DOI:
10.1039/C8FO02467G
(Paper)
Food Funct., 2019,
10, 3312-3323
Collagen peptide-loaded W1/O single emulsions and W1/O/W2 double emulsions: influence of collagen peptide and salt concentration, dispersed phase fraction and type of hydrophilic emulsifier on droplet stability and encapsulation efficiency†
Received
13th December 2018
, Accepted 25th April 2019
First published on 26th April 2019
Abstract
Bioactive peptides hold great promise as functional ingredients, but they are susceptible to hydrolysis (e.g., protease degradation) during digestion and under different physiological conditions in the human body. Thus, bioactive peptides must be encapsulated first to preserve their innate characteristics and to ensure delivery to an absorption site at the small intestine without biodegradation. The objective of this study was to formulate stable single emulsions of water in oil (W1/O) and double emulsions of water in oil in water (W1/O/W2) containing collagen peptide as core material. In this study, the influence of the following parameters was investigated: collagen peptide concentration (0–20%), salt (0% or 1% NaCl), hydrophilic emulsifiers for the outer water phase (Tween 80, lecithin, chitosan, pectin), and the use of different inner/outer water fractions (20–50% inner water, 50–80% outer water) on the droplet stability of the W1/O and W1/O/W2 emulsions during storage. In particular, the influence of the osmolytes was investigated to evaluate the change in osmotic balance and destabilization of the W1/O/W2 emulsion system as the encapsulated peptide was also osmotically active. Tween 80-stabilized W1/O/W2 emulsions showed the best droplet stability without phase separation (d90,3 = 36.6 μm) and the highest collagen peptide retention (encapsulation efficiency > 90%). During prolonged storage, collagen peptides were released from the W1/O/W2 emulsion system without significant droplet size changes, possibly due to diffusion of the collagen peptide from the inner to the outer water phase. Therefore, our findings can be utilized for preparing stable functional foods or cosmetic products from W1/O/W2 emulsions containing bioactive ingredients.
1. Introduction
Bioactive peptides are protein hydrolysates and hold great promise as valuable functional ingredients for humans. These peptides range in size from 2 to 50 amino acids and have molecular masses of less than 6000 Da. Their structural properties can induce beneficial biological activities, such as antihypertensive, antioxidative, immunomodulatory, anticancer, antimicrobial, and blood lipid-lowering effects.1,2 Some bioactive peptides are also multifunctional and produce more than one effect. The production and functionality of protein-derived bioactive peptides have been studied continuously in the food and cosmetic fields. Recently, the study of antioxidant peptides from foods, plants, and animals has become a very active research area and has received increasing attention in the pharmaceutical, alimentary health, and processing/preservation industries.3,4 In food systems, these food-derived antioxidants are considered to be safer and free from the side effects associated with synthetic antioxidants.4 Moreover, most studies on bioactive peptides have focused on the discovery of new bioactivities and protein precursors and the elucidation of their mechanisms, while limited attention has been given to their biostability and bioavailability.2,3 For examples, collagen peptide can be easily used in diverse fields including the food, cosmetics, and biomedical industries because of their bioactivities, such as antihypertensive and antioxidative activities.1,5 However, these peptides are susceptible to hydrolysis (e.g., protease degradation) under various physiological conditions encountered in the human body. The susceptibility of bioactive peptides to protease degradation is a major drawback, which can shorten the in vivo half-life of the peptide drug and may also lead to undesirable pharmacological reactions and potentially toxic end-products.6 Therefore, bioactive peptides must be encapsulated in order to retain their full functionality. Encapsulation techniques can protect the bioactive peptides in the stomach and allow their delivery to an absorption site within the small intestine without biodegradation.6 Moreover, they can preserve the innate characteristics of active substances from external environment (e.g., high temperatures, exposure to light, changes in pH, high levels of oxygen, and moisture content).
Until now, most studies on the encapsulation of active materials have focused on the protection of lipophilic food compounds such as carotene, fish oil, and essential oils from lipid oxidation.7–9 Many attempts have been made to encapsulate various lipophilic compounds via strategies such as molecular inclusion, emulsion systems, polymerization, microcapsules, and micelles in the food and pharmaceutical sector.8,10–12 In contrast, delivery systems for hydrophilic compounds have rarely been investigated in the context of food applications. Furthermore, most encapsulation techniques for hydrophilic compounds use liposomes as delivery systems. Mosquera et al. (2014)13 reported that the encapsulation of collagen peptide from sea bream scales in nanoliposomes could preserve the bioactivity of the peptide during storage. They also reported that the collagen peptide-loaded liposomes represented an alternative to improve the stability of these compounds when applied to a food product. However, the liposome formulation is more susceptible to leakage and fusion of the encapsulated materials during preparation, processing, storage, and gastrointestinal tract (GIT) digestion compared to other encapsulation systems (e.g., emulsions, nano-capsules or spheres, solid lipid particles).14 Normally, the encapsulation efficiency of active compounds using conventional liposome formulation was around 40–50%, whereas double emulsion system for active compounds has 80–90% encapsulation efficiency.5 Therefore, in this study, water-in-oil-in-water double emulsions are explored as an alternative encapsulation technique for the delivery of water-soluble compounds (e.g., bioactive peptides) to enhance their utilization for human health promotion.
Double emulsions, such as water-in-oil-in-water (W1/O/W2) emulsions, have already been successfully used for the encapsulation of hydrophilic ingredients15,16 and are therefore a possible carrier system for bioactive peptide encapsulation. These systems are multicompartment liquid dispersions in which the dispersed phase is itself an emulsion. They are also called ‘emulsions of emulsions’. W1/O/W2 emulsions are generally formulated by means of a two-step process.17 A water-in-oil (W1/O) emulsion, or so-called ‘inner’ emulsion, is prepared during the initial emulsification step, and then dispersed into a continuous water phase (W2). This type of system can overcome the weaknesses of liposomes that were discussed above. Therefore, the present work aims to encapsulate collagen peptides using a W1/O/W2 double emulsion system to enhance their chemical stability. The focus of the study is on the influence of the formulation parameters (inner/outer water fractions, collagen peptide concentration, hydrophilic emulsifiers for the outer water phase, etc.) on the emulsion droplet stability (inner and outer droplet size), the encapsulation efficiency of collagen peptide, and related formulation properties (such as viscosity).
