DOI:
10.1039/C9FO01326A
(Paper)
Food Funct., 2020,
11, 392-403
Beneficial effects of combination therapy of phloretin and metformin in streptozotocin-induced diabetic rats and improved insulin sensitivity in vitro
Received
20th June 2019
, Accepted 2nd December 2019
First published on 3rd December 2019
Abstract
The GLUT4 and PI3K/AKT signaling pathways are the key sensors of energy status and they regulate glucose and lipid metabolism. Phloretin activates the PI3K/AKT pathway by promoting GLUT4 translocation and expression, thereby improving glucose consumption and tolerance. As metformin can regulate glucose metabolism, we hypothesized that phloretin can amplify its gluco-regulatory effects. Male Sprague Dawley rats were fed with a high-fat and high-sugar diet for 8 weeks and injected with a low dose of streptozotocin to induce type 2 diabetes. The diabetic rats were randomized to receive phloretin (100 mg kg−1 d−1), metformin (250 mg kg−1 d−1), or phloretin + metformin via oral gavage for another 4 weeks. Random blood glucose, serum insulin, free fatty acid, total cholesterol, triglyceride, and low-density lipoprotein levels were detected in type 2 diabetic rats. Hematoxylin–eosin and Oil Red O staining were used to observe the pathological changes in the liver, pancreas, and adipose tissues of type 2 diabetic rats. The expression levels of IRS-1, PI3K, P-AKT, and GLUT4 in skeletal muscle were detected using western blotting. Phloretin plus metformin improved fasting blood glucose levels, glucose tolerance, and insulin sensitivity in type 2 diabetic rats. In addition, this combination reduced lipid accumulation, improved the pathological changes in the liver, pancreas, and adipose tissue, and increased IRS-1, PI3K, P-AKT, and GLUT4 expression in skeletal muscle and the liver of type 2 diabetic rats. Thus, phloretin can be used in a potential combination therapy with metformin for the prevention and rescue of type 2 diabetes.
1. Introduction
Diabetes, in particular type 2 diabetes (T2D), is increasing at an alarming rate and is one of the largest global health emergencies of the 21st century.1 Each year, the number of people living with this condition is increasing, which can result in life-changing complications. The International Diabetes Federation has indicated that 425 million adults are estimated to currently have diabetes and this number may double in many regions by 2045. At the same time, a further 352 million people with impaired glucose tolerance are at high risk of developing diabetes.2 Diabetes is a metabolic disorder caused by various etiologies, whereas T2D is mainly caused by decreased glucose transport into muscle and fat cells and increased hepatic glucose output owing to perturbations in insulin secretion or insulin resistance (IR).3,4
Metformin has been the gold standard in the treatment of type 2 diabetes for over two decades.5 It is the first-choice drug for monotherapy of patients newly diagnosed with T2D and is also recommended in combined treatment when monotherapy is no longer effective. Combination drugs include sulfonylurea, incretin, flozin, or insulin, irrespective of the number of insulin injections per day.6 Metformin is a relatively safe drug, which does not cause hypoglycemia or affect body weight.7 The mechanism of metformin action does not involve stimulation of insulin secretion by the beta cells of the pancreas, but inhibition of hepatic glucose production and increase in the insulin sensitivity of peripheral tissues, such as muscle and fatty tissues.8–10
Phloretin (2′,4′,6′-trihydroxy-3-(4-hydroxyphenyl)-propiophenone) is a dihydrochalcone found in apples, pears, and strawberries.11,12 Phloretin exerts wide biological activities, including antioxidant, antitumor, and anti-inflammatory properties, and alleviates vascular endothelial dysfunction and liver injury.13–16 We have previously demonstrated that phloretin provides protection from the development of T2D in rats and can be used for its treatment. We showed that phloretin can improve glucose consumption and glucose tolerance, which were associated with the stimulation of GLUT4 translocation and expression.17
As the mechanisms of action of metformin and phloretin differ, dual therapy with both drugs leads to better treatment for T2D than monotherapy with either drug. Surprisingly, to the best of our knowledge, this has never been investigated. Therefore, the present study was designed to compare the effects of phloretin and metformin, alone and in combination, in treating glucose disorders and insulin resistance in vivo and in vitro. Furthermore, the molecular mechanism of the two drugs in combination was also evaluated in L6 myotubes and BRL-3A cells.
2. Experimental
2.1 Chemicals and materials
Phloretin (Lot no. XC20140403, purity > 99%) was purchased from Xi'an Plants of Grass Technology Co. Ltd (Shaanxi, China). Metformin hydrochloride, 6-[4-(2-piperidin-1-ylethoxy)phenyl]-3-pyridin-4-ylpyrazolo[1,5-a]pyrimidine (CAS Number: 866405-64-3, purity > 99%), was purchased from Sigma-Aldrich Inc. (St Louis, MO, USA). Streptozotocin (STZ) was purchased from Sigma Chemical Co. STZ was dissolved in 0.1 mol L−1 sodium citrate hydrochloric acid buffer, pH 4.5, when required. The blood glucose meter and blood glucose test strips were obtained from Johnson & Johnson, Co. (NewBrunswick, NJ, USA). All other chemicals were of the highest commercially available grade.
2.2 Animals and treatment
All the studies on animals were performed in accordance with the Guidelines for the Care and Use of Laboratory Animals. The experimental protocol (20150701) involving animals was reviewed and approved by the Institutional Animal Care and Use Committee of the Fourth Military Medical University. Four-week-old, healthy, specific pathogen-free, male Sprague Dawley (SD) rats weighing 118 ± 10 g were supplied by the Experimental Animal Center of Fourth Military Medical University (Xi'an, People's Republic of China). All rats were housed internally in neat animal rooms maintained at constant temperature (23 °C ± 2 °C) and humidity (55% ± 10%), and a 12 h light/dark cycle. The rats had unrestricted access to food and water.
2.3 Experimental design
After feeding for 3 days,18 the rats were randomly divided into two groups: (1) Con group (n = 15): fed with a normal diet and received 0.5% (w/v) CMC-Na as the control (after STZ injection); (2) vehicle group (n = 60): fed with a high-fat and high-sugar diet (HFS diet contained 10% lard, 20% sucrose, 0.25% bile salt, and 1% cholesterol) for 8 weeks.19,20 After that, the vehicle rats were starved overnight and injected once intraperitoneally (ip) with 15 mg kg−1 of STZ (freshly dissolved in sodium citrate buffer, pH 4.0, prepared on ice, and protected from light) to induce diabetes.21 Seventy-two hours after injection, the vehicle rats with blood glucose level ≥11.1 mmol L−1 were considered as T2D rats and used in further experiments.22,23 Then, the T2D rats were randomly divided into four groups: (1) TD group (n = 15) received 0.5% (w/v) CMC-Na as vehicle; (2) Ph group (n = 15) received 100 mg kg−1 phloretin (dissolved in 0.5% [w/v] CMC-Na, after STZ injection);17 (3) MT group (n = 15) received 250 mg kg−1 metformin (dissolved in 0.5% [w/v] CMC-Na, after STZ injection); (4) CB group (n = 15) received a combination of metformin and phloretin in the same aforementioned doses (dissolved in 0.5% [w/v] CMC-Na, after STZ injection). Each group was fed through gastric gavage for 4 weeks to verify the hypoglycemic effect. Body weight, food consumption, and postprandial blood glucose (PBG) levels were monitored every week. The entire experimental scheme has been summarized in a flow chart (Fig. 1).
