DOI:
10.1039/C9NA00168A
(Paper)
Nanoscale Adv., 2019,
1, 2580-2585
Cupredoxin engineered upconversion nanoparticles for ratiometric luminescence sensing of Cu2+†
Received
19th March 2019
, Accepted 13th May 2019
First published on 13th May 2019
Abstract
The NIR excitation and large anti-Stokes shift of upconversion nanoparticles (UCNPs) have made them an ideal choice as biological nanoprobes. A key challenge in the field is to confer biorecognition units to UCNPs so that they can be used to probe specific targets in biological systems. While various agents have been combined with UCNPs to meet such a challenge, most studies are limited to small molecules, while biomolecules such as metalloproteins that possess much higher affinity and selectivity for metal ions have not been explored. Herein we demonstrate that fusion of zwitterion-coated UCNPs with azurin, a member of a family of redox-active copper proteins called cupredoxins that play important roles in diverse biological functions, can serve as an ideal platform for the label-free upconversion luminescence sensing of Cu2+ with a ratiometric response. The selective binding of apo-azurin with Cu2+ induces a significant absorbance at about 625 nm, and hence decreases the red emission of the UCNPs. In contrast, the green emission of the UCNPs remains constant and acts as an internal standard reference for the ratiometric sensing of Cu2+. This approach opens a new window for the development of assays for biosensing based on a combination of specific metalloproteins with UCNPs.
Introduction
Lanthanide ion doped upconversion nanoparticles (UCNPs) are a new class of luminescent probes that convert NIR excitation light into shorter wavelength luminescence via multiphoton absorption and energy transfer processes, which can achieve higher-contrast optical sensing and imaging as well as deeper tissue penetration due to a suppression of autofluorescence from biomolecules and a light scattering background.1–4 In contrast to organic fluorophores and quantum dots (QDs), UCNPs exhibit neither photoblinking nor photobleaching, and their rare earth components are much less toxic than the heavy metals within QDs.1a,b Due to these advantages, UCNPs have attracted attention from researchers in fields such as sensing,2d–g,5–7 imaging,1b,3a–d and therapeutics.3a,4b Within this context, a number of UCNP-based sensor systems have been reported to detect various targets, such as metal ions,5 anions,6 and biomolecules.7 The key for the construction of such sensors is the integration of UCNPs with suitable molecules that possess specific recognition sites. However, the molecules used are mainly limited to small organic molecules. Since nature has evolved a number of tight regulatory proteins with high selectivity and sensitivity for binding of targets such as metal ions, the usage of these proteins as a sensor tool is currently attracting much interest.8 A major limitation of this design is the transformation of the binding events into an easily detectable signal. In this report, UCNPs were coupled with a unique redox protein (azurin) as a ratiometric sensor for Cu2+ sensing (Fig. 1).
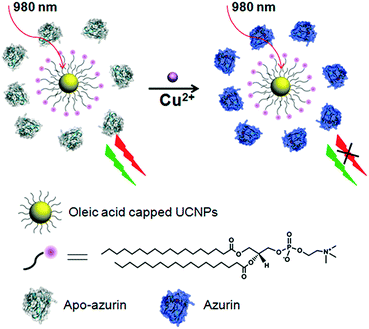 |
| Fig. 1 Schematic illustration of the ratiometric upconversion luminescence sensing of Cu2+ based on an azurin–UCNP biosensor. | |
As a redox-active metal ion, copper is a cofactor involved in many important biological processes, such as oxidative scavenging and respiration.9 However, at elevated concentrations, it can cause a number of severe health problems such as Menkes and Wilson's diseases, Alzheimer's disease, and liver or kidney damage.9c Therefore, the design of fluorescent copper sensors has become a hot topic in analytical chemistry and many effective metal ion sensors based on small organic molecules, proteins, and DNA have been reported.8,10 Despite the progress, facile on-site and real-time detection and quantification of Cu2+ in aqueous solution remain complicated due to its paramagnetic quenching effects on fluorophores.11 Alternatively, ratiometric sensors with a change in the ratio of multiple emission bands provide built-in correction for environmental effects, and thus are free of such quenching problems.