2. Materials and methods
2.1 Materials
Collagen peptide (CP, hydrolyzed fish collagen, Naticol®4000 MG, molecular weight < 3000 Da) was obtained from Weishardt International Group (Graulhet, France). Canola oil was provided by B. Schell GmbH (Baden, Germany) for use as the oil phase of the W1/O emulsion. Polyglycerol polyricinoleate (PGPR 4150) was provided by Palsgaard® (Juelsminde, Denmark) for use as an oil-soluble emulsifier. Sodium chloride (NaCl) with a purity >99.5% was purchased from Carl Roth (Karlsruhe, Germany). For hydrophilic emulsifiers, Tween 80 and lecithin (Lipoid S75: a mixture of soybean phospholipids, triglycerides and fatty acids), which were used as small molecule emulsifiers, were purchased from Carl Roth (Karlsruhe, Germany) and Lipoid GmbH (Ludwigshafen, Germany), respectively. Chitosan (from shrimp shells, ≥75% (deacetylated) and pectin (from citrus peel, galacturonic acid ≥ 74.0%, dried basis), which were used as large molecule emulsifiers, were purchased from Sigma-Aldrich (St Louis, USA).
2.2 Preparation of the CP-loaded W1/O single emulsions
The CP-loaded W1/O single emulsions (inner emulsions) were prepared using an internal aqueous phase (W1) and a continuous oily phase (O). CP (CCP = 0, 5, 10, or 20%, w/w) was dissolved in demineralized water or in a 1% (w/w) NaCl solution. NaCl, which is an osmolyte, was used in order to suppress Ostwald ripening in the double emulsion by balancing the Laplace pressure by increasing the osmotic pressure of the inner water phase.18–20 The continuous oily phase consisted of canola oil containing 5% (w/w) PGPR. At this concentration, the total coverage of the interface for the highest concentrations of the dispersed phase water droplets was ensured. The W1/O emulsions were produced with different internal aqueous phase content ΦW1 (inner water fraction) = 20, 30, 40, or 50% (w/w). The internal aqueous phase was slowly added to the continuous oily phase and stirred with a propeller stirrer until a homogeneous emulsion premix was obtained (x50,3 ≈ 10 μm). Approximately 300 g of this premix was then further homogenized using an IKA® magicLAB® lab scale rotor-stator device (a toothed rim dispersing machine, module UTL, IKA Stauffen, Germany) at 20
000 rpm, operating in the semi-continuous mode for 5 min. All emulsions were stored in a 50 mL glass bottle for 90 days at room temperature (Table 1).
Table 1 Composition of W1/O emulsions (inner single emulsions) and W1/O/W2 emulsions (whole double emulsions) formulated within this study; CCP = concentration of collagen peptide (CP, %, w/w) in the inner water phase, CN = concentration of NaCl (%, w/w) in the inner water phase, ΦW1 = water fraction of the inner W1/O emulsion, ΦO = oil fraction of the inner W1/O emulsion, ΦW2 = water fraction of the outer W1/O/W2 emulsion. All fractions are expressed on a weight basis
No. |
Emulsion type |
Water in oil in water (W1/O/W2) double emulsions |
Water in oil (W1/O) single emulsions |
|
|
Internal aqueous phase |
Continuous oily phase |
External aqueous phase |
C
CP
|
C
N
|
Φ
W1
|
Φ
O
|
Composition |
Φ
W2
|
Composition |
1 |
Single |
0% |
1% |
20% |
80% |
Canola (95%) + PGPR (5%) |
— |
— |
2 |
Single |
0% |
1% |
30% |
70% |
— |
— |
3 |
Single, double |
0% |
1% |
40% |
60% |
50% |
Tween 80 (1%) |
4 |
Single |
0% |
1% |
50% |
50% |
— |
— |
5 |
Single, double |
0% |
0% |
40% |
60% |
50% |
Tween 80 (1%) |
6 |
Single, double |
5% |
0% |
40% |
60% |
50% |
Tween 80 (1%) |
7 |
Single, double |
10% |
0% |
40% |
60% |
50% |
Tween 80 (1%) |
8 |
Single, double |
20% |
0% |
40% |
60% |
50% |
Tween 80 (1%) |
9 |
Single, double |
5% |
1% |
40% |
60% |
50% |
Tween 80 (1%) |
10 |
Single, double |
10% |
1% |
40% |
60% |
50% |
Tween 80 (1%) |
11 |
Single, double |
20% |
1% |
40% |
60% |
50% |
Tween 80 (1%) |
12 |
Double |
10% |
1% |
40% |
60% |
60% |
Tween 80 (1%) |
13 |
Double |
10% |
1% |
40% |
60% |
70% |
Tween 80 (1%) |
14 |
Double |
10% |
1% |
40% |
60% |
80% |
Tween 80 (1%) |
15 |
Double |
10% |
1% |
40% |
60% |
50% |
Lecithin (1%) |
16 |
Double |
10% |
1% |
40% |
60% |
50% |
Chitosan (1%) |
17 |
Double |
10% |
1% |
40% |
60% |
50% |
Pectin (1%) |
2.3 Preparation of the CP-loaded W1/O/W2 double emulsions
CP-loaded W1/O/W2 double emulsions were prepared by blending the W1/O single emulsions with an external aqueous phase containing a hydrophilic emulsifier (Tween 80, lecithin, chitosan, or pectin) that had been previously dissolved by stirring overnight. All hydrophilic emulsifiers were solubilized in distilled water at 1% concentration. The W1/O/W2 emulsions were produced with different external aqueous phase content ΦW2 (outer water fraction) = 50, 60, 70, or 80% (w/w). The W1/O emulsion was slowly added to the external aqueous phase and stirred with a propeller stirrer until a homogeneous emulsion premix was obtained. Approximately 200 g of this premix was then further homogenized using an IKA® magicLAB® lab scale rotor-stator device (a colloid mill, module MK, IKA Stauffen, Germany). The gap width was set to 0.64 mm, and a rotation speed of 15
000 rpm was applied for 1 min in a semicontinuous process. All emulsions were stored in a 50 mL glass bottle for 90 days at room temperature.
