DOI:
10.1039/C9LC00327D
(Paper)
Lab Chip, 2019,
19, 2233-2240
Continuous micellar electrokinetic focusing of neutral species driven by ion concentration polarization†
Received
8th April 2019
, Accepted 27th May 2019
First published on 28th May 2019
Abstract
Ion concentration polarization (ICP) has been broadly applied to accomplish electrokinetic focusing of charged species. However, ICP-based extraction and enrichment of uncharged (neutral) compounds, important for pharmaceutical, biological, and environmental applications, has not yet been reported. Here, we report the ICP-based continuous extraction of two neutral compounds from aqueous solution, by their partition into an ionic micellar phase. Our initial results show that the efficiency of the extraction increases with the concentration of the surfactant comprising the micellar phase, reaching 98 ± 2%, and drops precipitously when the concentration of the target compound exceeds the capacity of the micelles. As a key feature relevant to the practical application of this method, we show that focusing occurs even an order of magnitude below the critical micelle concentration through the local enrichment and assembly of surfactants into micelles, thus minimizing their consumption. To underscore the relevance of this approach to water purification, this method is applied to the extraction of pyrene, a model for polyaromatic hydrocarbons. This approach provides access to a broad range of strategies for selective separation that have been developed in micellar electrokinetic chromatography.
1. Introduction
Here, we report the continuous extraction of neutral (uncharged) compounds from aqueous solution using an electrokinetic process driven by ion concentration polarization (ICP). This outcome is achieved by the partition of these neutral compounds into an ionic micellar phase, thus conferring them with charge. The results of this study are important for two reasons. First, this approach provides access to a wide array of strategies that have been developed in the mature field of micellar electrokinetic chromatography (MEKC) for selective separations. Second, we show that focusing occurs even an order of magnitude below the critical micelle concentration (CMC) through the local enrichment and assembly of surfactants into micelles.
ICP is the simultaneous enrichment and depletion of ions at opposing ends of an ion permselective membrane1 or bipolar electrode2,3 when an electric field is applied across it. An ion selective membrane may be comprised of charged nanopores or nanochannels that electrostatically exclude co-ions. For example, the pores of a cation selective membrane (e.g., Nafion®) are lined with negatively charged moieties and therefore exclude anions. When a voltage is applied across the membrane, cations are selectively transported through, while anions migrate towards the anodic driving electrode, resulting in the formation of an ion depletion zone (IDZ) in the anodic compartment. The low ionic conductivity of the IDZ leads to a strong (>10-fold) local enhancement of the electric field and the formation of concentration and electric field gradients at the IDZ boundary. The non-linear migration of ions in these gradients results in further exclusion of charged species from entering the IDZ – a feature that has been leveraged for focusing and continuous separation of charged species.4
ICP has had a major impact in several areas of application including desalination,5–7 enrichment and separation of trace analytes3,8,9 and bioparticles,8,10 cellular dielectrophoresis,11 regeneration of sensing substrates,12 and mobility shift assays for bioanalysis.13 Most relevant to the current work is the ability of ICP to continuously separate charged species from an aqueous solution in a branched, flow-through microfluidic device (Scheme 1a) – a feature that has been employed for desalination5–7 and for the removal of excess fluid from blood plasma.14 While ICP has proven to be a versatile approach for enriching and separating charged species, neutral compounds are unaffected by the electric field, thereby limiting its application. This limitation is of particular concern for ICP-based water purification, which requires the removal of uncharged contaminants, and for evaluating the purity of food and pharmaceutical products, where the enrichment of uncharged compounds prior to analysis may be crucial.
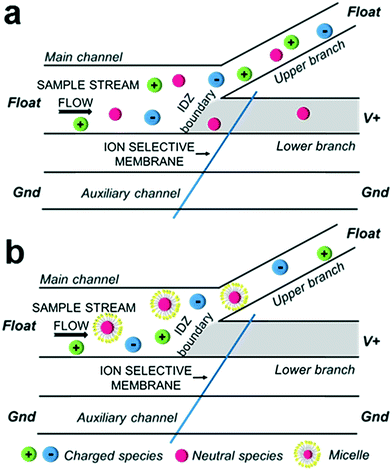 |
| Scheme 1 | |
Our previously published results indicate that ICP-based separation of neutral species is feasible – we observed electrostatic redirection of an uncharged fluorophore in blood plasma, which we attributed to intermolecular interaction of the dye with charged compounds native to blood.14 Here, we leverage this phenomenon to address the need for separation and enrichment of neutral species by their partition into an ionic micellar phase (Scheme 1b).