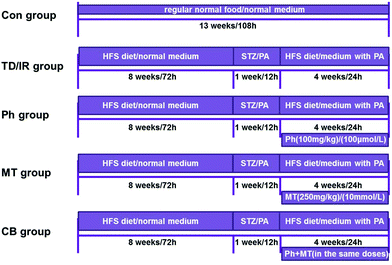 |
| Fig. 1 The time line of the animal and cell experimental process. The width of the rectangle represents the content of the rats’ feed or the medium of cells, the length represents the time course, and the rectangle under the line represents the treatment of rats or cells. | |
At the end of administration, blood samples were collected for separating serum and blood after a 12 h fast. Then, all rats were killed, and the organs and tissues were frozen and prepared for testing. A part of the pancreas was fixed with 4% paraformaldehyde, embedded in paraffin, and sequentially sectioned. After hematoxylin/eosin (HE) staining and double-immunofluorescence labeling staining, a section of each block was observed at 400× magnification.24
2.4 Oral glucose tolerance test (OGTT)
At the end of 12 weeks, all rats were fasted for 12 h before the test. They were administered 2 g kg−1 50% glucose solution by gavage. Then, blood samples were collected from the tail vein at 0, 15, 30, 60, 90, and 120 min after glucose loading for measurement using the blood glucose meter. The areas under the curve (AUC) values for glucose were calculated using the trapezoidal method.25
2.5 Serum analyses
PBG in each group was monitored using a blood glucose meter. Glycated hemoglobin (GHb) was measured using commercial kits (Axis-Shield, Norway). Fasting serum levels of free fatty acids (FFAs), triacylglycerol (TG), total cholesterol (TC), and low-density lipoprotein (LDL) in each group were assessed using the corresponding commercial kits (Biosino, Beijing, China). Fasting serum insulin levels were measured in duplicate using a commercial radioimmunoassay kit (Huaying, Beijing, China). The homeostatic model assessment of the insulin resistance index (HOMA-IR) was used to assess insulin resistance.26 The HOMA-IR was calculated using a standard formula from fasting plasma insulin and glucose levels as follows: HOMA-IR = [Insulin (μU mL−1) × glucose (mmol L−1)]/22.5.27
2.6 Cell culture
The myoblast cell line (L6) and BRL-3A rat liver cells were purchased from the cell bank of the Chinese Academy of Sciences (Shanghai, China). The cells were maintained at 37 °C in the presence of 95% O2 and 5% CO2 in low-glucose Dulbecco's modified Eagle's medium (DMEM) or minimal essential medium (MEM) supplemented with 10% fetal bovine serum (FBS) and antibiotics. The culture media were routinely changed after every 2 days, and the cells were passaged by trypsinization before reaching confluence. L6 skeletal muscle cells were considered to be L6 myotubes after 96 h of differentiation in a differentiation medium.28
The cells were also divided into five groups for different treatments (Fig. 1). The L6 cells were stimulated with 0.4 mmol L−1 palmitic acid (PA) for 12 h and the BRL-3A cells were stimulated with 0.2 mmol L−1 PA for 12 h to induce insulin resistance.
2.7 Cell viability assay
The BRL-3A cells were incubated with dimethyl sulfoxide (DMSO) or test compounds for 36 h. Phosphate buffered saline (PBS)-buffered 3-(4,5-dimethylthiazol-2-yl)-2, 5-diphenyltetrazolium bromide (MTT) (10 μL, 5 mg mL−1) solution was added to each well and the plates were incubated at 37 °C for another 4 h. Then, the medium was discarded and the formazan blue formed in the cells was dissolved in DMSO. The optical density was measured using a microplate spectrophotometer (Thermo Fisher Scientific, Waltham, MA, USA) at 570 nm.
2.8 Glucose consumption assay
The L6 cells and BRL-3A cells were treated separately in groups for 24 h. The glucose concentration in the medium was determined using the glucose oxidase and peroxidase (GOD-POD) method.29 The glucose concentration in the wells with cells was subtracted from the glucose concentration in the blank wells to obtain the amount of glucose consumed.
2.9 Western blot analysis
After treatment, rats or cells in each group were used for the preparation of protein extracts. Total proteins and membrane proteins were extracted from liver tissues, skeletal muscle tissue, L6 myotubes, or BRL-3A cell samples using the radioimmunoprecipitation assay (RIPA) buffer (Beyotime, Shanghai, China) supplemented with phenylmethanesulfonyl fluoride (1 mmol L−1; Sigma) and a protease and phosphatase inhibitor cocktail (100×; Thermo Fisher Scientific, Waltham, MA, USA). Equal amounts of protein samples were separated using 10% (v/v) sodium dodecyl sulfate-polyacrylamide gel electrophoresis (SDS-PAGE) and transferred onto a polyvinylidene difluoride (PVDF) membrane. After washing, the membranes were incubated overnight at 4 °C with one of the following primary antibodies: rabbit polyclonal antibodies against AKT, P-AKT (Thr308), GLUT4, PI3K (p85), and IRS-1 (1
:
1000; Abcam, Cambridge, UK), and actin (1
:
1000; CMCTAG, San Diego, CA, USA). After further washing, the membranes were incubated for 1 h with the corresponding horseradish peroxidase-conjugated secondary antibodies (anti-rabbit IgG or anti-mouse IgG, 1
:
10
000; ComWin Biotech Co., Ltd, Beijing, China). The immunoreactive bands were visualized using an enhanced chemiluminescent substrate (Thermo Fisher Scientific, Rockford, IL, USA) with a GE ImageQuant LAS 4000 mini (GE Healthcare, Waukesha, WI, USA). The intensity of the protein bands was quantitated using a Gel Doc XR system (Bio-Rad, Hercules, CA, USA).
2.10 Confocal laser scanning fluorescence microscopy
The L6 myotubes or BRL-3A cells were grown on glass cover slips. After the treatment, the cells were washed with PBS (Shenggong, Shanghai, China), fixed for 30 min at room temperature with 4% (w/v) paraformaldehyde (Boster, Wuhan, China), permeabilized with 1% (v/v) TritonX-100 in PBS for 10 min at room temperature, and then blocked with goat serum for 1 h.30 After washing, the cells were incubated overnight with an anti-GLUT4 antibody (1
:
100) in goat serum and then incubated for 1 h with a fluorescein isothiocyanate-conjugated secondary antibody (goat anti-rabbit IgG, Cy3 Conjugated, 1
:
100, ComWin Biotech Co.). The cover slips were mounted on glass slides with antifade mounting media (Invitrogen, Carlsbad, CA, USA), and the images were collected using an Olympus confocal microscope model FV1000 at 800 × 600 pixel resolution with a 60× objective lens (Carl Zeiss, Oberkochen, Germany). No fluorescence crossover was observed between the channels, and images were collected separately using the appropriate laser excitation wavelength and then merged.31
2.11 Statistical analysis
Values are expressed as mean ± SE. Two-way analysis of variance (ANOVA) was used to test homogeneity for variance and Tukey's test was used to determine significant differences between multiple groups. P < 0.05 was considered statistically significant.
