol concentrations between 1 and 10% m V−1, which are typically used in the literature.17 The corresponding variations of the index of refraction Δn are equal to 0.00113; 0.00227; 0.005764; and 0.01179, respectively.35 These measurements allowed the sensitivity to be evaluated directly by measuring the spectral shift of the reflectivity Δλ(R) for different values of the index of refraction of the environment (water + glycerol). Fig. 3b and c report the measured shifts and the obtained sensitivity (calculated considering the shift at R = 45% − Speak − and average shift − Savg − respectively) that resulted in good agreement with the evaluations discussed above.
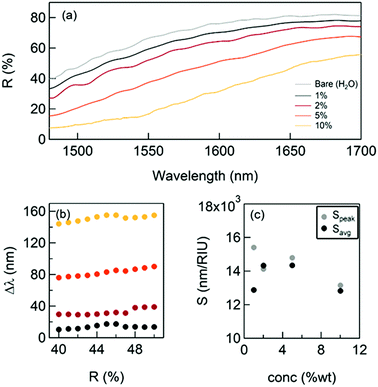 |
| Fig. 3 The NPG sensing performance in H2O + glycerol. (a) The reflectance curves with different concentrations of glycerol; (b) spectral shift measured at different R (between 40 and 50%); (c) obtained sensitivity in terms of Δλ/Δn (nm per RIU). Speak indicates the values obtained considering the shift at R = 45%, while Savg has been obtained considering the average spectral shift. | |
As a final test, the performance of the proposed sensor was validated by detecting low molecular weight proteins in solution. As a proof of concept, the detection approach was tested using a small molecule (ca. 1 kDa). The samples containing a different concentration of polyhystideine – 7HIS were analyzed after the functionalization of the sensor surface. (Fig. 4 – top panel). The method can be successfully used for the detection of bigger molecules such as proteins. The covalent linkage of the analyte to the surface allows a better and more trustable detection, which can be accomplished both in a wet and dry situation.
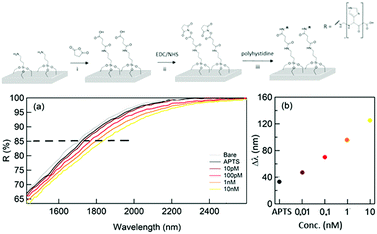 |
| Fig. 4 The sensing performance of a bioanalyte. Top panel: functionalization protocol to link 7HIS with different concentrations on the NPG surface; (a) measured reflectance curves; (b) spectral shifts. | |
The results of the reflectance spectroscopy performed by the means of FTIR for concentrations of 7HIS between 10 pM and 10 nM are illustrated in Fig. 4(a) and (b). Indeed, by using this approach, a detection limit of 10 pM was achieved, in agreement with what was obtained by using state of the art metamaterial sensors.36
The first important fact to underline is the ability of the NPG platform to detect the first functional layer of (3-aminopropyl)triethoxysilane (APTS) deposited by means of ALD as a monolayer. In order to evaluate this first detection limit, the thickness of the organic monolayer has been measured by means of XPS (see ESI† – Supporting note #4) and was found to be about 0.6 nm.
Moreover, the illustrated results demonstrate how the NPG platform is able to detect low concentrations of a polypeptide (down to the equivalent of 1.4 nm in thickness) correlating them into significant spectral shifts and reflectance variations. In total, we expect an analyte layer 2 nm thick with a coverage that depends on the 7HIS concentration and the linkage efficiency. In the frequency region of the reflectivity drop, the refractive indexes of 7HIS and SiO2 are 1.70 and 1.47, respectively and lead to a maximum shift comparable to that obtained with the first SiO2 deposition.
The reported sensitivity can be explained by considering the plasmonic response of the NPG in the NIR range. As illustrated in the authors’ experiments, the field enhancement and confinement provided by the NPG at the metal/air or metal/liquid interface were much higher than that provided by the bulk counterpart. The porous structure enabled a spontaneous co-localization of the plasmonic field and the analyte, thus promoting effective interaction between them.
Indeed, similar results can be achieved without having to measure the spectral response. In fact, after a proper sensor calibration, a direct measurement of the reflectivity at a fixed wavelength and a fixed angle can give an estimation of the analyte concentration. In perspective, such a scheme would lead to the development of cheap and portable SPR sensors.
Conclusions
This study demonstrated that nanoporous gold films show an effective plasma frequency or plasma edge whose spectral position is extremely sensitive to the index of refraction of the material filling the nanopores. Therefore, NPG films can be used as very sensitive SPR sensors. Both the optical excitation and detection schemes are very easy and do not require sophisticated fabrications or experimental setups. A sensitivity exceeding 15
000 nm per RIU was demonstrated by using different approaches. In light of these features, the authors believe that these classes of metamaterials can lead to a novel generation of portable SPR sensors with extreme sensitivity.