The addition of ionic surfactants above the CMC has proven to be an effective technique for electrokinetic separation of neutral compounds in capillary electrophoresis.15–18 In MEKC, micelles act as a pseudostationary phase, into which analytes partition based on their affinity for the functional groups comprising the core (e.g., through hydrophobic or pi–pi interactions). In this scenario, compounds that are not ionic under experimental conditions can be incorporated into the micelle and then electrophoretically separated by virtue of the charge on the micelles. MEKC techniques leverage anionic, cationic, and non-ionic surfactants, mixed micelles, and additives, such as organic solvents, ionic liquids, and cyclodextrin, to tightly control guest–host interactions. These strategies accomplish electrokinetic separation of compounds that differ in size, hydrophobicity, charge, and conformation – even distinguishing enantiomers. Most relevant to the work reported here is that MEKC has been utilized for sample preconcentration by field-induced sample stacking and sweeping.16
For example, Palmer et al. reported a method for stacking neutral analytes in micellar capillary electrophoresis.19 By using a background electrolyte (BGE) less conductive than the sample matrix, they achieved field amplification within the BGE, resulting in stacking of the charged micelles. The stacked micelles complex with neutral analytes efficiently and concentrate them locally thus achieving higher sensitivity. This approach was shown to be particularly relevant for separation of nitroaromatic and nitroamine explosives present in seawater. In comparison to MEKC, longer injection times in conjunction with stacking, lead to improved detection limits to sub mg L−1 levels of these compounds. However the resolution in comparison to MEKC, is decreased.20
Here, we demonstrate that in combination with ICP, micelles permit electrokinetic focusing of two uncharged species that partition into the micellar phase, thus leading to their continuous extraction from a flowing solution. We quantify the dependence of the efficiency of this extraction on the input concentration of the surfactant and analyte, the flow rate, and the applied voltage. Critical to the practical application of this method, we have demonstrated neutral analyte extraction at input concentrations of surfactants below the CMC through their local enrichment and assembly into micelles at the IDZ boundary. Collectively, these results demonstrate the capabilities of a new technique for enrichment of neutral targets – continuous micellar electrokinetic focusing (CMEKF).
2. Materials and methods
2.1. Chemicals
Texas Red and CellTracker Green BODIPY dye were obtained from Molecular Probes (Eugene, OR). Sodium dodecylsulfate (SDS), Nafion® perfluorinated resin (20 wt% solution in lower aliphatic alcohols) and Pluronic F-108 (poly(ethylene glycol)-block-poly(propylene glycol)-block-poly(ethylene glycol)) were purchased from Sigma-Aldrich, (St. Louis, MO). Sodium cholate (SC) and pyrene were purchased from Alfa Aesar (Haverhill, MA). Platinum electrodes (99.95%) were purchased from Strem Chemicals (Newburyport, MD). All solutions were made with reagent grade chemicals (Fisher Scientific, Waltham, MA) and diluted with double deionized water (18.2 MΩ cm, Sartorius Arium Pro, Göttingen, Germany) before use. Spent dialysate (from hemodialysis) was obtained from Mary Greely Hospital (Ames, IA) and used without additional purification.
2.2. Device fabrication
The PDMS/glass devices were fabricated using standard soft lithography techniques.21 Briefly, channel molds were patterned using negative photoresist (SU-8 2050, Microchem Corp., Westborough, MD) on a Si substrate. Poly(dimethylsiloxane) (PDMS) (Sylgard 184 elastomer kit, Dow Corning Corp., Midland, MI) was used for microfluidic device fabrication. Nafion® was used as an ion permselective material. A permselective membrane was incorporated into the device employing a mechanical incision/self-sealing method.7 Briefly, using a scalpel, an incision (∼2 mm deep) was made across the lower branch (Scheme 1) of the microchannel approximately 300 μm downstream of the branching junction. A 10 μL droplet of the Nafion® precursor solution was pipetted at one end of the incision, which was gently opened by bending the PDMS monolith to allow the Nafion® solution to wick into it. The Nafion® was then cured in an oven at 95 °C for 10 min, and then any excess Nafion® removed by repeatedly adhering residue-free tape to the PDMS surface. The PDMS layer and glass slide were then treated in an air plasma (PDC-001, Harrick Plasma, Ithaca, NY) for 60 s and finally bonded together. A more detailed description of the device fabrication and dimensions are included in the ESI.† Immediately after bonding, all microfluidic devices were rinsed with deionized water and coated by filling the channels with Pluronic solution and incubating at 4.0 °C for at least 18 h. The Pluronic coating was used to suppress electroosmotic flow. The microfluidic devices were then rinsed with 20.0 mM surfactant solution (SDS or SC) in 10.0 mM phosphate buffer (pH 7.4) for one hour prior to use to ensure uniform wall charge regardless of the surfactant concentration employed in the experiment.