3. Results
3.1 Phloretin combined with metformin ameliorates glucose metabolism in STZ-induced T2D rats
3.1.1 Phloretin combined with metformin reduces body weight in STZ-induced T2D rats.
A T2D model was first established by feeding rats with a high-fat and high-sugar diet/STZ. As shown in Fig. 2A, the high-fat and high-sugar diet resulted in body weight gain during the modeling period in a time-dependent manner. STZ treatment significantly decreased the body weight in the TD group compared to the Con group (P < 0.05), in which the body weights continued to increase steadily. However, the combination of phloretin and metformin induced a notable increase in body weight (P < 0.05). Compared to the TD group, the other treatment groups did not show any significant difference during the 4 weeks of treatment (P > 0.05).
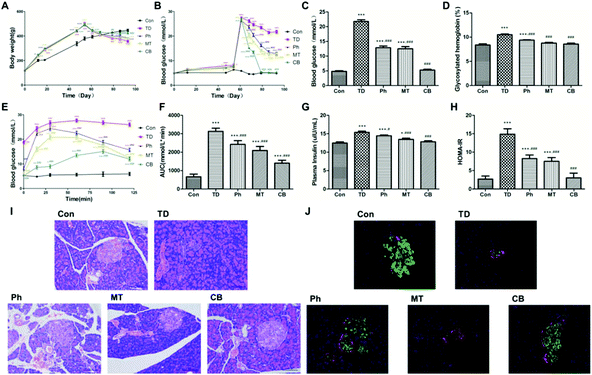 |
| Fig. 2 Phloretin combined with metformin ameliorates glucose metabolism and insulin resistance in STZ induced T2D rats. (A) Evolution of the body weight of each group; (B) evolution of the postprandial blood glucose of each group; (C) postprandial blood glucose of each group at the end of experiment; (D) glycosylated hemoglobin level of each group at the end of experiment; (E and F) OGTT and AUC of each group; (G and H) serum insulin content and the insulin resistance index of each group at the end of experiment; (I) HE staining of the islets (×200) of each group; (J) immunofluorescence labeling of the islets (×100) with insulin (red), glucagon (green) and DAPI (blue) in each group. Values were expressed as means ± SEM (n = 6). *p < 0.05, **p < 0.01, ***p < 0.001 vs. the Con group; #p < 0.05, ##p < 0.01, ###p < 0.001 vs. the TD group. | |
3.1.2 Phloretin combined with metformin reduced PBG and glycosylated hemoglobin levels in STZ-induced T2D rats.
Fig. 2B shows that the initial PBG level did not differ among the groups during 4 weeks of consumption of the high-fat and high-sugar diet (P > 0.05). Compared to the Con group, the PBG level in the TD group and treatment groups increased significantly after intraperitoneal STZ injection (P < 0.001). However, compared to the TD group, phloretin and metformin treatment reduced PBG levels in weeks 10 to 13 (P < 0.001). Furthermore, the PBG level in the CB group was lower than that in Ph and MT groups, which was almost identical to that in the Con group (P > 0.05). Eventually, at the end of the experiment, the PBG level in the CB group was 76% of that in the TD group (P < 0.001), whereas that in the Ph and MT groups decreased by 41% and 42%, respectively (Fig. 2C).
Administration of the combination of phloretin and metformin significantly reduced the glycosylated hemoglobin level compared to that in unadministered rats (P < 0.001). The GHb level in the rats of the Ph and MT groups also decreased, although the reduction rate was lesser than that in the CB group (Fig. 2D).
3.1.3 Phloretin combined with metformin ameliorated glucose tolerance in STZ-induced T2D rats.
Glucose homeostasis was evaluated using the OGTT. As shown in Fig. 2E, after the intake of glucose, the blood glucose level of the TD group increased rapidly and remained at a level higher than that of the Con group, which was considerably low. Compared to the TD group, the Ph and MT groups showed a sharp decline in blood glucose levels after the 30 min peak. However, it did not return to the baseline after 120 min in the Ph and MT groups. Interestingly, the blood glucose level in the CB group increased slowly; the blood glucose level peaked at 90 min and then decreased rapidly. The corresponding AUC profile is shown in Fig. 2F. Compared to the TD group, the AUC for glucose in the Ph, MT, and CB groups showed a significant decrease of 23%, 33%, and 55%, respectively. These findings indicated that the combination therapy of phloretin and metformin had beneficial effects on impaired glucose tolerance in T2D rats.
3.1.4 Phloretin combined with metformin ameliorated serum insulin levels in STZ-induced T2D rats.
Insulin resistance, a hallmark of T2D, is usually accompanied by hyperinsulinemia. Improvement in the serum insulin levels was important for the improvement of insulin resistance. According to our results, the TD group had markedly higher levels of insulin than the Con group, and the insulin levels were significantly reduced by phloretin and metformin treatment. Among all groups, the insulin levels of the CB group showed the most obvious reduction (Fig. 2G). Fig. 2H shows that compared to the corresponding TD group, insulin resistance was also significantly improved after Ph and MT treatment. The combination therapy showed a significantly larger effect on the HOMA-IR.
3.1.5 Phloretin combined with metformin improved islet injury in STZ-induced T2D rats.
HE staining showed that pancreatic islets from Con rats were regularly round or elliptical and had clear boundaries, and the islet cells exhibited normal architecture, namely normal size and shape with an eosinophilic cytoplasm and centrally placed nuclei. Compared to the Con rats, the number of islets in the TD group was obviously lower; furthermore, the islets were atrophied and had no clear boundaries. However, phloretin and metformin treatments markedly improved the pathological damage, and well-formed regenerating islets were observed in rats treated with both drugs in combination, indicating more protection against pancreatic tissue damage (Fig. 2I).
As shown in Fig. 2J, insulin was labeled red and glucagon was labeled green in the islets of each group. Secretion of insulin was significantly decreased in the TD group. Phloretin and metformin ameliorated the reduction of insulin secretion, especially in the CB group. However, there was no significant difference in glucagon secretion in other groups.
3.2 Phloretin combined with metformin ameliorated lipid metabolism and lipid accumulation in STZ-induced T2D rats
3.2.1 Phloretin combined with metformin ameliorated lipid metabolism in STZ-induced T2D rats.
To determine whether phloretin and metformin improved hyperlipidemia, we investigated the levels of serum lipid in each group. Compared to rats fed with a normal diet, the levels of FFA, TG, TC, LDL, and HDL in the TD group increased significantly (P < 0.001). The plasma lipid profile improved significantly (P < 0.05) when phloretin and metformin were administered. Furthermore, the levels of FFA, TG, TC, LDL, and HDL decreased more in the combination group than in groups treated with either drug alone (P < 0.001), which were 25.6%, 44.5%, 69.0%, 77.1%, and 41.1% lower than those in the TD group, respectively (Fig. 3A).