Results and discussion
Typical NaYF4:20%Yb,2%Er UCNPs were synthesized using oleic acid as a capping ligand.2a Upon NIR excitation (980 nm), the UCNPs exhibit multiple upconversion luminescence (UCL) bands in the green and red regions, respectively (Fig. 2). Azurin is a well-characterized, 14 kDa electron-transfer protein involved in bacterial denitrification, such as those in Pseudomonas aeruginosa.12 It has unique spectroscopic features including strong absorption (>5000 M−1 cm−1) in the UV-vis absorption spectrum and a small hyperfine splitting (∼50 × 10−4 cm−1) for EPR.12 In the form without Cu2+ binding, apo-azurin shows no absorption in the visible region. Apo-azurin could bind Cu2+ quite tightly through two histidines (N), a cysteine (S), and a methionine (S) (Fig. 3) and yield a significant absorbance at 625 nm with a molar extinction coefficient of 5600 M−1 cm−1,12 which ideally overlaps with the red emission band of the UCNPs. In this way, the red emission band at 654 nm of UCNPs is absorbed to various degrees, while its emission band at 521 nm, by contrast, is constant and serves as an internal reference for the ratiometric sensing of Cu2+.
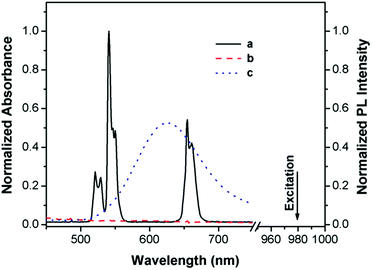 |
| Fig. 2 UCL spectrum of DSPC-coated NaYF4:20%Yb,2%Er (DSPC–UCNPs) under excitation from a 980 nm laser (a), and UV-vis spectra of the protein apo-azurin in the absence (b) and presence of Cu2+ (c). | |
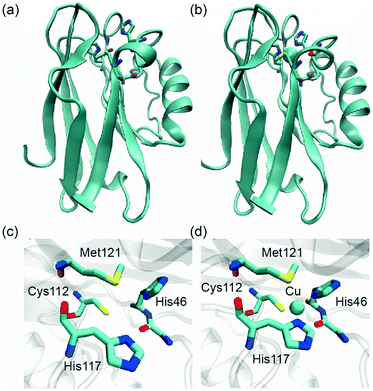 |
| Fig. 3 (a and b) Three-dimensional illustration showing the structure of (a) apo-azurin with a β barrel structure without Cu2+ and (b) azurin with Cu2+; the Cu2+-binding domain (c) without and (d) with Cu2+ coordination. | |
The NaYF4:20%Yb,2%Er nanocrystals display a uniform hexagonal plate-like morphology with mean sizes of ∼60 nm (Fig. 4a and b and S1†). As they are prepared in organic solvents and capped with hydrophobic ligands, they are less water-dispersible and lack any functional groups for surface modification, which is a problem for biomedical application of this class of materials.4i,4j To increase their water-dispersibility and biocompatibility, a phospholipid with a zwitterionic headgroup, distearoylphosphatidylcholine (DSPC), was used to coat UCNPs with an outer surface mimicking the functionality of the cell's external membrane, since phosphorylcholine (PC) is the major hydrophilic part of the cell outer membrane. In an aqueous medium, the fatty acid chains of the phospholipid DSPC were embedded in the hydrophobic surface of the UCNPs, and the hydrophilic phosphorylcholine groups point out toward the aqueous environment, thus forming a coating with a structure analogous to the cell membrane.4i Representative TEM images of the resulting DSPC–UCNPs show that they remain monodisperse in water without aggregation (Fig. 4c and S1†). High-resolution TEM investigation confirms the single crystallized nanocrystals with a ∼4 nm thick uniform amorphous oleic acid/lipid layer around it (Fig. 4d). The DSPC–UCNPs could be well dispersed in water due to the phospholipids on the outer surface. Upon continuous excitation at 980 nm, the total luminescence of the DSPC–UCNPs in water appears yellow-green in color because of the combination of green and red emissions (Fig. 4e). The corresponding UCL spectrum of the DSPC–UCNPs in water is similar to that of the as-prepared UCNPs in cyclohexane, shown in Fig. 4f. Excited with 980 nm, the Yb3+ ions transfer energy to Er3+ and result in the characteristic Er3+ green emission bands between 514 and 534 nm and between 534 and 560 nm due to the transitions from 2H11/2 and 4S3/2 to 4I15/2, respectively, and a red emission band between 635 and 680 nm due to the transition from 4F9/2 to 4I15/2.1a Furthermore, DSPC–UCNPs showed prominently long-term (months) stability in water and excellent resistance to aggregation due to a balanced charge of the zwitterionic surface (Fig. S2†). Considering the cell membrane mimetic surface, high water solubility and stability, and unique optical properties of DSPC–UCNPs, it will offer an ideal platform for biosensing and bioimaging.