2.3. Electrokinetic separation experiments
Prior to ICP experiments, each device was rinsed with 10.0 mM phosphate buffer solution for 15 min to remove excess surfactant. The rinsing solution was then replaced with the sample solution. A driving voltage was applied across the nanojunction using a DC power supply (HY3005D, Mastech and DIGI360, Electro Industries, Westbury, NY) connected to Pt electrode wires positioned in the main (V+) and auxiliary (Gnd) channel reservoirs (Scheme 1). The concentration, and volume of solution and voltages employed for individual experiments are indicated in the Results and discussion section and in the ESI.†
2.4. Fluorescence measurements
All fluorescence measurements were performed using an Eclipse Ti-S inverted fluorescence microscope (Nikon Industries, New York, NY) equipped with a digital camera (Orca Flash 4.0, Hamamatsu Corp., Bridgewater, NJ). All images were processed using NIS-Elements 4.6 software (Nikon). Fluorescence measurements used for quantitative comparison of species concentrations were background subtracted.
3. Results and discussion
3.1. Response of neutral species to ICP in the absence of surfactants
We first characterized the behavior of charged and neutral species in ICP-based separation in the absence of surfactant. Fig. 1 shows the behavior of charged (red) and neutral (green) fluorophores near the ion permselective membrane (dashed line) in a sodium phosphate buffer solution (10.0 mM, pH 7.4) as it is flowed (left to right) into a branched microfluidic channel. An IDZ formed immediately upon application of a 60.0 V driving voltage (V+) versus ground applied in the auxiliary channel (not shown), and as a result, charged species, represented by Texas Red, were re-directed into the upper branch (Fig. 1a), while a neutral species (BODIPY) was unaffected (Fig. 1b). These results clearly demonstrate that neutral species are not repelled by the IDZ in the absence of surfactant.
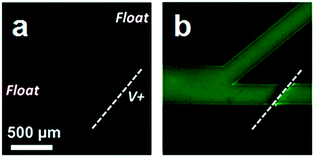 |
| Fig. 1 Fluorescence micrographs showing the location of (a) Texas red (1.0 μM) and (b) BODIPY (50 μM) dyes, which are representative of charged and neutral species, respectively, during ICP in 10.0 mM phosphate buffer (pH 7.4); V+ = 60.0 V. | |
3.2. Surfactant influence on the extraction of neutral compounds by ICP
Next, we investigated the impact of a surfactant present at several distinct concentrations (Fig. 2). It was anticipated that increasing surfactant concentration above the CMC would result in neutral species repulsion from the IDZ and into the upper branch. Fig. 2a is a series of fluorescence micrographs that shows the enrichment and extraction of an uncharged dye (BODIPY, at an initial concentration of 50 μM) as a function of the concentration of the anionic surfactant, sodium dodecyl sulfate (SDS), under an applied voltage of 60.0 V.