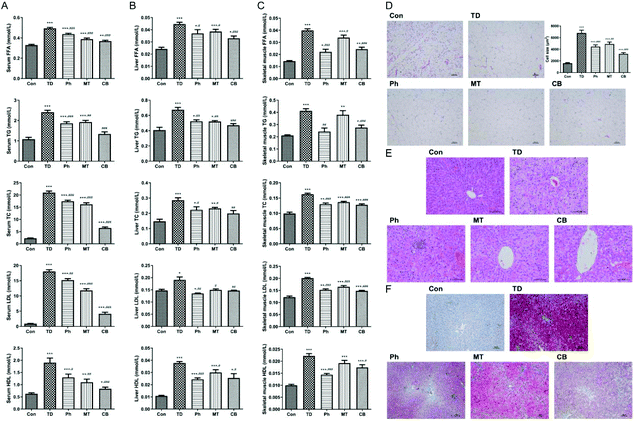 |
| Fig. 3 Phloretin combined with metformin ameliorates lipid metabolism and lipid accumulation in STZ induced T2D rats. (A) FFA, TG, TC, LDL and HDL levels in serum; (B) FFA, TG, TC, LDL and HDL levels in the liver; (C) FFA, TG, TC, LDL and HDL levels in skeletal muscles; (D)HE staining of the adipose tissue (×100) and fat cell size comparison of each group; (E) HE staining of the liver tissue (×200) of each group; (F) Oil Red O staining of the liver tissue (×100) of each group. Values were expressed as means ± SEM (n = 6). *p < 0.05, **p < 0.01, ***p < 0.001 vs. the Con group; #p < 0.05, ##p < 0.01, ###p < 0.001 vs. the TD group. | |
Next we detected these corresponding indicators in the liver and skeletal muscle to ensure the lipid metabolism improvement of phloretin combined with metformin. As shown in Fig. 3B and C, compared with the Ph and MT groups, the lipid metabolism disorder was significantly improved in the CB group of T2D rats.
3.2.2 Phloretin combined with metformin improved the pathological changes in adipose tissue in STZ-induced T2D rats.
Adipocytes are round or polygonal, and the centers of the cells contain a large lipid droplet; the cytoplasm and nucleus are located at the periphery of the cell. According to the results of HE staining of adipose tissue (Fig. 3D), the adipocytes of the Con group were neatly arranged and uniform in size, while the entire volume of the adipocytes in the TD group was significantly large. The size of the cells was different, and even large cells were observed. The pathological damage was improved to some extent in the Ph and MT groups. Despite the presence of large cells in the tissue, they were few in number and the cells were arranged neatly. The most obvious improvement was observed in the CB group.
3.2.3 Phloretin combined with metformin improved the pathological changes and lipid accumulation in the liver of STZ-induced T2D rats.
To directly assess the effects of phloretin and metformin on liver morphology, rat liver tissue was stained with HE. As shown in Fig. 3E, a typical architecture of the hepatic lobule was observed in the Con group. The hepatocytes were arranged in cords and radiated around the central vein; they had branches, which anastomosed each other into meshes. In contrast, T2D rats showed degenerative changes in the hepatocytes, such as hepatic steatosis, cellular swelling, and cell death. After treatment, the degeneration of the hepatocytes was markedly alleviated, and the architecture of the hepatic lobules returned to the nearly normal state; hepatic steatosis, cellular swelling, and cell death were partially recovered, especially in the CB group.
Next, we verified the effects of phloretin and metformin on lipid accumulation in liver tissues using Oil Red O staining, which stains the lipid droplets red. As shown in Fig. 3F, Oil Red O staining revealed that numerous fat droplets and microvesicles were observed in the TD group compared to the Con group, whereas only smaller lipid droplets were observed in the Ph and MT groups, especially in the CB group.
3.3 Phloretin combined with metformin promoted glucose consumption and suppressed gluconeogenesis in skeletal muscle via AKT/GLUT4 signaling pathways
To investigate the effect of phloretin and metformin on glucose consumption in skeletal muscle, the protein levels of key markers regulating glucose metabolism in skeletal muscle were investigated. As shown in Fig. 4A, compared to the TD group, phloretin and metformin upregulated P-AKT, PI3K, IRS-1, and GLUT4 (P < 0.05) in the skeletal muscle of T2D rats, whereas no changes in the total AKT protein level was observed (P > 0.05). The upregulation was most obvious in the CB group, in which the P-AKT, PI3K, IRS-1, and GLUT4 levels increased by 286.8%, 53.2%, 101.3%, and 292.4%, respectively.
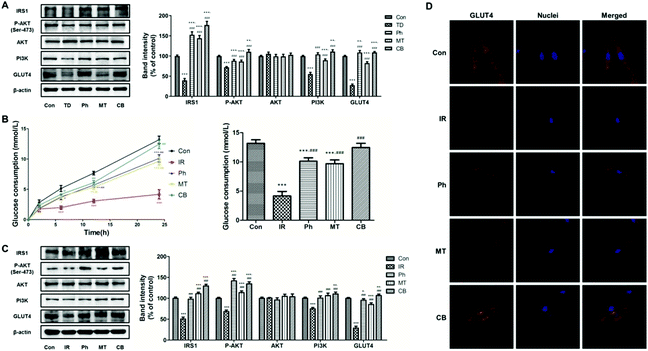 |
| Fig. 4 Phloretin combined with metformin promotes glucose consumption and suppresses gluconeogenesis in skeletal muscle via Akt/GLUT4 signaling pathways. (A) Western blotting was used to analyze IRS-1, P-AKT, AKT, PI3K, and GLUT4 protein expression in the skeletal muscle of the STZ induced T2D rats; (B) evolution of the glucose consumption in L6 myotubes of each group and the glucose consumption of each group after 24 h of different treatments; (C) western blotting was used to analyze IRS-1, P-AKT, AKT, PI3K, and GLUT4 protein expression in the L6 myotubes; (D) immunofluorescence staining of GLUT4 in L6 myotubes. Values were expressed as means ± SEM (n = 6 or n = 5). *p < 0.05, **p < 0.01, ***p < 0.001 vs. the Con group; #p < 0.05, ##p < 0.01, ###p < 0.001 vs. the TD/IR group. | |
Based on previous studies, glucose consumption in PA-induced L6 myotubes was used to assess whether the phloretin and metformin combination can affect glucose metabolism. Phloretin and metformin ameliorated the reduction of glucose consumption caused by insulin resistance in a time-dependent manner, especially in the CB group (Fig. 4B). After 24 h of different treatments, similar results were obtained, and the glucose consumption in the CB group was almost identical to that in the Con group (P > 0.05). Furthermore, the results of western blotting of the L6 myotubes showed similar changes in the P-AKT, PI3K, IRS-1, and GLUT4 expression (Fig. 4C). Phloretin combined with metformin significantly increased the expression of P-AKT, PI3K, IRS-1, and GLUT4 (P < 0.001).
Next, we used immunofluorescence staining to determine whether phloretin combined with metformin enhanced muscle glucose consumption via GLUT4. The L6 myotubes were stained with Cy3 (red) for determining GLUT4 expression and the nuclei were stained with Hoechst 33
342 (blue). The expression of GLUT4 in the IR group was lower than that in the Con group. However, phloretin and metformin treatments further decreased the GLUT4 expression, especially when the two drugs were administered together. Interestingly, phloretin promoted the translocation of GLUT4. As shown in Fig. 4D, GLUT4 was more distributed in the cell membrane in the Ph and CB groups, especially in the CB groups, which indicated improvement in glucose consumption.
3.4 Establishment of an insulin resistance model in BRL-3A cells
To investigate whether phloretin and metformin can promote glucose consumption in BRL-3A cells, an insulin resistance model was first established. The cell counting kit-8 (CCK-8) method was used to observe the effect of different concentrations and times of PA on the proliferation of BRL-3A cells. As shown in Fig. 5A, 0.025–0.2 mmol L−1 PA and the blank reagent had no effect on the proliferation of BRL-3A cells within 36 h (P > 0.05). Next, a glucose consumption assay was used to determine the optimal concentration of PA required to induce insulin resistance in BRL-3A cells. Compared to the Con group, glucose consumption decreased when cells were treated with 0.4 mmol L−1 PA for 2 h, or with 0.25 mmol L−1 and 0.2 mmol L−1 PA for 12 h (P < 0.001, Fig. 5B). However, previous cytotoxicity experiments (Fig. 5A) showed that 0.4 mmol L−1 and 0.25 mmol L−1 PA inhibited BRL-3A cell proliferation. Therefore, 12 h incubation with 0.2 mmol L−1 PA was selected for inducing insulin resistance in BRL-3A cells. Fig. 5C and D show that long-term basic and insulin-stimulated glucose consumption was lower in the IR group than in the Con group after 48 h, which indicated that the insulin resistance model was stabilized within 48 h.