2.4 Interfacial tension measurement using the pendant drop technique
The interfacial tension between the internal aqueous phase and the canola oil (pure oil or 5% w/w PGPR oil) was measured at various collagen peptide concentrations using a PAT-1 drop shape tensiometer (Sinterface Technologies, Germany). The measurements were carried out as a function of time to establish the equilibrium time required to obtain a constant interfacial tension at 25 °C. Each measurement was repeated three times.
2.5 Emulsion droplet size distribution
The droplet size distributions of W1/O emulsions were characterized using a laser diffraction particle analyzer (Horiba LA-950, Retsch Technology GmbH, Haan, Germany). The refractive index of the continuous phase was set to 1.473 and that of the dispersed water phase to 1.333; the imaginary part of the refractive index was set to zero. The dispersing medium for the emulsions was oil with 5% (w/w) PGPR (i.e., the same continuous oil phase used for emulsion production).
The droplet size distributions of the W1/O/W2 emulsions were characterized using a laser diffraction particle analyzer (LS13
320, Beckman Coulter GmbH, Krefeld, Germany). The W1/O/W2 double emulsion sample was added dropwise to the circulation chamber, which was initially filled with demineralized water. The refractive index of the continuous phase was set to 1.333.
All measurements were performed three times. The 90th percentile of the cumulative volumetric droplet size distribution, i.e., the maximum droplet size d90,3, was used as the characteristic value to describe the droplet size distribution. This can be used as a measure of the stability of an emulsion against creaming.
The relative span of the droplet size distribution was used as the indicator of the degree of droplet uniformity, as described by eqn (1):
| Span value = (d90,3 − d10,3)/d50,3 | (1) |
where
d10,3,
d50,3, and
d90,3 are the equivalent volume diameters at 10, 50, and 90% of the cumulative distribution.
2.6 Emulsion viscosity
The flow behavior of the emulsions was analyzed in a rotational rheometer with a plate-and-plate system (Physics MCR 301; geometry: PP-50, Anton Paar, Graz, Austria). A shear experiment was conducted with shear rates between 0.01 and 1000 s−1. The emulsion viscosity was derived from the flow curve. All analyses were conducted at a controlled temperature of 25 °C in triplicate.
2.7 Encapsulation efficiency
The encapsulation efficiency (EE, %) of CP was determined using UV/VIS spectrophotometry. To extract free CP from the W1/O/W2 emulsions, the emulsions (6 g) were diluted with demineralized water (6 g) in a 50 mL conical tube and then gently hand-shaken. The diluted emulsions were centrifuged at 7619g and 4 °C for 90 min using a Centrifuge 5430 R (Eppendorf AG, Germany) to separate them into 2 layers: the creamed layer (upper) and the serum layer (bottom). The serum layer from each centrifuged sample was collected using a syringe needle (0.80 × 120 mm) and filtered using a 0.22 μm syringe (33 mm, Rotilabo®-syringe filters, CME, Carl Roth GmbH + Co. KG, Germany) to remove oil droplets. The filtrate was used to detect the free CP. The EE was evaluated by determining the amount of CP in each of the collected filtrates by the BCA protein assay (Roti®-Quant Universal, Carl Roth GmbH + Co. KG, Germany). The EE was indirectly calculated using a calibration curve constructed from a series of CP solutions with standard concentrations. The EE was then obtained as a percentage using eqn (2): | EE (%) = (1 − A/A0) × 100 | (2) |
where A0 is the amount of CP added to the internal aqueous phase, and A is the amount of free CP extracted from the external aqueous phase.
2.8 Microscopic observation
Images of the W1/O/W2 emulsion droplets were taken using an Eclipse LV100ND (Nikon GmbH, Düsseldorf, Germany) equipped with a DS-Fi1c camera (Nikon GmbH, Düsseldorf, Germany) connected to the relevant software. A single droplet of a diluted (1
:
10) emulsion was applied to a microscope slide and covered with a cover glass. The images were recorded at 20, 50 and 100-fold magnification.
2.9 Statistical analysis
Statistical analysis was performed using the OriginPro 9.1G software (OriginLab Corp., Northampton, MA, USA). The results of the triplicate analyses were used to calculate the averages and standard deviations. The data were analyzed by one-way analysis of variance. Significant differences between the mean values of variables (p < 0.05) were determined using Scheffe's method.
3. Results and discussion
3.1 Characterization of the W1/O single emulsions
3.1.1 Influence of inner water fraction on the W1/O emulsions.
To produce a stable double emulsion, the stability of the single W1/O emulsion must first be ensured. The emulsion stability was characterized by measuring its droplet size distribution for a given time. By varying the emulsification conditions and the type and amount of emulsifier, the presence of salts, and the ratio of the dispersed and continuous phases, different droplet size distributions can be produced.21,22 In this study, several W1/O emulsions were prepared with different inner water fractions (ΦW1 = 20, 30, 40, or 50%) containing 1% w/w NaCl in the W1-phase. The maximum droplet size (d90,3) and span value of the volume distribution of the droplet sizes were determined at the time of fabrication (day 0) and after 90 days of storage (Fig. 1A). On day 0, the d90,3 of the W1/O emulsion significantly increased, along with an increase in ΦW1, from 0.467 to 0.603 μm. Even after 90 days of storage, no phase separation was observed for any of the emulsions (data not shown). The lack of separation was attributed to the nanosized small droplets (<1 μm) and the high viscosity of the continuous oil phase. The span values of the W1/O emulsions increased along with increase in ΦW1. The span values of all W1/O emulsions increased after 90 days of storage. During storage, the maximum droplet sizes d90,3 of all of the W1/O emulsions decreased, which may be attributed to of the possibility of the shrinking behaviors of water droplets by the solubility of water in the oil phase. Shrinking is, however, more difficult to prove. The mechanism of this effect will be investigated in more detail in future research.