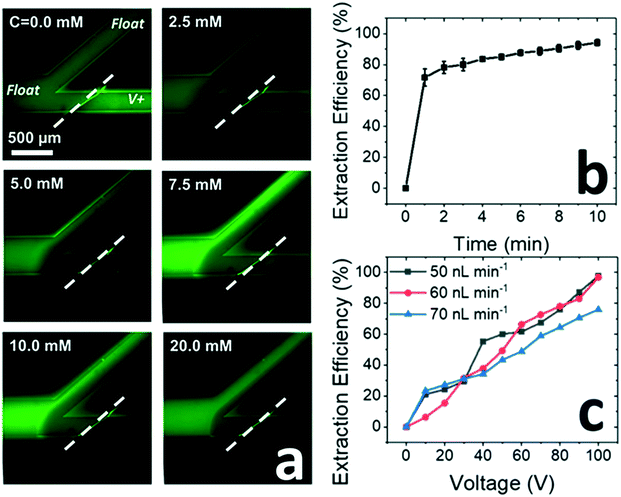 |
| Fig. 2 (a) Fluorescence micrographs showing the location of neutral species (BODIPY, 50 μM input) in solutions having distinct SDS concentrations under an applied voltage of 60.0 V. (b) Extraction efficiencies obtained under the same conditions as in (a) (n = 3). (c) Extraction efficiency as a function of flow rate and applied voltage in 10.0 mM SDS (n = 3). Images employed to calculate efficiency obtained 1 min after the application of each voltage. | |
Extraction efficiency is a commonly used measure in ICP studies to describe the degree of exclusion of a target compound from the IDZ under an applied voltage and is defined here as the percent decrease in background subtracted fluorescence intensity in the lower microfluidic branch downstream of the membrane. Extraction efficiencies above 95% were achieved at an input SDS concentration of 10.0 mM (Fig. 2b). We further observed that modest extraction (about 50%) is achieved at an SDS concentration of 2.5 mM – about half of the CMC (4.53 ± 0.03 mM, see ESI†). These results are significant because they highlight the utility of micelles for electrokinetic focusing of neutral species and demonstrate that onset of extraction occurs below the CMC. This latter outcome is attributed to local enrichment of the surfactant near the IDZ boundary.
Next, fixing the SDS concentration at 10.0 mM, we further investigated the dependence of extraction efficiency on flow rate and applied voltage. After establishing each flow rate, the voltage was decreased from 100.0 V in 10.0 V increments at 1 min intervals (detailed procedure in ESI†). Fig. 2c demonstrates that extraction efficiency is insensitive to flow rate over the range tested (50–70 nL min−1) and increases monotonically with applied voltage to a maximum of 90–95% at 100.0 V. However, by increasing the time interval between voltages from 1 min to 10 min, the extraction efficiency further increased to 94 ± 2% at voltages as low as 60.0 V (at 60 nL min−1) (Fig. S4†). This result is important because it indicates a delay in increased extraction efficiency in response to an increase in electric field strength – a delay which is attributed here to the time required for a higher concentration of SDS micelles to be established upstream of the IDZ boundary. This phenomenon was further investigated and is further addressed in section 3.4.
3.3. Influence of neutral species concentration on extraction efficiency
To ensure high extraction efficiency, the concentration of the micelles must be sufficiently high to avoid saturation with the neutral guest. Fig. 3 shows the extraction of an uncharged dye present at three distinct concentrations, each in 10.0 mM SDS. In the case that the concentration of this neutral species is lower than or similar to that of the micelles, the extraction efficiency is as high as 95% (Fig. 3a and c). The concentration of micelles can be estimated by dividing the surfactant concentration by the aggregation number, which is the number of surfactant molecules per assembled micelle. This value depends sensitively on ionic strength and is on the order of 60–70 for SDS. Therefore, neglecting free surfactant molecules, 10 mM SDS corresponds to a maximum of approximately 0.15 mM micelles. The actual micelle concentration near the IDZ is likely higher, due to its gradual electrokinetic enrichment. When BODIPY concentration surpasses the micelle concentration, the neutral species saturate the micelles, resulting in a sharp decrease in extraction efficiency (Fig. 3b and c). Fig. 3c shows extraction efficiency as a function of neutral species concentration. These results clearly demonstrate the limitation of CMEKF at high guest–host ratios and follow the previous finding in MEKC that the capacity of the extraction is directly proportional to the micelle concentration and the solubilization number.16
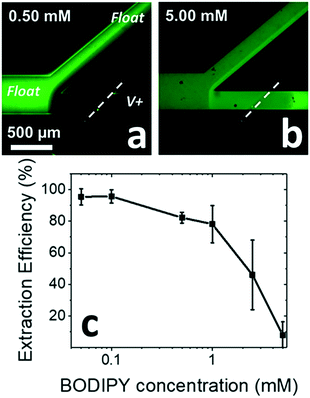 |
| Fig. 3 Fluorescence micrographs showing the extraction of a neutral dye (BODIPY) at two distinct concentrations (a) 0.50 and (b) 5.0 mM, each in 10.0 mM SDS (10.0 mM phosphate buffer). Micrographs were obtained 5 min after applying 60.0 V. Flow rate, 60 nL min−1; (c) extraction efficiency as a function of BODIPY dye concentration for the conditions employed in (a and b) (n = 3). | |
3.4. Local formation of micelles by enrichment of surfactant