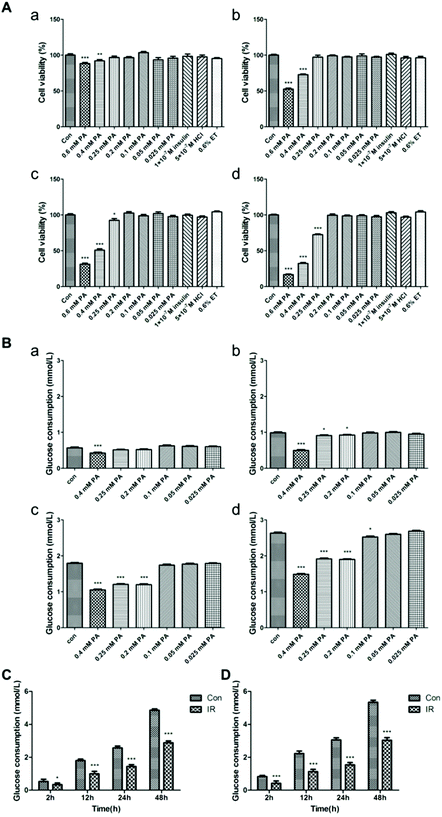 |
| Fig. 5 Establishment of an insulin resistance model in BRL-3A cells. (A) Effect of different concentrations and times of PA or insulin on cytoactivity in BRL-3A cells (n = 8); (B) effect of different concentrations and times of PA on glucose consumption in BRL-3A cells (mmol L−1, n = 12); (C) long-term basic glucose consumption changes in IR cells (mmol L−1, n = 6); (D) long-term insulin-stimulated glucose consumption changes in IR cells (mmol L−1, n = 6). Values were expressed as means ± SEM (n = 6 or n = 5). *p < 0.05, **p < 0.01, ***p < 0.001 vs. the Con group; #p < 0.05, ##p < 0.01, ###p < 0.001 vs. the IR group. | |
3.5 Phloretin combined with metformin promoted glucose consumption and suppressed gluconeogenesis in the liver via AKT/GLUT4 signaling pathways
To investigate the effect of phloretin and metformin on glucose consumption in the liver, the expression levels of P-AKT, AKT, PI3K, IRS-1, and GLUT4 in liver tissues were determined using western blotting. As shown in Fig. 6A, the protein levels of P-AKT, PI3K, IRS-1, and GLUT4 were significantly lower in the TD groups (P < 0.05) than in the Con group, and no changes in the total AKT protein level were observed (P > 0.05). However, administration of phloretin and metformin increased the expression of these proteins (P < 0.05). In addition, the upregulation effect in the CB group was the most obvious, in which the P-AKT, PI3K, IRS-1, and GLUT4 levels increased by 124.4%, 84.0%, 256.6%, and 205.8%, respectively.
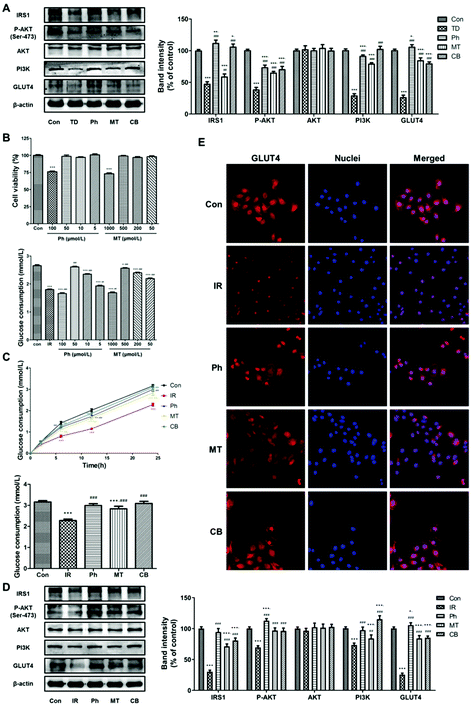 |
| Fig. 6 Phloretin combined with metformin promotes glucose consumption and suppresses gluconeogenesis in the liver via Akt/GLUT4 signaling pathways; (A) western blotting was used to analyze IRS-1, P-AKT, AKT, PI3K, and GLUT4 protein expression in the liver of the STZ induced T2D rats; (B) effect of different concentrations of phloretin and metformin on cytoactivity and glucose consumption in BRL-3A cells (n = 12); (C) evolution of glucose consumption in BRL-3A cells of each group and the glucose consumption of each group after 24 h of different treatments (n = 12); (D) western blotting was used to analyze IRS-1, P-AKT, AKT, PI3K, and GLUT4 protein expression in BRL-3A cells; (E) immunofluorescence staining of GLUT4 in BRL-3A cells (n = 5). Values were expressed as means ± SEM. *p < 0.05, **p < 0.01, ***p < 0.001 vs. the Con group; #p < 0.05, ##p < 0.01, ###p < 0.001 vs. the TD/IR group. | |
CCK8 assays were conducted to investigate whether phloretin and metformin exerted toxic effects on BRL-3A cells. Results suggested that 5 μmol L−1–50 μmol L−1 phloretin and 50 μmolL−1–500 μmol L−1 metformin were not cytotoxic in the experimental system and did not affect cell viability (P > 0.05, Fig. 6B). Next, glucose consumption in BRL-3A cells was assessed to determine the optimal concentrations of phloretin and metformin. Glucose consumption was obviously lower in the IR group than in the Con group. However, phloretin and metformin treatment increased the reduction of glucose consumption caused by PA in a dose-dependent manner, ranging from 5 μmol L−1–50 μmol L−1 and 50 μmol L−1–500 μmol L−1, respectively (P < 0.05, Fig. 6B). Based on the above results, we selected 50 μmol L−1 phloretin and 500 μmol L−1 metformin for further experiments. Both phloretin and metformin ameliorated the reduction in glucose consumption caused by PA in a time-dependent manner (P < 0.001), especially in the two-drug combination group (Fig. 6C). After 24 h of different treatments, results similar to that mentioned above were obtained, and glucose consumption in the CB group was almost similar to that in the Con group (P > 0.05, Fig. 6C). Furthermore, the results of western blotting of BRL-3A cells showed similar changes in protein expression (Fig. 6D). Phloretin combined with metformin significantly increased the expression of P-AKT, PI3K, IRS-1, and GLUT4 (P < 0.001).
Next, we used immunofluorescence staining to determine whether phloretin combined with metformin enhanced liver glucose consumption via GlUT4. As mentioned previously, BRL-3A cells were stained with Cy3 (red) for determining GLUT4 expression and the nuclei were stained with Hoechst 33
342 (blue). The GLUT4 expression in the IR group was lower than that in the Con group. However, phloretin and metformin treatments markedly decreased the GLUT4 expression, with the lowest expression observed in the combination group (Fig. 6E). Similar to that observed in L6 myotubes, phloretin promoted the translocation of GLUT4. As shown in Fig. 6E, GLUT4 was distributed more in the cell membrane in the Ph and CB groups, especially in the CB groups, which indicated improvement in glucose consumption.