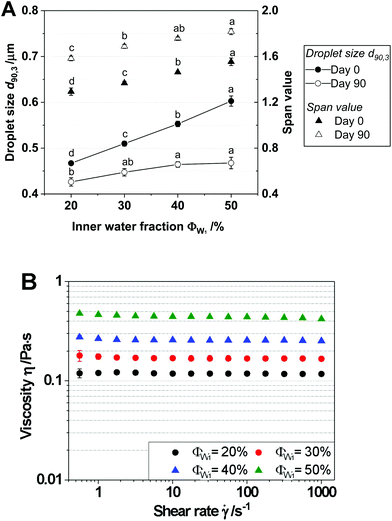 |
| Fig. 1 Characteristics of blank-W1/O single emulsions with different water fractions in the inner W1/O emulsion (ΦW1): (A) droplet size and span value; (B) viscosity. All emulsions were prepared with 1% NaCl and without collagen peptide. Percent is expressed on a weight basis. Data labeled with different letters (a–d) show statistically significant differences (p < 0.05). | |
Fig. 1B shows the viscosities of the W1/O emulsions as a function of the inner water fractions ΦW1 for those emulsions containing 1% w/w NaCl in the W1-phase. All of the W1/O emulsions exhibited Newtonian behavior (n ∼ 1), which indicates that their apparent shear viscosity characteristics were independent of the applied shear rate. However, the viscosity of the W1/O emulsions increased as ΦW1 increased. The observed trend was similar to the results of Schuch, et al. (2013)17 for W1/O emulsions and is typical for dispersion.23 According to Farah et al. (2005),24 the effective viscosity of W1/O emulsions depends mainly on the volume fraction of the dispersed phase, along with several other minor effects such as the shear rate, droplet size and distribution, and the viscosity. The correlation between the inner water fraction ΦW1 and the viscosity affected the droplet size of the W1/O emulsions: high emulsion viscosities can induce viscosity ratios between the dispersed phase and surrounding phase that are unfavorable for effective droplet breakup, ultimately leading to larger droplet sizes.25
3.1.2 Influence of the collagen peptide concentration.
The W1/O emulsions were stabilized mainly by steric forces, due to the low electrical conductivity of the oily continuous phase. We hypothesized that the collagen peptide used in this study might also change the osmotic pressure of the inner water phase, thereby producing an imbalance in the system leading to emulsion destabilization. Furthermore, proteins (but also peptides) are well-known to show interfacial activity. In the system under investigation, the expected interfacial activity of CP might interfere with PGPR at the water/oil interface, leading again to destabilized emulsions. Thus, CP-loaded W1/O emulsions were prepared with different concentrations of CP in the presence of 1% w/w NaCl or without NaCl, and their d90,3 and viscosity were measured (Fig. 2). As shown in Fig. 2A, the W1/O emulsions with NaCl (d90,3 range from 0.553 μm to 0.691 μm) had smaller d90,3 values than those without NaCl (d90,3 range from 1.187 μm to 2.976 μm). In the W1/O emulsions containing NaCl, increasing the concentration of CP resulted in a slight increase in the d90,3 of the W1/O emulsions. On the other hand, in the absence of NaCl, the d90,3 of the W1/O emulsions decreased significantly with increase in CP concentration. In particular, a low CP concentration (5% CP) led to an increased droplet size for the W1/O emulsions in the absence of NaCl, whereas a high CP concentration (20% CP) resulted in a d90,3 value similar to the W1/O emulsion (∼1.2 μm) without CP (0% CP).
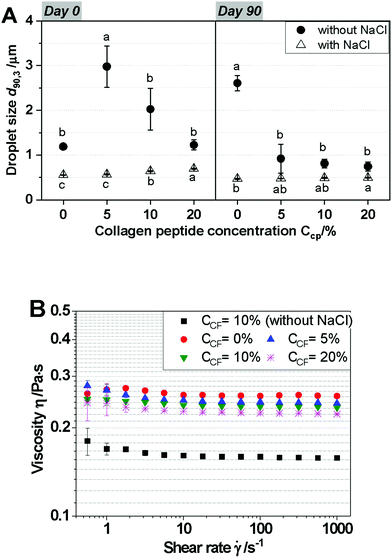 |
| Fig. 2 Characteristics of collagen peptide (CP)-loaded W1/O single emulsions with different CP concentrations in the inner water phase (CCP): (A) droplet size; (B) viscosity. All emulsions were prepared using 40% water fraction in the inner W1/O emulsion (ΦW1). Percent is expressed on a weight basis. Data labeled with different letters (a–d) show statistically significant differences for the same treatment (p < 0.05). | |
Typically, salts or other small molecules are used as osmolytes in the inner water phase to stabilize water-in-oil emulsions against Ostwald ripening. The significant increase in droplet size in CP-loaded W1/O emulsions, especially when no salt was added to the inner water phase, shows that the osmotic effect of CP is less pronounced. In comparison to the osmolytes previously used (NaCl, CaCl2, and glucose),26–29 CP possesses a relatively much larger molecular weight (<3000 Da). However, the W1/O emulsions containing NaCl and CP in the W1-phase also contained larger droplets than those without CP. This could be an indicator of the interference of PGPR and CP, e.g. competitive adsorption, at the interfaces. After 90 days of storage, the d90,3 values of all of the W1/O emulsions were decreased, except in the case of the W1/O emulsions without NaCl and CP. However, no remarkable differences in the changes in d90,3 were observed among the W1/O emulsions containing different amounts of CP. These size reductions may also be attributed to the shrinking behavior of water droplets as above mentioned, and it can be affected by the dissolved osmolytes by alteration of the solubility balance of water in oil.30 Additionally, larger effects of any additional osmolytic substance would be expected at lower osmolality and lower overall osmolyte concentrations (e.g., systems without NaCl). This also explains the small differences in droplet size with storage, as well as the excellent long-term stability of W1/O emulsions containing NaCl and CP.