Methods
Optical spectroscopy
The optical response in the IR range was investigated by means of FT-IR reflectance microscopy measurements by using a Nicolet™ iS™ 50 FT-IR Spectrometer coupled with a Nicolet Continuμm Infrared Microscope by Thermo Scientific.™ A gold mirror was used as a reference. The optical response of this effective porous conductor was investigated using a Drude–Lorentz model. Within this context, the dielectric permittivity was characterized by a reduction of the absolute values with respect to the bulk counterpart, mitigating the optical losses and increasing the skin depth, thus enabling the penetration of the electromagnetic field deeply into the porous matrix loaded with the analyte to be detected.
In order to assess the benefits of NPG in an SPR configuration, the far-field optical response of the fabricated film was measured by means of FTIR spectroscopy using a microscope with a 30°-incidence Cassegrain objective in the reflection mode.
Refractive index calculation
In the case of SiO2 deposition, the refractive index variation Δn was empirically calculated under the following assumption: the film covered by the SiO2 can be thought of as an insulator–metal multilayered metamaterial, whose overall effective dielectric function was dominated by the component longitudinal to the film plane. This can be easily calculated by applying the interface conditions for the electric field vector. The dielectric functions used for these estimations are found in the literature for gold and silicon dioxide, while for the NPG the previously calculated dielectric function was used. Finally, recalling that
, the following relation can be established:
where εnpg,SiO2 and tnpg,SiO2 are the dielectric functions and the thicknesses of the NPG and the silicon dioxide, respectively. Under the above assumption, the refractive index shifts are reported in ESI† – Fig. S5 as a function of the wavelength for a 200 nm thick NPG film.
Surface functionalization protocol for dry sensing experiments
The NPG surface was firstly covered by APTES by ALD – self-limited deposition of a single layer (see ESI† – Note #4). The surface was then incubated for two hours at 40 °C with 5 mM (5 mL) solution of succinic anhydride in water. The surface was washed with water (5 mL) to remove the unreacted anhydride, and the carboxyl groups thus exposed at the surface were activated with 5 mM EDC 5 mM NHS solution for 30 minutes at room temperature (R.T.). The coupling reagents were removed by flushing the surface with water. The surface was then treated with 50 and 10 nM solution of polyhystidine at R.T. After 2 hours, the surface was washed once and analysed in dry conditions.
The amino groups available on the surface were modified by succinic anhydride in order to achieve carboxyl groups at the surface. After EDC/NHS activation, the carboxyl groups available on the surface were coupled with the primary amine of the desired analyte.
Conflicts of interest
There are no conflicts to declare.
Acknowledgements
The research leading to these results has received funding from the Horizon 2020 Program, FET-Open: PROSEQO, Grant Agreement no. 687089.
Notes and references
-
F.-G. Bănică, Chemical Sensors and Biosensors, John Wiley & Sons, Ltd, Chichester, UK, 2012 Search PubMed.
-
Label-Free Biosensors, ed. M. A. Cooper, Cambridge University Press, Cambridge, 2009 Search PubMed.
- G. Zanchetta, R. Lanfranco, F. Giavazzi, T. Bellini and M. Buscaglia, Nanophotonics, 2017, 6(4), 627–645 CAS.
- J. N. Anker, W. P. Hall, O. Lyandres, N. C. Shah, J. Zhao and R. P. Van Duyne, Nat. Mater., 2008, 7, 442–453 CrossRef CAS PubMed.
- G. Homola, J. Yee and S. S. Gauglitz, Sens. Actuators, B, 1999, 54, 3–15 CrossRef.
- B. Liedberg, C. Nylander and I. Lunström, Sens. Actuators, 1983, 4, 299–304 CrossRef CAS.
- J. R. Mejía-Salazar and O. N. Oliveira, Chem. Rev., 2018, 118, 10617–10625 CrossRef PubMed.
- Y. Lee, S.-J. Kim, H. Park and B. Lee, Sensors, 2017, 17, 1726 CrossRef PubMed.
- H. H. Nguyen, J. Park, S. Kang and M. Kim, Sensors, 2015, 15, 10481–10510 CrossRef CAS PubMed.
- F. Yesilkoy, R. A. Terborg, J. Pello, A. A. Belushkin, Y. Jahani, V. Pruneri and H. Altug, Light: Sci. Appl., 2018, 7, 17152 CrossRef CAS PubMed.
- Y. Shen, J. Zhou, T. Liu, Y. Tao, R. Jiang, M. Liu, G. Xiao, J. Zhu, Z. K. Zhou, X. Wang, C. Jin and J. Wang, Nat. Commun., 2013, 4, 1–9 Search PubMed.
- M.-C. Estevez, M. A. Otte, B. Sepulveda and L. M. Lechuga, Anal. Chim. Acta, 2014, 806, 55–73 CrossRef CAS PubMed.
- J.-E. Lee, K. Chung, J. Lee, K. Shin and D. H. Kim, Adv. Funct. Mater., 2015, 25, 6716–6724 CrossRef CAS.