The results of Fig. 2 indicate that neutral compounds can be extracted in the presence of surfactant concentrations as low as half of the CMC. Based on these results, we hypothesized that the surfactant is locally enriched to an extent dependent upon the branching geometry at the microfluidic junction – enriching approximately 2-fold at a 1
:
1 branch. This estimate assumes that the flow rate in both branches is equal and that the IDZ is completely contained in the lower branch. To test this hypothesis, the extraction of the uncharged tracer dye (BODIPY) was quantified in devices having a range of channel width ratios between the upper and lower branches (Fig. S2†). Fig. 4 shows neutral species extraction in the presence of only 1.0 mM SDS (well below the CMC) in three devices with distinct ratios of upper branch to lower branch width (1
:
9, 1
:
4, and 1
:
1, respectively) as a function of time (experimental details, ESI†). In the high channel width ratio devices, extraction efficiencies above 90% were observed 20 min after applying the driving voltage (Fig. 4a and b). In contrast, only modest local enrichment and extraction of neutral species occurred in the 1
:
1 ratio device (Fig. 4c). These results demonstrate that by using high channel width ratio devices, surfactant present at an input concentration well below the CMC exhibits an ability to encapsulate and extract BODIPY to an increasing degree over time. This behavior is attributed to gradual accumulation of SDS upstream of the IDZ boundary and the local formation of guest–host pairs (Fig. S6†). Using this strategy, extraction was achieved at as low as a full order of magnitude below the CMC (0.5 mM SDS, Fig. S5†). These results are significant because they indicate that CMEKS can be made effective at low concentrations of surfactant, thus reducing its consumption.
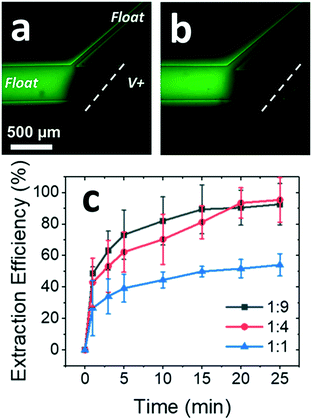 |
| Fig. 4 Fluorescence micrographs showing continuous extraction of BODIPY (initial concentration, 50 μM) in the presence of 1.0 mM SDS in (a) 1 : 9, and (b) 1 : 4 channel width ratio devices in 10.0 mM phosphate buffer solution. Images obtained 20 min after applying 60.0 V. Flow rate, 60 nL min−1; (c) extraction efficiency shown for 1 : 9 (black), 1 : 4 (red), and 1 : 1 (blue) ratio branches (n = 3). | |
Physical and chemical properties of surfactants are of great importance to their ability to bind compounds to be analyzed or separated. To test an alternative surfactant for micelle formation, we chose to use the environmentally benign bile salt, sodium cholate (SC). SC has been used as an additive to enhance the solubilization of hydrophobic compounds in pharmaceuticals,22 to bind polyaromatic hydrocarbons (PAHs) for water and soil purification,23,24 and to discriminate analytes in MEKC.25,26 Due to a relatively flat-shaped hydroxy-substituted steroid portion, and a side-chain with a carboxyl group structure, SC has also proven to be suited for enantiomer separation by MEKC.26–28 In contrast to SDS, SC has a wide range of reported CMC values and its aggregation number (4–16) is strongly dependent on surfactant concentration (10–60 mM).23 Typical concentrations of SC employed for separation range from 25 mM to 100 mM.26,27 Additionally, the solubilization of organic compounds increases with cholate concentration up to 60.0 mM.23 Here, we employ a branched microchannel with a 1
:
9 width ratio to demonstrate local enrichment of 3.0 mM SC to a concentration above the CMC,23,25 at which it can bind a target compound. Fig. 5a is a fluorescence micrograph showing the continuous extraction of BODIPY from a flowing solution at t = 45 min after initiating the driving voltage for the separation. Fig. 5b is a plot of the extraction efficiency (percent of BODIPY repelled from the lower branch) over time. The increase in extraction efficiency over time is attributed to gradual accumulation of SC just upstream of the IDZ. Under these conditions, up to 60% extraction was observed (at t = 45 min). An improved extraction efficiency (65%) was obtained by increasing the driving voltage to 80.0 V (Fig. S8†). However the increased driving voltage is known to increase linear and angular velocity of vortex flow in the IDZ, causing solution mixing, thus disrupting the IDZ boundary.29 This phenomenon is likely contributing to the limited extraction efficiency.