With respect to emulsion viscosities, all W1/O emulsions exhibited Newtonian behavior (n ∼ 1) regardless of the presence of NaCl and CP concentration (Fig. 2B). The emulsion viscosities decreased slightly as the CP concentration increased from 0.257 Pa s to 0.223 Pa s (
at 1000 s−1) in the presence of NaCl of W1/O emulsions. However, in the absence of NaCl, emulsion viscosities did not significantly change in a CP concentration-dependent fashion (data not shown). Thus, W1/O emulsions with NaCl (green dot) and without NaCl (black dot) were compared to investigate NaCl effects at identical CP concentration. The results showed that the W1/O emulsion with NaCl (η = 0.234 Pa s at
at 1000 s−1) had a much higher viscosity than that without NaCl (η = 0.158 Pa s at
at 1000 s−1), which is most likely the effect of the reduced droplet size (see Fig. 2A).
To evaluate the interaction between the CP (with or without NaCl) and the emulsifier PGPR, the interfacial tension of the water/oil system was examined for a water/pure oil system (no PGPR added) and a water/oil system as used in the emulsion (5% w/w PGPR added). First, to ensure the absence of impurities in both the oil phase and the water phase (with or without NaCl), the water/oil interfacial tension values were measured and determined to be 24.77 mN m−1 (without NaCl) and 21.74 mN m−1 (with NaCl). The interfacial tension of the water/oil system was then measured at different CP concentrations in the water phase (5, 10, or 20% w/w), as represented in Fig. 3. The interfacial tension in the water/pure oil system significantly decreased with the addition of CP to the water phase (Fig. 3A), demonstrating that CP is interfacially active.31 The addition of NaCl exerted only minor effects on the interfacial tension. All of the water/oil + 5% w/w PGPR oil systems showed significantly reduced interfacial tensions compared to the corresponding water/pure oil interface, regardless of the CP concentration. In other words, the CP concentration had no remarkable effect on interfacial tension at the water/oil + 5% w/w PGPR oil interface (Fig. 2B), indicating that PGPR dominated at the interface. However, lower interfacial tension values were measured for the systems containing NaCl, which agrees with previous reports.26,28 Based on these results, we suggest that PGPR at the water/oil interface was more sensitive towards NaCl than to CP.
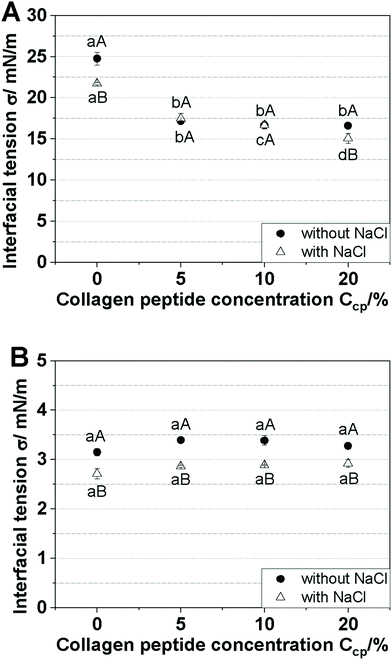 |
| Fig. 3 Interfacial tension between the aqueous phase and oily phase with different collagen peptide concentrations in the aqueous phase: (A) oil phase without PGPR; (B) oil phase with PGPR. Percent is expressed on a weight basis. a–dData labeled with different letters show statistically significant differences depending on the collagen peptide concentration (p < 0.05); A–BData labeled with different letters show statistically significant differences depending on whether NaCl is added (p < 0.05). | |
Overall, the droplet size and stability of W1/O emulsions was more dependent on the water fraction and on the presence of NaCl than on the CP concentration in the water phase. For an efficient encapsulation of bioactive ingredients in double emulsions, small droplets are required; this effect can be achieved by choosing formulations containing NaCl and a low dispersed phase fraction. Low dispersed phase fractions of the inner water phase, however, result in limited overall peptide loads in the emulsion. Therefore, a compromise must be reached between a high amount of encapsulated peptides and high encapsulation efficiency.