4 Discussion
Metformin (dimethylbiguanide) was identified in 1959 as an agent that lowers blood glucose levels in individuals with T2D, which has been a boon for millions affected by this common metabolic disorder.32 Metformin is also recommended in combined treatment with sulfonylurea, an incretin, or insulin, irrespective of the number of insulin injections per day when monotherapy is no longer effective.8 Metformin functions to primarily reduce hepatic glucose production and decrease intestinal glucose absorption.33 Considering its current and potential therapeutic uses, metformin's mode of action might be thought to be well-understood. However, the precise molecular mechanism of action of metformin remains elusive.34 Currently, the most classical view regarding the mechanism via which metformin induces hypoglycemia is related to the activation of AMP-activated protein kinase (AMPK). Studies have shown that metformin-induced AMPK activation suppresses glucose production in the liver and also promotes glucose uptake in skeletal muscles.35 AMPK is activated via two separate routes: one via AMP
:
ATP ratio-stimulated phosphorylation by the upstream kinase LKB1, and another via calcium-induced phosphorylation by calcium/calmodulin-dependent kinase kinase 2 (CAMKK2). However, Carling also noted that “it wasn't clear how metformin was causing AMPK to be activated, what the molecular mechanisms were”.36
Based on previous experimental results, we observed that phloretin can improve glucose and lipid metabolism in STZ-induced T2D rats. Furthermore, phloretin can increase glucose uptake and glucose consumption in L6 myotubes via GLUT4, as well as the PI3K/AKT pathway. GLUT4 is part of a family of glucose transporter proteins containing 12-transmembrane domains,37 and is expressed primarily in skeletal muscles, adipose tissue, and cardiac tissues.38,39 In the absence of insulin, 90% GLUT4 remains intracellular, whereas in the presence of insulin, GLUT4 storage vesicles undergo exocytosis to the plasma membrane, as well as the sarcolemma and T-tubules of skeletal muscle cells, where it can perform functions related to glucose transport.40,41 These alterations increased glucose consumption and decreased the blood glucose level. Accumulating evidence indicates that either deregulation of expression or functional impairment of GLUT4 can cause insulin resistance. Owing to its crucial role, GLUT4 has been considered a potential therapeutic target for T2D. Insulin-regulated GLUT4 translocation can occur via lipid kinase phosphatidylinositol 3-kinase (PI3K). IRS-1 is the insulin receptor substrate protein and is mainly located in the hepatic and adipose tissue, which is sensitive to insulin.42 IRS-1 can associate with IR as the key intermediate in the PI3K/AKT signaling pathway.43 Blockade of IRS-1 phosphorylation can reduce IRS-1-associated PI3K and AKT activity. When the levels of P-AKT, the activated form of AKT, would be reduced, inhibition of PI3K/AKT might suppress the translocation and activity of GLUT4 and ultimately decrease insulin-stimulated glucose transport activity.44
In the present study, we used high-fat and high-sugar diet-fed and low-dose STZ-treated rats to model T2D. At the end of treatment, phloretin and metformin induced a significant reduction in the PBG level, and the highest improvement was observed in the CB group. The GHb level showed identical changes, which indicated that the combination of the two drugs can stabilize the blood glucose of T2D rats for a long time. Results of the OGTT assay showed that the blood glucose level in the CB group increased slowly and the blood glucose peak was low, which also indicated that the combination therapy of phloretin and metformin had beneficial effects on impaired glucose tolerance and maintained blood glucose homeostasis in T2D rats. An improvement in islet injury was observed in the CB group.
The liver plays a crucial role in metabolic homeostasis. It is the main place for the metabolism, synthesis, and redistribution of carbohydrates, glucose, and lipids, and for the development of insulin resistance.45 In the present study, we observed that the combination treatment significantly improved hyperlipidemia, as is evident from the changes in serum FFA, TG, TC, LDL, and HDL levels. Furthermore, the abnormalities in liver morphology and lipid accumulation were also attenuated, which contributed to the suppression of hepatic steatosis. As shown in Fig. 3, the improvement in lipid metabolism by the combined administration was significantly better than that by single administration, which indicated that the combination of phloretin and metformin had beneficial effects on the liver in T2D rats.
The target tissues of insulin are liver, skeletal muscle, and adipose tissue. The L6 cells were isolated from primary cultures of rat thigh muscles as they are able to maintain many morphological and metabolic characteristics of skeletal muscles.46 BRL-3A is a normal rat liver cell line and is a common cell model used for studying normal hepatocyte proliferation and oxidative damage. In this study, the L6 myotubes and BRL-3A cells were induced to insulin resistance using PA and the effect of glucose consumption was investigated. Our results showed that, compared to the TD/IR group, the expression levels of P-AKT, PI3K, IRS-1, and GLUT4 in the CB group were obviously high in both skeletal muscles and L6 myotubes. Meanwhile, phloretin combined with metformin promoted glucose consumption in L6 myotubes via stimulation of GLUT4 translocation and expression. In addition, insulin resistance is one of the characteristics of the low efficiency of glucose transportation and utilization on the cell membrane of liver tissue.47 Increased hepatic glucose production is one of the reasons for insulin resistance in T2D.45 Our results showed that the combination treatment upregulated the expression of GLUT4 and other target proteins in the liver tissue of T2D rats, contributing to an increase in glucose consumption. Glucose uptake in liver tissue is compromised in patients with T2D, leading to elevated levels of hepatic glucose and metabolic disorder.48 Hence, we also investigated the effect of phloretin and metformin on glucose uptake and consumption in PA-induced BRL-3A cells. Our results showed that the glucose consumption in the CB group is almost similar to that in the Con group, and it considerably exceeded that of the Ph and MT groups. The translocation and expression of GLUT4 were obviously improved in the CB group, which indicated that phloretin combined with metformin promoted glucose consumption via activation of the GLUT4 signaling pathway.
5. Conclusions
Considering that currently, combination therapy with naturally occurring antidiabetic agents has been shown to exert beneficial effects on diabetic disturbances, it might be an interesting strategy to use this approach for the treatment of T2D. The present study showed the additive effects of phloretin combined with metformin on improving glucose and lipid metabolism in T2D rats. This study provides the first evidence regarding a promising therapeutic strategy based on a combination of phloretin and metformin for the improvement of glucose consumption and glucose tolerance, at least partially, via stimulation of GLUT4 translocation and expression. Further studies are required to clarify whether this combination therapy also prevents diabetes in other animal models or humans, and whether it affects other metabolism-related signaling pathways.
Conflicts of interest
There are no conflicts to declare.
Acknowledgements
This work was financially supported by the Shaanxi Key Research and Development Program (2017KW-047).
References
- C. D. Mathers and D. Loncar, Projections of global mortality and burden of disease from 2002 to 2030, PLoS Med., 2006, 3, e442 CrossRef PubMed.
- K. Ogurtsova, J. D. da Rocha Fernandes, Y. Huang, U. Linnenkamp, L. Guariguata, N. H. Cho, D. Cavan, J. E. Shaw and L. E. Makaroff, IDF Diabetes Atlas: Global estimates for the prevalence of diabetes for 2015 and 2040, Diabetes Res. Clin. Pract., 2017, 128, 40–50 CrossRef CAS PubMed.