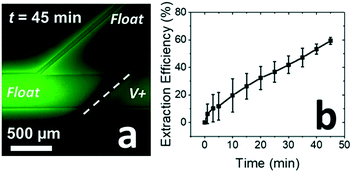 |
| Fig. 5 (a) Fluorescence micrograph showing extraction of BODIPY (initial concentration, 50 μM) in the presence of 3.0 mM SC in 10.0 mM phosphate buffer. Flow rate, 60 nL min−1; V+ = 60.0 V. (b) Plot of extraction efficiency obtained under these conditions as a function time (voltage applied at t = 0; n = 3). | |
In comparison to SDS, the lower extraction efficiency can be explained by the lower mobility of SC micelles leading to poorer repulsion at the IDZ.30–32 Additionally, several physical and chemical parameters such as polarity and the ability to donate or accept hydrogen bonds influences the solvation properties of surfactants, and thus will ultimately impact the neutral species extraction efficiency by ICP.31,32
PAHs found in soil and water pose a serious threat to human health and to water-dwelling species. Remediation of PAHs can be accomplished by micellar solubilization.33 Here, we demonstrate that CMEKF with SC micelles can be employed to both solubilize and continuously extract PAHs from aqueous solution into a concentrated waste stream. It has been reported that the average solubilization number increases with the aggregation number of SC, and therefore, the local concentration of SC is critical to this application. For example, pyrene – employed here as a model PAH – has a solubilization number of 0.059 to 0.18 (pyrene molecules per micelle) for SC aggregation numbers of 8.6 and 16, respectively.23Fig. 6a–c show the solubilization and simultaneous extraction of two neutral compounds (pyrene and BODIPY) from an aqueous solution in the presence of 40.0 mM SC. At early timepoints after applying the driving voltage (Fig. 6a, t = 30 s), bright fluorescent aggregates of pyrene were observed in the flowing solution. Pyrene solubility increased over a period of five minutes (Fig. 6c) as indicated by homogenization of the solution. The extraction efficiency for pyrene achieved at 5 min reached 95%, which may be improved by employing surfactants (or mixed surfactants) that yield a higher partition coefficient for this model PAH.30Fig. 6d–f show the location of BODIPY dye at the same timepoints as in Fig. 6a–c. BODIPY was added to the sample mixture to verify IDZ formation and local enrichment of the surfactant. Further, once the applied voltage was removed, solution with pyrene aggregates and BODIPY again filled the main channel (Fig. S10†). These results are important because they indicate that the reported approach may prove to be a useful route to enhance ICP-based water purification. Additionally, the increased solubilization of pyrene over time supports our earlier conclusion that surfactants are locally enriched upstream of the IDZ boundary.
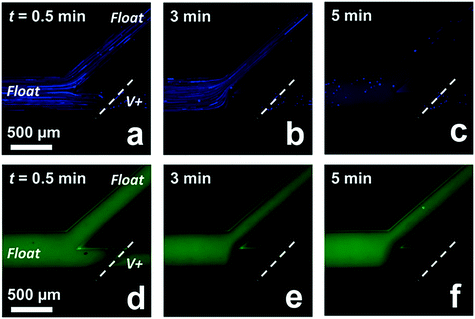 |
| Fig. 6 Fluorescence micrographs showing the continuous focusing and solvation of neutral species (a–c) pyrene (blue, 150 μM input concentration) and (d–f) BODIPY (green, 50 μM input concentration) in the presence of 40.0 mM SC in 10.0 mM phosphate buffer (pH 7.4). Flow rate, 60 nL min−1. V+ = 60.0 V. | |
An important point is that many of the strategies currently under investigation to increase the volumetric throughput of ICP-based desalination can be used in conjunction with CMEKF.5,33 We investigated the ability of CMEKF to proceed in a more complex matrix (spent dialysate) employing a larger channel for increased throughput and microfins to suppress vortex flow patterns.33 The spent dialysate is saline solution (pH 9.15, 13.9 mS cm−2), with added glucose (122 mg dL−1) and uremic toxins (e.g., urea, creatinine, small proteins, indoles, phenols, aliphatic amines) dialyzed from a patient during hemodialysis. Fig. S11a–d† demonstrate the extraction of fluorescently tagged bovine serum albumin (negatively charged) and uncharged species (BODIPY) in the presence and absence of SDS (10 mM) in undiluted spent dialysate under an applied voltage of 120.0 V. These results demonstrate the extraction of albumin from spent dialysate in the absence of the surfactant (Fig. S11a,† 89%), while the neutral species is unaffected by the electric field (Fig. S11b†). After adding surfactant above the CMC to the sample, both charged species and neutral species were repelled into the upper branch with 87 and 85% efficiency, respectively (Fig. S11c and d†). These results indicate, that CMEKF can be used in more complex matrices.