3.2 Characterization of the W1/O/W2 double emulsions
3.2.1 Influence of the outer water fraction on the W1/O/W2 emulsions.
To investigate the influence of the outer water fraction ΦW2 on the W1/O/W2 emulsions, all of the W1/O/W2 emulsions were prepared using 10% CP-loaded W1/O single emulsions (40% inner water fraction in the presence of 1% NaCl) as the dispersed phase and 1% Tween 80 solution as the continuous phase. All 10% CP-loaded W1/O/W2 emulsions were successfully produced by different outer water fractions ΦW2 (ESI†). The resulting d90,3 values, span values, and encapsulation efficiencies (EE) at the time of fabrication (day 0) and after 90 days of storage were measured and are shown in Fig. 4. The d90,3 values of the W1/O/W2 emulsions increased with increase in ΦW2, while their EE decreased with increase in ΦW2 from 97.1% (50% ΦW2) to 91.2% (80% ΦW2). After 90 days of storage, the EE of the W1/O/W2 emulsions showed an even more pronounced decreasing tendency with increasing ΦW2 and had EE values of 86.0% (50% ΦW2), 64.2% (60% ΦW2), 62.6% (70% ΦW2), and 53.8% (80% ΦW2), respectively: the EE also could be represented to 17.2%, 10.3%, 7.5% and 4.3%, respectively, when CP amount% was calculated by real water droplet fraction ΦW1. Namely, the higher the inner water fraction of the W1/O/W2 emulsion, the lower was its CP emission (i.e., the higher the encapsulation efficiency). In this study, CP emulsions leading to the instability of W1/O/W2 emulsions could be assumed to occur by two possible mechanisms: (a) rupture of the oil film, resulting in the loss of the internal aqueous droplets; (b) passage of the water and water-soluble substances through the oil layer between both water phases.30,32 This phenomenon can occur by reverse micellar transport created by the lipophilic emulsifier and by simple diffusion across the oil phase in conjunction with osmotic differences between both water phases.30 In detail, CP emission can be the result of the CP concentration gradient between the inner water phase (initially 10% CP) and outer water phase (initially 0% CP). To balance this concentration gradient, CP might diffuse through the oil phase to the outer water phase. This directed diffusion will come to an end once a critical CP concentration in the outer water phase is reached. The higher ΦW2, the more peptide molecules are required to obtain that critical concentration. In reverse, this means that more molecules must diffuse out of the inner water phase, hence the lower EE at high ΦW2. Furthermore, loss of inner water itself did not seem to have occurred, as there were no significant differences in d90,3 or the span values among the emulsions after 90 days of storage. Therefore, loss of inner water would have led to a shrinkage of the outer oil emulsion droplets.
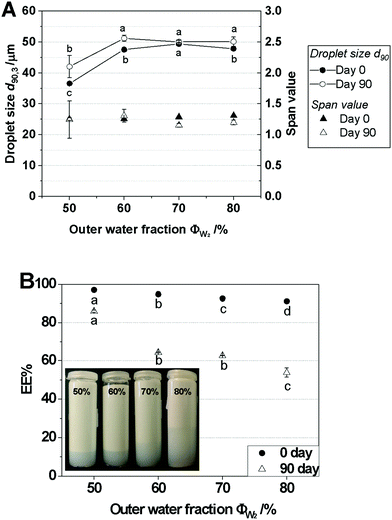 |
| Fig. 4 Characteristic of collagen peptide (CP)-W1/O/W2 double emulsions with different water fractions in the outer W1/O/W2 emulsions (ΦW2): (A) droplet size and span value; (B) encapsulation efficiency/appearance. Appearance (B) is shown after 90 days. All emulsions were prepared under the following conditions: 10% collagen peptide and 1% NaCl concentration in the inner water phase; 40% water fraction in the inner W1/O emulsions. Percent is expressed on a weight basis. Data labeled with different letters (a–d) show statistically significant differences (p < 0.05). | |
The viscosity of W1/O/W2 emulsions was shown to depend on the volume fraction of inner or out water fraction in double emulsion system. As seen in Fig. 5A, the viscosity of the W1/O/W2 emulsions decreased as ΦW2 increased (or ΦW1 decreased); their viscosities were 0.402 s−1 (50% ΦW2), 0.205 s−1 (60% ΦW2), 0.041 s−1 (70% ΦW2), and 0.009 s−1 (80% ΦW2) at a shear rate of 1000 s−1. In addition, the W1/O/W2 emulsions with ΦW2 = 70% and 80% exhibited Newtonian behavior (n ∼ 1). At high ΦW2, the emulsion flow behavior was dominated by the continuous water phase, hence the Newtonian profile and the low viscosity. Conversely, the W1/O/W2 emulsions with ΦW2 = 50% and 60% had flow behavior indices less than unity (n < 1), indicating that they were prone to shear thinning, i.e., their viscosity decreased as the shear rate increased. This shear-thinning behavior is explained by the structural deformation: when the dispersed phase concentration in W1/O/W2 emulsions increased, i.e., when ΦW2, was lower, droplets were more likely to make contact and cause friction during shearing. This increases the emulsion viscosity and, on the other hand, induces shear-thinning behavior when droplets deform in order to pass each other.22,33 Finally, the W1/O/W2 emulsions also showed more pronounced creaming, with an increase in ΦW2 after 90 days of storage (see the image in Fig. 4B), which is a consequence of both the lower viscosity and the larger outer droplet size at high ΦW2.
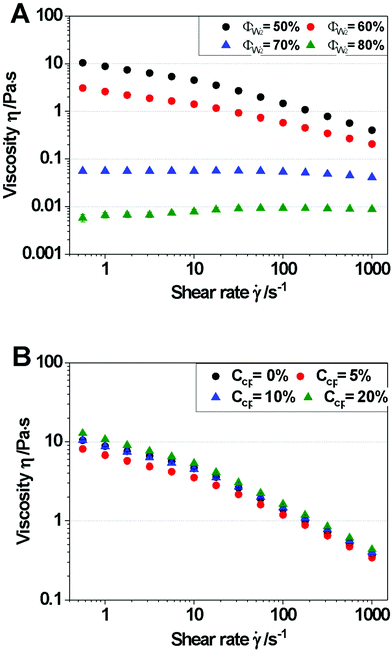 |
| Fig. 5 Viscosity of W1/O/W2 double emulsions: (A) with different water fractions in the outer W1/O/W2 emulsion (ΦW2); (B) with different collagen peptide concentrations in the inner water phase (CCP). All W1/O/W2 double emulsions were prepared using a 40% water fraction in the inner W1/O emulsions with 1% NaCl. Percent is expressed on a weight basis. | |