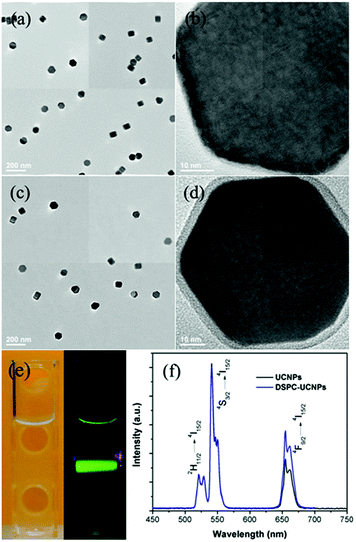 |
| Fig. 4 TEM images of the (a and b) as-prepared and (c and d) phospholipid DSPC coated UCNPs deposited on a TEM grid from a drop of UCNP cyclohexane solution and DSPC–UCNP water solution, respectively; (e) photographs of the water solution of DSPC–UCNPs without laser illumination and the upconverted visible luminescence under continuous-wave 980 nm laser illumination; (f) room-temperature UCL spectra of the as-prepared UCNPs in cyclohexane and DSPC–UCNPs in water under excitation at 980 nm. | |
Upon addition of Cu2+ to a solution of apo-azurin and DSPC–UCNP system, a color change from colorless to blue was observed. The intensity of the red UCL emission at 654 nm decreased gradually with increasing Cu2+ concentration, whereas the intensity of the green UCL band at 521 nm remained constant (Fig. 5a). Introduction of Cu2+ induced an increase in the spectral overlap between the red UCL emission of the UCNPs and the absorption spectrum of the metalloprotein, corresponding to decreased red UCL through the internal filter effect (IFE).12b Since the cell membrane mimetic coating of UCNPs could prevent the adsorption of biomolecules in a biological environment, non-specific interactions were avoided between the protein and DSPC–UCNPs, making IFE play a major role. For a control experiment, the response of DSPC–UCNPs without apo-azurin on Cu2+ was investigated, where the Cu2+-dependent UCL emission change was not observed, which further confirms the importance of the protein to the sensor design (Fig. S3†). Since the green UCL emission at 520 nm is independent of Cu2+, this UCL emission could serve as an internal standard reference and the ratio I521/I654 was used as the detection signal. As shown in Fig. 5b, the intensity ratios of the two UCL emissions (I521/I654) gradually increase with the increasing amount of Cu2+. The signal ratios versus Cu2+ concentrations can be fitted to a linear regression equation with a detection limit of 2 μM, which is comparable to or lower than that of the previously reported methods.10d–f In addition, the detection limit is well below the US Environmental Protection Agency and World Health Organization defined limits of 20 and 30 μM. Therefore, the detection system provides a novel way of copper detection in drinking water.
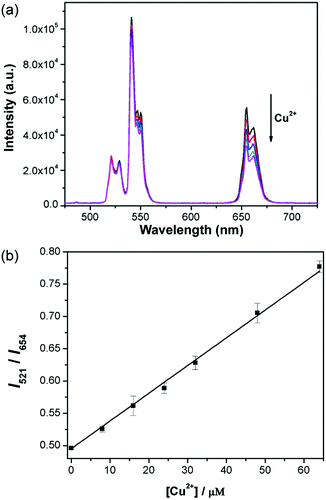 |
| Fig. 5 (a) UCL spectra of the apo-azurin–UCNP system (under excitation from a 980 nm laser) in the presence of varying concentrations of Cu2+ ions; (b) the ratio (I521/I654) of UCL intensities of the sensor system at 521 and 654 nm as a function of Cu2+ concentration. | |
Furthermore, the time-dependent response of the system to Cu2+ was investigated. A response time of ∼40 seconds was observed upon addition of Cu2+ (Fig. S4†), which suggests that the hybrid system with a quick response can be employed as a luminescent probe for real-time detection of Cu2+. For the copper-bound azurin, in its oxidised (Cu2+) form the protein displays a strong absorption at 625 nm due to a ligand-to-metal charge-transfer transition involving mainly a sulfur 3p orbital on the Cys112 and Cu dx2−y2 orbital,12 and this absorption disappears when the Cu site is reduced into the reduced (Cu+) form, which suggests that the UCL intensity of UCNPs can be reversibly switched based on modulation of the protein redox states. As shown in Fig. S5,† binding with Cu2+ causes an obvious decrease of upconversion luminescence intensity at 654 nm while subsequent reduction of Cu2+ into the Cu+ form through the addition of a reductant (L-ascorbate acid) could bring the luminescence back to the original level. These results indicate that the azurin/UCNP sensor system shows no response to Cu+. This optical switch observation may provide a novel route for efficient nondestructive biomemory device fabrication.