- S. Gao, N. Koshizaki, H. Tokuhisa, E. Koyama, T. Sasaki, J.-K. Kim, J. Ryu, D.-S. Kim and Y. Shimizu, Adv. Funct. Mater., 2010, 20, 78–86 CrossRef CAS.
- K. V. Sreekanth, Y. Alapan, M. ElKabbash, A. M. Wen, E. Ilker, M. Hinczewski, U. A. Gurkan, N. F. Steinmetz and G. Strangi, Adv. Opt. Mater., 2016, 4, 1767–1772 CrossRef CAS PubMed.
- A. V. Kabashin, P. Evans, S. Pastkovsky, W. Hendren, G. A. Wurtz, R. Atkinson, R. Pollard, V. A. Podolskiy and A. V. Zayats, Nat. Mater., 2009, 8, 867 CrossRef CAS PubMed.
- K. V. Sreekanth, Y. Alapan, M. Elkabbash, E. Ilker, M. Hinczewski, U. A. Gurkan, A. De Luca and G. Strangi, Nat. Mater., 2016, 15, 621–627 CrossRef CAS PubMed.
- J. Biener, G. W. Nyce, A. M. Hodge, M. M. Biener, A. V. Hamza and S. A. Maier, Adv. Mater., 2008, 20, 1211–1217 CrossRef CAS.
- A. Wittstock, J. Biener and M. Bäumer, Phys. Chem. Chem. Phys., 2010, 12, 12919 RSC.
- G. V. Naik, V. M. Shalaev and A. Boltasseva, Adv. Mater., 2013, 25, 3264–3294 CrossRef CAS PubMed.
- X. Zhang, Y. Zheng, X. Liu, W. Lu, J. Dai, D. Y. Lei and D. R. MacFarlane, Adv. Mater., 2015, 27, 1090–1096 CrossRef CAS PubMed.
- E. Detsi, E. De Jong, A. Zinchenko, Z. Vuković, I. Vuković, S. Punzhin, K. Loos, G. Ten Brinke, H. A. De Raedt, P. R. Onck and J. T. M. De Hosson, Acta Mater., 2011, 59, 7488–7497 CrossRef CAS.
- D. Garoli, E. Calandrini, A. Bozzola, A. Toma, S. Cattarin, M. Ortolani and F. De Angelis, ACS Photonics, 2018, 5, 3408–3414 CrossRef.
- D. Garoli, E. Calandrini, A. Bozzola, M. Ortolani, S. Cattarin, S. Barison, A. Toma and F. De Angelis, Nanoscale, 2017, 9, 915–922 RSC.
- L. Zhang, X. Lang, A. Hirata and M. Chen, ACS Nano, 2011, 5, 4407–4413 CrossRef CAS PubMed.
- C. Zhu, Z. Qi, V. A. Beck, M. Luneau, J. Lattimer, W. Chen, M. A. Worsley, J. Ye, E. B. Duoss, C. M. Spadaccini, C. M. Friend and J. Biener, Sci. Adv., 2018, 4, eaas9459 CrossRef PubMed.
- L. Qian, W. Shen, B. Shen, G. W. Qin and B. Das, Nanotechnology, 2010, 21(30), 305705 CrossRef PubMed.
- L. Qian, B. Shen, G. W. Qin and B. Das, J. Chem. Phys., 2011, 134, 014707 CrossRef PubMed.
- J. Homola, I. Koudela and S. S. Yee, Sens. Actuators, B, 1999, 54, 16–24 CrossRef CAS.
- E. Rouya, S. Cattarin, M. L. Reed, R. G. Kelly and G. Zangari, J. Electrochem. Soc., 2012, 159(4), K97–K102 CrossRef CAS.
- A. I. Aristov, M. Manousidaki, A. Danilov, K. Terzaki, C. Fotakis, M. Farsari and A. V. Kabashin, Sci. Rep., 2016, 1–8 Search PubMed.
- X. Chen, T. M. Grzegorczyk, B.-I. Wu, J. Pacheco and J. A. Kong, Phys. Rev. E: Stat., Nonlinear, Soft Matter Phys., 2004, 70, 016608 CrossRef PubMed.
-
F.-G. Hu, J. Song and T. Kamgaing, in 19th Topical Meeting on Electrical Performance of Electronic Packaging and Systems, IEEE, 2010, pp. 225–228.
- O. Limaj, D. Etezadi, N. J. Wittenberg, D. Rodrigo, D. Yoo, S.-H. Oh and H. Altug, Nano Lett., 2016, 16, 1502–1508 CrossRef CAS PubMed.
- L. F. Hoyt, Ind. Eng. Chem., 1934, 26, 329–332 CrossRef CAS.
- G. Hong, C. Li and L. Qi, Adv. Funct. Mater., 2010, 20, 3774–3783 CrossRef CAS.
Footnote |
† Electronic supplementary information (ESI) available. See DOI: 10.1039/c9nh00168a |
|
This journal is © The Royal Society of Chemistry 2019 |
Click here to see how this site uses Cookies. View our privacy policy here.