3.2.2 Influence of the collagen peptide concentration on the W1/O/W2 emulsions.
To investigate the influence of the CP concentration on the W1/O/W2 emulsions, all of the W1/O/W2 emulsions were prepared with W1/O single emulsions (40% inner water fraction in the presence of 1% w/w NaCl or in the absence of NaCl) as the dispersed phase and 1% Tween 80 solution as the continuous phase. An outer water fraction ΦW2 of 50% was chosen because only at that outer water fraction could high EE values of >80% be achieved after 90 days. The resulting d90,3 and encapsulation efficiencies EE of W1/O/W2 emulsions with different CP concentrations were measured on the day of fabrication (day 0) and after 90 days of storage and are shown in Fig. 6. In the absence of NaCl, the d90,3 values of the W1/O/W2 emulsions increased as the CP concentration increased (from 16.38 μm to 32.07 μm), which is caused by slightly higher emulsion viscosity at higher CP concentration (Fig. 5B), indicating enhanced droplet breakup. In contrast, in the presence of NaCl, their d90,3 values decreased as the CP concentration increased (from 39.70 μm to 34.49 μm) (Fig. 6A). Moreover, the W1/O/W2 emulsions with NaCl showed higher d90,3 values than those without NaCl. In particular, the blank-W1/O/W2 emulsion (containing neither NaCl nor CP) showed the smallest d90,3 value of 16.38 μm; this is also shown in Fig. 7. After 90 days of storage, there is no significant difference in d90,3 of W1/O/W2 emulsions at different CP concentrations. Only slight increases in d90,3 were observed for all concentrations after 90 days of storage, which is probably caused by oil droplet coalescence and/or diffusion of outer water into inner water droplets due to the higher osmotic pressure within the inner aqueous phase (due to NaCl and CP).
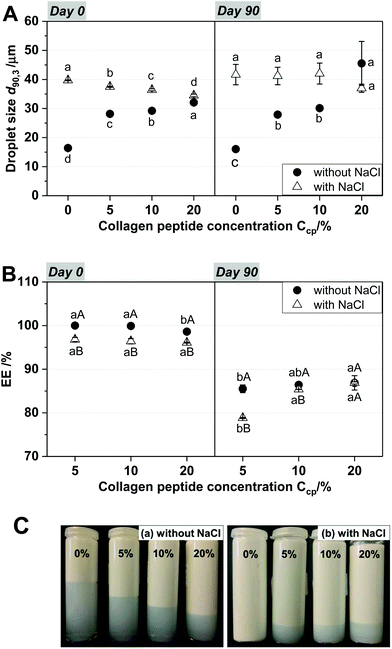 |
| Fig. 6 Characteristics of collagen peptide (CP)-W1/O/W2 double emulsions with different CP concentrations in the inner water phase (Ccp): (A) droplet size; (B) encapsulation efficiency; (C) appearance. Appearance (C) is shown after 90 days. All emulsions were prepared under the following conditions: 1% NaCl concentration in the inner water phase; 40% water fraction in the inner W1/O emulsions; 50% water fraction in the outer W1/O/W2 emulsions. Percent is expressed on a weight basis. a–dData labeled with different letters show statistically significant differences depending on the collagen peptide concentration (p < 0.05); A–BData labeled with different letters show statistically significant differences depending on whether NaCl was added (p < 0.05). | |
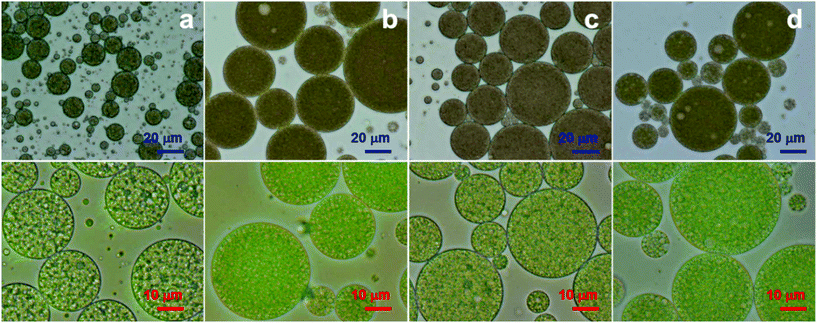 |
| Fig. 7 Microscope images of W1/O/W2 double emulsions with Tween 80: (a) 0% collagen peptide (CP) without NaCl; (b) 0% CP with NaCl; (c) 10% CP without NaCl; (d) 10% CP with NaCl. All emulsions were prepared under the following conditions: 40% water fraction in the inner W1/O emulsions; 50% water fraction in the outer W1/O/W2 emulsions. | |
As seen in Fig. 6B, the EE values of all of the CP-loaded W1/O/W2 emulsions were greater than 96.1%, and the W1/O/W2 emulsions with NaCl showed lower EE values than those without NaCl on the day of fabrication. However, there is no significant difference in EE at different CP concentrations regardless of the addition of NaCl. This indicates that EE% was not affected by CP concentration directly after production of W1/O/W2 emulsions. After 90 days of storage, the EE values of all the emulsions had decreased. Nevertheless, EE values were still high, with EE >78.68%. The reduction of EE value is probably caused by the emission of CP. In Fig. 6A, d90,3 values of the emulsions did not change significantly during prolonged storage; this may be because only the CPs were released from the inner water of the inner emulsion (the W1/O emulsions) to the outer water phase (W2) of the W1/O/W2 emulsions during prolonged storage, as previously described. The emission of only CP from the inner emulsions could be due to the small molecular weight (approximately 3 kDa) and amphipathic properties of the CP. Although the addition of NaCl decreased the EE values, it was effective in preventing the creaming of the W1/O/W2 emulsions. Moreover, the creaming of the W1/O/W2 emulsions in the absence of NaCl clearly decreased with an increase in CP concentration, while creaming increased in the presence of NaCl with an increase in CP concentration. In general, creaming could be explained by the large oil droplet size (40 μm). Interestingly, creaming occurred in all of the W1/O/W2 emulsions after 90 days of storage, except for the emulsion containing NaCl without CP (Fig. 6C), although the droplet sizes of emulsions did not change during prolonged storage. Therefore, creaming in emulsion systems with CP in the presence of NaCl might be caused by expressed CP causing depletion flocculation of oil droplets.