High selectivity toward other biologically important cation species is necessary for a chemosensing material. The optical responses of the hybrid system were examined for other metal cations in aqueous solution. Since only Cu2+ could bind with apo-azurin and cause a drastic increase in the absorbance peak at ca. 625 nm from the formed metalloprotein (Fig. 6a), the introduction of Cu2+ led to significant changes in the I521/I654 ratio of UCL intensities, and no obvious UCL changes were observed for other metal ions, such as Ag+, Mg2+, Ca2+, Ba2+, Co2+, Ni2+, Fe3+, Pb2+, Zn2+, Cd2+, and Hg2+ (Fig. 6b). Therefore, the azurin–UCNP biosensor system described here showed highly selective ratiometric UCL sensing for Cu2+.
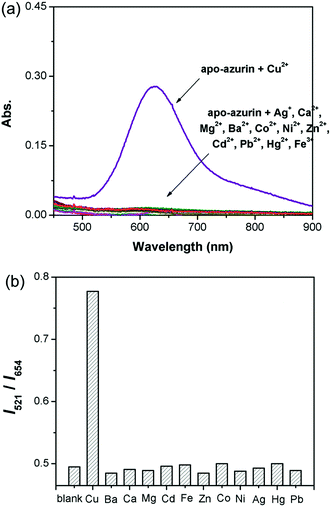 |
| Fig. 6 (a) Changes in the absorption spectra of apo-azurin upon addition of one eq. of various metal ions; (b) the ratio (I521/I654) of UCL intensities at 521 and 654 nm of the sensor system (under excitation from a 980 nm laser) in the presence of various representative metal ions (64 μM Cu2+ and 100 μM other metal ions). | |
Conclusions
In conclusion, zwitterion-coated UCNPs were synthesized through a simple biomimetic approach. By integration of the obtained water-soluble UCNPs with a redox-active copper protein, the label-free UCL sensing of Cu2+ with a ratiometric response was demonstrated, and it possesses attractive features including NIR excitation, absence of a luminescence background, and ease of fabrication. Owing to the facile design, the method could be readily developed to build up sensing and imaging platforms for various targets by fusion of an optical-switchable protein with UCNPs. Furthermore, since proteins have well-defined and predictable structures, and various biological activities, the protein/UCNP system offers an ideal system that should be applicable in a diverse range of areas, such as medical diagnostics, environmental monitoring, and the electronic industry. On the other hand, since zwitterionic groups on NPs will not alter the water H-bonding structure and can minimize nonspecific protein adsorption,13 zwitterion-coated UCNPs can be useful for various biomedical applications.
Experimental
Materials and reagents
All of the chemicals used were of analytical grade and were used without further purification. Rare earth chlorides, oleic acid, and 1-octadecene were purchased from Sigma-Aldrich. Phospholipid distearoylphosphatidylcholine (DSPC) was purchased from Avanti Polar Lipids. The wild-type apo-azurin was expressed and purified as previously reported.12a β-NaYF4:Yb,Er was synthesized according to a previously reported method using rare earth chlorides as a precursor and oleic acid as a stabilizing agent.14
Phospholipid coated UCNPs (DSPC–UCNPs)
The oleic acid capped UCNPs (ca. 0.05 mmol) were dissolved in 0.5 mL of chloroform and then mixed with a chloroform solution (2 mL) containing 12 mg DSPC. The solvent was evaporated slowly, and the obtained film was heated to 75 °C for a while to completely remove chloroform. The film was hydrated with water (5 mL), and the UCNPs became water-dispersible after vigorous sonication and stirring at 75 °C. The solution was centrifuged briefly and the sediment was discarded to remove large aggregates. Excess lipids were purified from DSPC–UCNPs by ultracentrifugation and washing.