- L. Xiang, W. S. Cheang, S. H. Lin, L. Wang, Y. L. Li, Y. Huang and Z. W. Cai, Plasma metabolic signatures reveal the regulatory effect of exercise training in db/db mice, Mol. BioSyst., 2015, 11, 2588–2596 RSC.
- D. R. Whiting, L. Guariguata, C. Weil and J. Shaw, IDF diabetes atlas: global estimates of the prevalence of diabetes for 2011 and 2030, Diabetes Res. Clin. Pract., 2011, 94, 311–321 CrossRef PubMed.
- S. E. Inzucchi, R. M. Bergenstal, J. B. Buse, M. Diamant, E. Ferrannini, M. Nauck, A. L. Peters, A. Tsapas, R. Wender and D. R. Matthews, Management of hyperglycaemia in type 2 diabetes: a patient-centered approach. Position statement of the American Diabetes Association (ADA) and the European Association for the Study of Diabetes (EASD), Diabetologia, 2012, 55, 1577–1596 CrossRef CAS PubMed.
- A. J. Scheen and C. Mathieu, Management of hyperglycaemia in type 2 diabetes: a patient-centered approach, Rev. Med. Liege, 2012, 67, 623–631 CAS.
- M. Stumvoll, N. Nurjhan, G. Perriello, G. Dailey and J. E. Gerich, Metabolic effects of metformin in non-insulin-dependent diabetes mellitus, N. Engl. J. Med., 1995, 333, 550–554 CrossRef CAS PubMed.
- M. P. Wrobel, B. Marek, D. Kajdaniuk, D. Rokicka, A. Szymborska-Kajanek and K. Strojek, Metformin - a new old drug, Endokrynol. Pol., 2017, 68, 482–496 CrossRef CAS PubMed.
- R. S. Hundal, M. Krssak, S. Dufour, D. Laurent, V. Lebon, V. Chandramouli, S. E. Inzucchi, W. C. Schumann, K. F. Petersen, B. R. Landau and G. I. Shulman, Mechanism by which metformin reduces glucose production in type 2 diabetes, Diabetes, 2000, 49, 2063–2069 CrossRef CAS PubMed.
- N. Musi, M. F. Hirshman, J. Nygren, M. Svanfeldt, P. Bavenholm, O. Rooyackers, G. Zhou, J. M. Williamson, O. Ljunqvist, S. Efendic, D. E. Moller, A. Thorell and L. J. Goodyear, Metformin increases AMP-activated protein kinase activity in skeletal muscle of subjects with type 2 diabetes, Diabetes, 2002, 51, 2074–2081 CrossRef CAS PubMed.
- K. W. Lee, Y. J. Kim, D. O. Kim, H. J. Lee and C. Y. Lee, Major phenolics in apple and their contribution to the total antioxidant capacity, J. Agric. Food Chem., 2003, 51, 6516–6520 CrossRef CAS PubMed.
- P. Hilt, A. Schieber, C. Yildirim, G. Arnold, I. Klaiber, J. Conrad, U. Beifuss and R. Carle, Detection of phloridzin in strawberries (Fragaria x ananassa Duch.) by HPLC-PDA-MS/MS and NMR spectroscopy, J. Agric. Food Chem., 2003, 51, 2896–2899 CrossRef CAS PubMed.
- S. T. Lin, S. H. Tu, P. S. Yang, S. P. Hsu, W. H. Lee, C. T. Ho, C. H. Wu, Y. H. Lai, M. Y. Chen and L. C. Chen, Apple Polyphenol Phloretin Inhibits Colorectal Cancer Cell Growth via Inhibition of the Type 2 Glucose Transporter and Activation of p53-Mediated Signaling, J. Agric. Food Chem., 2016, 64, 6826–6837 CrossRef CAS PubMed.
- D. Ren, Y. Liu, Y. Zhao and X. Yang, Hepatotoxicity and endothelial dysfunction induced by high choline diet and the protective effects of phloretin in mice, Food Chem. Toxicol., 2016, 94, 203–212 CrossRef CAS PubMed.
- G. Shu, N. S. Lu, X. T. Zhu, Y. Xu, M. Q. Du, Q. P. Xie, C. J. Zhu, Q. Xu, S. B. Wang, L. N. Wang, P. Gao, Q. Y. Xi, Y. L. Zhang and Q. Y. Jiang, Phloretin promotes adipocyte differentiation in vitro and improves glucose homeostasis in vivo, J. Nutr. Biochem., 2014, 25, 1296–1308 CrossRef CAS PubMed.
- H. P. Vasantha Rupasinghe and A. Yasmin, Inhibition of oxidation of aqueous emulsions of omega-3 fatty acids and fish oil by phloretin and phloridzin, Molecules, 2010, 15, 251–257 CrossRef CAS PubMed.
- X. Shen, N. Zhou, L. Mi, Z. Hu, L. Wang, X. Liu and S. Zhang, Phloretin exerts hypoglycemic effect in streptozotocin-induced diabetic rats and improves insulin resistance in vitro, Drug Des., Dev. Ther., 2017, 11, 313–324 CrossRef CAS PubMed.
- L. Peng, J. Li, Y. Xu, Y. Wang, H. Du, J. Shao and Z. Liu, The Protective Effect of Beraprost Sodium on Diabetic Nephropathy by Inhibiting Inflammation and p38 MAPK Signaling Pathway in High-Fat Diet/Streptozotocin-Induced Diabetic Rats, Int. J. Endocrinol., 2016, 2016, 1690474 Search PubMed.
- Y. F. Gao, M. N. Zhang, T. X. Wang, T. C. Wu, R. D. Ai and Z. S. Zhang, Hypoglycemic effect of D-chiro-inositol in type 2 diabetes mellitus rats through the PI3K/Akt signaling pathway, Mol. Cell. Endocrinol., 2016, 433, 26–34 CrossRef CAS PubMed.
- Z. K. Salman, R. Refaat, E. Selima, A. El Sarha and M. A. Ismail, The combined effect of metformin and L-cysteine on inflammation, oxidative stress and insulin resistance in streptozotocin-induced type 2 diabetes in rats, Eur. J. Pharmacol., 2013, 714, 448–455 CrossRef CAS PubMed.
- F. Zhang, C. Ye, G. Li, W. Ding, W. Zhou, H. Zhu, G. Chen, T. Luo, M. Guang, Y. Liu, D. Zhang, S. Zheng, J. Yang, Y. Gu, X. Xie and M. Luo, The rat model of type 2 diabetic mellitus and its glycometabolism characters, Exp. Anim., 2003, 52, 401–407 CrossRef CAS PubMed.
- K. Srinivasan, B. Viswanad, L. Asrat, C. L. Kaul and P. Ramarao, Combination of high-fat diet-fed and low-dose streptozotocin-treated rat: a model for type 2 diabetes and pharmacological screening, Pharmacol. Res., 2005, 52, 313–320 CrossRef CAS PubMed.
- D. Wu, W. Wen, C. L. Qi, R. X. Zhao, J. H. Lu, C. Y. Zhong and Y. Y. Chen, Ameliorative effect of berberine on renal damage in rats with diabetes induced by high-fat diet and streptozotocin, Phytomedicine, 2012, 19, 712–718 CrossRef CAS PubMed.