4. Conclusions
In summary, we have shown that continuous electrokinetic separation of a neutral species from an aqueous buffer solution can be achieved using charged micelles in combination with ICP. Importantly, 98 ± 2% extraction efficiency can be achieved (in a device with a 1
:
1 branching ratio) while using SDS concentrations above the CMC. In addition, we demonstrated that high micelle concentration ensures high extraction efficiencies until the micelles become saturated and can accept no further neutral species as guests. A key feature of this approach is that the enrichment of surfactant near to the IDZ allows for local micelle formation, and thus, neutral species extraction can be achieved using low input surfactant concentrations. We have further demonstrated the solubilization and continuous removal of pyrene, a model PAH, from a flowing aqueous solution. Additionally, we have demonstrated that CMEKF can be used in more complex sample matrices like spent dialysate. Based on these results, we anticipate that CMEKF will prove to be a useful method for removal of neutral contaminants during ICP-based purification of water.
Our future work in this area is focused on analytical applications of micelles used in conjunction with ICP. In analytical applications, the specificity of certain micelles to bind targeted compounds can be leveraged to tailor separations. Note that when two neutral solutes are present, if one has a significantly higher micelle-water partition coefficient (Kmw = [solute]micelle/[solute]water), then it will be selectively focused and enriched. Kmw is a highly sensitive indicator of molecular structure and varies over a wide range. For example, Kmw for a series of aliphatic alcohols in SDS increases by an order of magnitude for every two methylene units added (2.4, 18.3, and 193.9 for ethanol, butanol, and hexanol). Common beta blockers atenolol and propranolol have widely differing Kmw for SDS micelles of 389 and 19
055. Indeed, micellar phases that are much more selective than SDS have been devised for specific systems.28,34 Therefore, we anticipate that continuous and selective electrokinetic separation of one or more neutral compounds from a mixture by CMEKF is attainable. In our ongoing studies, we are developing such selective separations by CMEKF and further leveraging a non-branching device35 to achieve ‘static’ electrokinetic focusing of uncharged compounds into discrete bands.
Conflicts of interest
The authors declare no competing financial interests.
Acknowledgements
This material is based upon work supported by the National Science Foundation under Grant No. (1849109). The authors would like to thank Dr. Alexander and Mary Greely Hospital for providing samples of spent dialysate.
References
- M. Li and R. K. Anand, Analyst, 2016, 141, 3496–3510 RSC.
- R. K. Perdue, D. R. Laws, D. Hlushkou, U. Tallarek and R. M. Crooks, Anal. Chem., 2009, 81, 10149–10155 CrossRef CAS PubMed.
- R. K. Anand, E. Sheridan, K. N. Knust and R. M. Crooks, Anal. Chem., 2011, 83, 2351–2358 CrossRef CAS PubMed.
- W. Ouyang, X. Ye, Z. Li and J. Han, Nanoscale, 2018, 10, 15187–15194 RSC.
- B. D. MacDonald, M. M. Gong, P. Zhang and D. Sinton, Lab Chip, 2014, 14, 681–685 RSC.
- K. N. Knust, D. Hlushkou, R. K. Anand, U. Tallarek and R. M. Crooks, Angew. Chem., Int. Ed., 2013, 52, 8107–8110 CrossRef CAS PubMed.
- S. J. Kim, S. H. Ko, K. H. Kang and J. Han, Nat. Nanotechnol., 2010, 5, 297–301 CrossRef CAS PubMed.
- R. Kwak, J. Y. Kang and T. S. Kim, Anal. Chem., 2016, 88, 988–996 CrossRef CAS PubMed.
- H. Jeon, H. Lee, K. H. Kang and G. Lim, Sci. Rep., 2013, 3, 1–7 Search PubMed.