Detection
In a 1 × 1 cm quartz cuvette, a freshly prepared solution mixture (2 mL) containing 70 μM apo-azurin and 100 μL of the above nanoparticles in HEPES buffer (10 mM, pH 7.4) was used for Cu2+ detection, into which a small volume (2 μL) of concentrated metal stock solution was added and equilibrated for 5 min before the spectral measurements. To determine the selectivity of the sensor, 100 μM of various metal ions (M2+) including Ag+, Mg2+, Ca2+, Ba2+, Co2+, Ni2+, Fe3+, Pb2+, Zn2+, Cd2+, and Hg2+ was added to the sensor solution individually and the luminescence response was monitored.
Instrumentation
Transmission electron microscopy (TEM) images were taken on a JEOL 2100 Cryo transmission electron microscope with an accelerating voltage of 200 kV. Fluorescence spectra were recorded on a FluoroMax-P fluorimeter (HORIBA Jobin Yvon Inc., Edison, NJ) equipped with a commercial CW IR laser (980 nm). UV/vis spectra were recorded on a Hewlett–Packard 8453 spectrometer.
Conflicts of interest
There are no conflicts to declare.
Acknowledgements
We acknowledge primary financial support from NSFC (No. 21822401 and 21771044) (to L. L.) and the U.S. National Institutes of Health (Grants GM124316 and MH110975) (to Y.L.) for support.
Notes and references
-
(a) F. Wang and X. Liu, Chem. Soc. Rev., 2009, 38, 976–989 RSC;
(b) F. Wang, D. Banerjee, Y. Liu, X. Chen and X. Liu, Analyst, 2010, 135, 1839–1854 RSC;
(c) X. Chen, D. Peng, Q. Ju and F. Wang, Chem. Soc. Rev., 2015, 44, 1318–1330 RSC;
(d) J. Zhou, Q. Liu, W. Feng, Y. Sun and F. Li, Chem. Rev., 2015, 115, 395–465 CrossRef CAS PubMed;
(e) X. Wang, R. R. Valiev, T. Y. Ohulchanskyy, H. Ågren, C. Yang and G. Chen, Chem. Soc. Rev., 2017, 46, 4150–4167 RSC;
(f) G. Tian, X. Zhang, Z. Gu and Y. Zhao, Adv. Mater., 2015, 27, 7692–7712 CrossRef CAS PubMed;
(g) X. Xie, Z. Li, Y. Zhang, S. Guo, A. I. Pendharkar, M. Lu, L. Huang, W. Huang and G. Han, Small, 2017, 13, 1602843 CrossRef PubMed.
-
(a) B. Zhou, B. Shi, D. Jin and X. Liu, Nat. Nanotechnol., 2015, 10, 924–936 CrossRef CAS PubMed;
(b) Y. Li, Z. Di, J. Gao, P. Cheng, C. Di, G. Zhang, B. Liu, X. Shi, L.-D. Sun, L. Li and C.-H. Yan, J. Am. Chem. Soc., 2017, 139, 13804–13810 CrossRef CAS PubMed;
(c) Z. G. Chen, H. L. Chen, H. Hu, M. X. Yu, F. Y. Li, Q. Zhang, Z. G. Zhou, T. Yi and C. H. Huang, J. Am. Chem. Soc., 2008, 130, 3023–3029 CrossRef CAS PubMed;
(d) R. Deng, X. Xie, M. Vendrell, Y. T. Chang and X. Liu, J. Am. Chem. Soc., 2011, 133, 20168–20171 CrossRef CAS PubMed;
(e) Z. Li, H. Liu, H. Li, Y. H. Tsou, Y. Gao, X. Xu, W. Du, L. Wei and M. Yu, Sens. Actuators, B, 2019, 280, 94–101 CrossRef CAS;
(f) J. Zhao, H. Chu, Y. Zhao, Y. Lu and L. Li, J. Am. Chem. Soc., 2019, 141, 7056–7062 CrossRef CAS PubMed;
(g) F. Hu, B. Liu, H. Chu, C. Liu, Z. Li, D. Chen and L. Li, Nanoscale, 2019, 11, 9201–9206 RSC.