- C. Ju, W. Yue, Z. Yang, Q. Zhang, X. Yang, Z. Liu and F. Zhang, Antidiabetic effect and mechanism of chitooligosaccharides, Biol. Pharm. Bull., 2010, 33, 1511–1516 CrossRef CAS PubMed.
- S. Jin, C. Chang, L. Zhang, Y. Liu, X. Huang and Z. Chen, Chlorogenic acid improves late diabetes through adiponectin receptor signaling pathways in db/db mice, PLoS One, 2015, 10, e0120842 CrossRef PubMed.
- D. R. Matthews, J. P. Hosker, A. S. Rudenski, B. A. Naylor, D. F. Treacher and R. C. Turner, Homeostasis model assessment: insulin resistance and beta-cell function from fasting plasma glucose and insulin concentrations in man, Diabetologia, 1985, 28, 412–419 CrossRef CAS PubMed.
- L. Fu, A. Bruckbauer, F. Li, Q. Cao, X. Cui, R. Wu, H. Shi, M. B. Zemel and B. Xue, Leucine amplifies the effects of metformin on insulin sensitivity and glycemic control in diet-induced obese mice, Metabolism, 2015, 64, 845–856 CrossRef CAS PubMed.
- T. D. Allerton and S. D. Primeaux, QRFP-26 enhances insulin's effects on glucose uptake in rat skeletal muscle cells, Peptides, 2015, 69, 77–79 CrossRef CAS PubMed.
- S. E. Park, M. H. Cho, J. K. Lim, J. S. Kim, J. H. Kim, D. Y. Kwon and C. S. Park, A new colorimetric method for determining the isomerization activity of sucrose isomerase, Biosci., Biotechnol., Biochem., 2007, 71, 583–586 CrossRef CAS PubMed.
- K. Fang, H. Dong, S. Jiang, F. Li, D. Wang, D. Yang, J. Gong, W. Huang and F. Lu, Diosgenin and 5-Methoxypsoralen Ameliorate Insulin Resistance through ER-alpha/PI3K/Akt-Signaling Pathways in HepG2 Cells, Evid. Based Complement. Alternat. Med., 2016, 2016, 7493694 Search PubMed.
- J. Hao, C. Chen, K. Huang, J. Huang, J. Li, P. Liu and H. Huang, Polydatin improves glucose and lipid metabolism in experimental diabetes through activating the Akt signaling pathway, Eur. J. Pharmacol., 2014, 745, 152–165 CrossRef CAS PubMed.
- J. Sterne, Treatment of diabetes mellitus with N,N-dimethylguanylguanidine (LA. 6023, glucophage), Therapie, 1959, 14, 625–630 CAS.
- I. Pernicova and M. Korbonits, Metformin–mode of action and clinical implications for diabetes and cancer, Nat. Rev. Endocrinol., 2014, 10, 143–156 CrossRef CAS PubMed.
- M. J. Spiering, The mystery of metformin, J. Biol. Chem., 2019, 294, 6689–6691 CrossRef CAS PubMed.
- G. Zhou, R. Myers, Y. Li, Y. Chen, X. Shen, J. Fenyk-Melody, M. Wu, J. Ventre, T. Doebber, N. Fujii, N. Musi, M. F. Hirshman, L. J. Goodyear and D. E. Moller, Role of AMP-activated protein kinase in mechanism of metformin action, J. Clin. Invest., 2001, 108, 1167–1174 CrossRef CAS PubMed.
- L. G. Fryer, A. Parbu-Patel and D. Carling, The Anti-diabetic drugs rosiglitazone and metformin stimulate AMP-activated protein kinase through distinct signaling pathways, J. Biol. Chem., 2002, 277, 25226–25232 CrossRef CAS PubMed.
-
E. Vargas, V. Podder and M. A. Carrillo Sepulveda, in StatPearls, Treasure Island (FL), 2019 Search PubMed.
- A. C. Poletto, A. David-Silva, A. P. Yamamoto, U. F. Machado and D. T. Furuya, Reduced Slc2a4/GLUT4 expression in subcutaneous adipose tissue of monosodium glutamate obese mice is recovered after atorvastatin treatment, Diabetol. Metab. Syndr., 2015, 7, 18 CrossRef PubMed.
- P. Fang, M. Shi, L. Guo, B. He, Q. Wang, M. Yu, P. Bo and Z. Zhang, Effect of endogenous galanin on glucose transporter 4 expression in cardiac muscle of type 2 diabetic rats, Peptides, 2014, 62, 159–163 CrossRef CAS PubMed.
- N. J. Bryant, R. Govers and D. E. James, Regulated transport of the glucose transporter GLUT4, Nat. Rev. Mol. Cell Biol., 2002, 3, 267–277 CrossRef CAS PubMed.
- R. T. Watson, M. Kanzaki and J. E. Pessin, Regulated membrane trafficking of the insulin-responsive glucose transporter 4 in adipocytes, Endocr. Rev., 2004, 25, 177–204 CrossRef CAS PubMed.
- K. Morino, K. F. Petersen, S. Dufour, D. Befroy, J. Frattini, N. Shatzkes, S. Neschen, M. F. White, S. Bilz, S. Sono, M. Pypaert and G. I. Shulman, Reduced mitochondrial density and increased IRS-1 serine phosphorylation in muscle of insulin-resistant offspring of type 2 diabetic parents, J. Clin. Invest., 2005, 115, 3587–3593 CrossRef CAS PubMed.
- Y. Zhu, R. O. Pereira, B. T. O'Neill, C. Riehle, O. Ilkun, A. R. Wende, T. A. Rawlings, Y. C. Zhang, Q. Zhang, A. Klip, I. Shiojima, K. Walsh and E. D. Abel, Cardiac PI3K-Akt impairs insulin-stimulated glucose uptake independent of mTORC1 and GLUT4 translocation, Mol. Endocrinol., 2013, 27, 172–184 CrossRef CAS PubMed.
- Z. Jiang, B. Yu and Y. Li, Effect of Three Statins on Glucose Uptake of Cardiomyocytes and its Mechanism, Med. Sci. Monit., 2016, 22, 2825–2830 CrossRef CAS PubMed.
- Y. Liu, J. Deng and D. Fan, Ginsenoside Rk3 ameliorates high-fat-diet/streptozocin induced type 2 diabetes mellitus in mice via the AMPK/Akt signaling pathway, Food Funct., 2019, 10, 2538–2551 RSC.
- D. Yaffe, Retention of differentiation potentialities during prolonged cultivation of myogenic cells, Proc. Natl. Acad. Sci. U. S. A., 1968, 61, 477–483 CrossRef CAS PubMed.
- A. Dihingia, D. Ozah, S. Ghosh, A. Sarkar, P. K. Baruah, J. Kalita, P. C. Sil and P. Manna, Vitamin K1 inversely correlates with glycemia and insulin resistance in patients with type 2 diabetes (T2D) and positively regulates SIRT1/AMPK pathway of glucose metabolism in liver of T2D mice and hepatocytes cultured in high glucose, J. Nutr. Biochem., 2018, 52, 103–114 CrossRef CAS PubMed.
- S. Herzig and R. J. Shaw, AMPK: guardian of metabolism and mitochondrial homeostasis, Nat. Rev. Mol. Cell Biol., 2018, 19, 121–135 CrossRef CAS PubMed.
Footnote |
† These authors contributed equally to this work. |
|
This journal is © The Royal Society of Chemistry 2020 |