- R. Kwak, S. J. Kim and J. Han, Anal. Chem., 2011, 83, 7348–7355 CrossRef CAS.
- R. K. Anand, E. S. Johnson and D. T. Chiu, J. Am. Chem. Soc., 2015, 137, 776–783 CrossRef CAS PubMed.
- D.-T. Phan, L. Jin, S. Wustoni and C.-H. Chen, Lab Chip, 2018, 18, 3962–3979 RSC.
- L. F. Cheow, A. Sarkar, S. Kolitz, D. Lauffenburger and J. Han, Anal. Chem., 2014, 86, 7455–7462 CrossRef CAS PubMed.
- B. Berzina and R. K. Anand, Anal. Chem., 2018, 90, 3720–3726 CrossRef CAS.
- P. G. Muijselaar, K. Otsuka and S. Terabe, J. Chromatogr. A, 1997, 780, 41–61 CrossRef CAS.
- S. Terabe, Anal. Chem., 2004, 76, 240 A–246 A CrossRef CAS.
- S. I. Wakida, K. Fujimoto, H. Nagai, T. Miyado, Y. Shibutani and S. Takeda, J. Chromatogr. A, 2006, 1109, 179–182 CrossRef CAS PubMed.
- S. Shen, Y. Li, S. I. Wakida and S. Takeda, Environ. Monit. Assess., 2009, 153, 201–208 CrossRef CAS PubMed.
- J. Palmer, N. J. Munro and J. P. Landers, Anal. Chem., 1999, 71, 1679–1687 CrossRef CAS PubMed.
- B. C. Giordano, C. L. Copper and G. E. Collins, Electrophoresis, 2006, 27, 778–786 CrossRef CAS PubMed.
- J. C. McDonald and G. M. Whitesides, Acc. Chem. Res., 2002, 35, 491–499 CrossRef CAS PubMed.
- G. Hancu, B. Simon, A. Rusu, E. Mircia and Á. Gyéresi, Adv. Pharm. Bull., 2013, 3, 1–8 Search PubMed.
- H. Sugioka and Y. Moroi, Biochim. Biophys. Acta, Lipids Lipid Metab., 1998, 1394, 99–110 CrossRef CAS.
- A. Shah, S. Shahzad, A. Munir, M. N. Nadagouda, G. S. Khan, D. F. Shams, D. D. Dionysiou and U. A. Rana, Chem. Rev., 2016, 116, 6042–6074 CrossRef CAS PubMed.
- E. Fuguet, C. Ràfols, M. Rosés and E. Bosch, Anal. Chim. Acta, 2005, 548, 95–100 CrossRef CAS.
- A. L. C. Navazo, M. L. Marina and M. J. Gonzalez, Electrophoresis, 2005, 19, 2113–2118 CrossRef PubMed.
- E. Fuguet, C. Ràfols, E. Bosch and M. Rosés, Electrophoresis, 2002, 23, 93–101 CrossRef.
- K. Otsuka and S. Terabe, J. Chromatogr. A, 2000, 875, 163–178 CrossRef CAS.
- S. J. Kim, Y.-C. Wang, J. H. Lee, H. Jang and J. Han, Phys. Rev. Lett., 2007, 99, 1–9 Search PubMed.
- L. Hao, R. Lu, D. G. Leaist and P. R. Poulin, J. Solution Chem., 1997, 26, 113–125 CrossRef CAS.
- E. Fuguet, C. Ràfols and M. Rosés, Langmuir, 2003, 19, 6685–6692 CrossRef CAS.
- E. Fuguet, C. Ràfols, E. Bosch and M. Rosés, Langmuir, 2003, 19, 55–62 CrossRef CAS.
- M. K. Gupta, R. K. Srivastava and A. K. Singh, E-J. Chem., 2010, 7, 73–80 CrossRef CAS.
- K. L. Rundlett and D. W. Armstrong, Anal. Chem., 1995, 67, 2088–2095 CrossRef CAS PubMed.
- U. Tallarek, R. K. Perdue, R. M. Crooks, D. Hlushkou and D. R. Laws, Anal. Chem., 2009, 81, 8923–8929 CrossRef PubMed.
Footnote |
† Electronic supplementary information (ESI) available. See DOI: 10.1039/c9lc00327d |
|
This journal is © The Royal Society of Chemistry 2019 |