-
(a) H. Dong, S. R. Du, X. Y. Zheng, G. M. Lyu, L. D. Sun, L. D. Li, P. Z. Zhang, C. Zhang and C. Yan, Chem. Rev., 2015, 115, 10725–10815 CrossRef CAS PubMed;
(b) Y. Wang, G. Yang, Y. Wang, Y. Zhao, H. Jiang, Y. Han and P. Yang, Nanoscale, 2017, 9, 4759–4769 RSC;
(c) Q. Qin, P. Zhang, L. Sun, S. Shi, N. Chen, H. Dong, X. Zheng, M. Li and C. Yan, Nanoscale, 2017, 9, 4660–4664 RSC;
(d) J. Peng, A. Samanta, X. Zeng, S. Han, L. Yuan, X. Liu and Y.-T. Chang, Angew. Chem., Int. Ed., 2017, 56, 4165–4169 CrossRef CAS PubMed;
(e) Y. Wang, S. Song, J. Liu, D. Liu and H. Zhang, Angew. Chem., Int. Ed., 2015, 54, 536–540 CAS;
(f) J. Liu, J. Bu, W. Bu, S. Zhang, L. Pan, W. Fan, F. Chen, L. Zhou, W. Peng, K. Zhao, J. Du and J. Shi, Angew. Chem., Int. Ed., 2014, 126, 4639–4643 CrossRef;
(g) W. Ren, Y. Zhou, S. Wen, H. He, G. Lin, D. Liu and D. Jin, Chem. Commun., 2018, 54, 7183–7186 RSC.
-
(a) R. Shi, X. Ling, X. Li, L. Zhang, M. Lu, X. Xie, L. Huang and W. Huang, Nanoscale, 2017, 9, 13739–13746 RSC;
(b) D. Yang, P. A. Ma, Z. Hou, Z. Cheng, C. Li and J. Lin, Chem. Soc. Rev., 2015, 44, 1416–1448 RSC;
(c) S. Chen, A. Z. Weitemier, X. Zeng, X. Liu and T. J. McHugh, Science, 2018, 359, 679–684 CrossRef CAS;
(d) S. Han, A. Samanta, X. Xie, L. Huang and X. Liu, Adv. Mater., 2017, 29, 1700244 CrossRef PubMed;
(e) Y. Xiao, L. Zeng, T. Xia, Z. Wu and Z. Liu, Angew. Chem., Int. Ed., 2015, 54, 5323–5327 CrossRef CAS PubMed;
(f) Z. Gu, L. Yan, G. Tian, S. Li, Z. Chai and Y. Zhao, Adv. Mater., 2013, 25, 3758–3779 CrossRef CAS PubMed;
(g) N. M. Idris, M. K. G. Jayakumar, A. Bansal and Y. Zhang, Chem. Soc. Rev., 2015, 44, 1449–1478 RSC;
(h) L. Li and Y. Lu, J. Am. Chem. Soc., 2015, 137, 5272–5275 CrossRef CAS PubMed;
(i) L. Li, R. Zhang, L. Yin, K. Zheng, W. Qin, P. R. Selvin and Y. Lu, Angew. Chem., Int. Ed., 2012, 51, 6121–6125 CrossRef CAS PubMed;
(j) L. Li, P. Wu, K. Hwang and Y. Lu, J. Am. Chem. Soc., 2013, 135, 2411–2414 CrossRef CAS.
-
(a) Y. Liu, M. Chen, T. Cao, Y. Sun, C. Li, Q. Liu, T. Yang, L. Yao, W. Feng and F. Li, J. Am. Chem. Soc., 2013, 135, 9869–9876 CrossRef CAS PubMed;
(b) J. Peng, W. Xu, C. L. Teoh, S. Han, B. Kim, A. Samanta, J. C. Er, L. Wang, L. Yuan, X. Liu and Y.-T. Chang, J. Am. Chem. Soc., 2015, 137, 2336–2342 CrossRef CAS PubMed;
(c) Q. Su, W. Feng, D. Yang and F. Li, Acc. Chem. Res., 2017, 50, 32–40 CrossRef CAS PubMed;
(d) Z. Yang, K. Y. Chu, R. Feng, N. S. R. Satyavolu, M. Xiong and Y. Lu, J. Am. Chem. Soc., 2018, 140, 17656–17665 CrossRef CAS PubMed.
-
(a) J. Liu, Y. Liu, Q. Liu, C. Li, L. Sun and F. Li, J. Am. Chem. Soc., 2011, 133, 15276–15279 CrossRef CAS PubMed;
(b) L. Zhao, J. Peng, M. Chen, Y. Liu, L. Yao, W. Feng and F. Li, ACS Appl. Mater. Interfaces, 2014, 6, 11190–11197 CrossRef CAS PubMed.
-
(a) D. Tu, L. Liu, Q. Ju, Y. Liu, H. Zhu, R. Li and X. Chen, Angew. Chem., Int. Ed., 2011, 50, 6306–6310 CrossRef CAS PubMed;
(b) Y. Liu, S. Zhou, Z. Zhuo, R. Li, Z. Chen, M. Hong and X. Chen, Chem. Sci., 2016, 7, 5013–5019 RSC;
(c) J. Zhao, J. Gao, W. Xue, Z. Di, H. Xing, Y. Lu and L. Li, J. Am. Chem. Soc., 2018, 140, 578–581 CrossRef CAS PubMed;
(d) Y. Cen, Y. M. Wu, X. J. Kong, S. Wu, R. Q. Yu and X. Chu, Anal. Chem., 2014, 86, 7119–7127 CrossRef CAS PubMed.
-
(a) S. V. Wegner, H. Arslan, M. Sunbul, J. Yin and C. He, J. Am. Chem. Soc., 2010, 132, 2567–2569 CrossRef CAS PubMed;
(b) P. Chen and C. He, J. Am. Chem. Soc., 2004, 126, 728–729 CrossRef CAS PubMed.
-
(a) B. E. Kim, T. Nevitt and D. J. Thiele, Nat. Chem. Biol., 2008, 4, 176–185 CrossRef CAS PubMed;
(b) P. Brzezinski and R. B. Gennis, J. Bioenerg. Biomembr., 2008, 40, 521–531 CrossRef CAS PubMed;
(c) E. Madsen and J. D. Gitlin, Annu. Rev. Neurosci., 2007, 30, 317–337 CrossRef CAS PubMed.
-
(a) J. Liu and Y. Lu, J. Am. Chem. Soc., 2007, 129, 9838–9839 CrossRef CAS PubMed;
(b) L. Gao, L. Li, X. Wang, P. Wu, Y. Cao, B. Liang, X. Li, Y. Lin, Y. Lu and X. Guo, Chem. Sci., 2015, 6, 2469–2473 RSC;
(c) L. Shang and S. Dong, J. Mater. Chem., 2008, 18, 4636–4640 RSC;
(d) A. Zhu, Q. Qu, X. Shao, B. Kong and Y. Tian, Angew. Chem., Int. Ed., 2012, 51, 7185–7189 CrossRef CAS PubMed;
(e) H. Chen, J. Zhang, X. Liu, Y. Gao, Z. Ye and G. Li, Anal. Methods, 2014, 6, 2580–2585 RSC;
(f) X. Xu, W. L. Daniel, W. Wei and C. A. Mirkin, Small, 2010, 6, 623–626 CrossRef CAS PubMed.
-
(a) R. Bergonzi, L. Fabbrizzi, M. Licchelli and C. Mangano, Coord. Chem. Rev., 1998, 170, 31–46 CrossRef CAS;
(b) C. J. Chang, J. Jaworski, E. M. Nolan, M. Sheng and S. J. Lippard, Proc. Natl. Acad. Sci. U.S.A., 2004, 101, 1129–1134 CrossRef CAS PubMed.
-
(a) N. M. Marshall, D. K. Garner, T. D. Wilson, Y. G. Gao, H. Robinson, M. J. Nilges and Y. Lu, Nature, 2009, 462, 113–116 CrossRef CAS PubMed;
(b) S. Tian, J. Liu, R. E. Cowley, P. Hosseinzadeh, N. M. Marshall, Y. Yu, H. Robinson, M. J. Nilges, N. J. Blackburn, E. I. Solomon and Y. Lu, Nat. Chem., 2016, 8, 670–677 CrossRef CAS PubMed.
- J. B. Schlenoff, Langmuir, 2014, 30, 9625–9636 CrossRef CAS PubMed.
- Z. Q. Li and Y. Zhang, Nanotechnology, 2008, 19, 345606 CrossRef PubMed.
Footnotes |
† Electronic supplementary information (ESI) available. See DOI: 10.1039/c9na00168a |
‡ These authors contributed equally. |
|
This journal is © The Royal Society of Chemistry 2019 |
Click here to see how this site uses Cookies. View our privacy policy here.