In the viscosity measurements, all of the W1/O/W2 emulsions exhibited pronounced shear thinning in the measured shear rate range (Fig. 5B). The CP concentration had slight effects on the flow curve, which suggests that the CP encapsulated in the inner water phase affected the viscosities of the double emulsions only to a very limited extent. In general, osmolytes have been reported to increase the viscosity of W1/O/W2 emulsions, most likely due to the outflow of water from the outer into the inner water phase during the emulsification process.34 However, in the presence of NaCl, the viscosity of the W1/O/W2 emulsions was not influenced by CP, indicating that NaCl demonstrated much stronger activity as an osmolyte than CP when NaCl and CP were both present in the double emulsion system. Additionally, increasing the CP concentration had no remarkable effect on the viscosity, which suggested that the viscosity of W1/O/W2 emulsions was unaffected by the amount of CP encapsulated in the inner water phase.
3.2.3 Influence of hydrophilic emulsifier on the W1/O/W2 emulsions.
From literature, it is known that the choice of the hydrophilic emulsifier can affect the encapsulation efficiency of bioactive substances by stabilizing/destabilizing the entire W1/O/W2 emulsion system.30 Therefore, W1/O/W2 emulsions were prepared using several hydrophilic emulsifiers to determine the best formulation, i.e., which stabilizer provides the highest encapsulation efficiency (EE) for CP. All of the CP-loaded W1/O/W2 emulsions were prepared using W1/O single emulsions (40% inner water fraction in the presence of 10% w/w CP and 1% w/w NaCl) as the dispersed phase and different hydrophilic emulsifier solutions (50% outer water fraction ΦW2) as the continuous phase; all of the hydrophilic emulsifiers were added to the continuous phase in the same concentration, 1% (w/w). The hydrophilic emulsifiers were chosen to cover various types of food-grade emulsifiers. Two small molecule emulsifiers, the synthetic Tween 80 and the natural lecithin, are compared to two hydrocolloid emulsifiers. Both chitosan (cationic) and pectin (anionic) are polymer emulsifiers of much larger molecular weight than either Tween 80 or lecithin. The droplet size distribution Q3, encapsulation efficiency EE, and microscopy images of the resulting emulsions were measured and are shown in Fig. 8. The Q3 results and microscopy images revealed that emulsions prepared with different hydrophilic emulsifiers possessed different droplet sizes; the droplet sizes increased in the order of chitosan (d90,3 = 335 μm) > lecithin (d90,3 = 224 μm) > pectin (d90,3 = 204 μm) > Tween 80 (d90,3 = 37 μm) (Fig. 8A). In comparing Tween 80 and lecithin, this observation can probably be explained by the different hydrophilicities of the emulsifiers. The hydrophilicity of emulsifiers is usually measured by their hydrophilic-lipophilic balance (HLB) values, with higher numbers indicating greater hydrophilicity; emulsifiers with greater hydrophilicity could wrap and stabilize the particles in an O/W emulsion more efficiently, thus resulting in smaller particle sizes.35 Of the employed emulsifiers in this study, the HLB values of Tween 80 and lecithin were 15.0 and 4.3, respectively.35 The higher HLB value of Tween 80 could be responsible for the smaller droplet sizes in the emulsions produced with this emulsifier. Schmidts, et al. (2009)30 suggested that the effect of the hydrophilic emulsifiers on the formulation properties is related not only to HLB values, but additionally to chemical composition. Furthermore, droplet sizes were larger in chitosan or pectin-stabilized W1/O/W2 emulsions than in Tween 80-stabilized W1/O/W2 emulsions. This could be attributed to the molecular weight or structure of emulsifiers. The larger molecular emulsifiers (chitosan and pectin) would prevent close packing of the points of contact with the interface, resulting in decreased reduction of the interfacial tension.36 Due to the low adsorption kinetics, chitosan and pectin could not efficiently and quickly stabilize the droplets. In contrast, small molecule emulsifiers (Tween 80 and lecithin) can be easily adsorbed onto the droplet surface, with a consequent reduction in the interfacial tension, which favors the formation of small droplets.36,37 In addition, small-molecule emulsifiers also more rapidly stabilize droplets against recoalescence during colloid mill production, which also results in smaller droplets.25
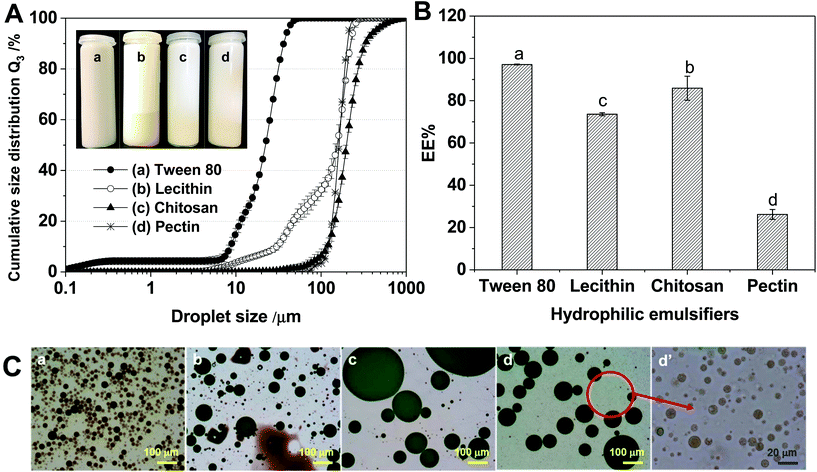 |
| Fig. 8 Characteristics of collagen peptide-loaded W1/O/W2 double emulsions stabilized by (a) Tween 80, (b) lecithin, (c) chitosan, and (d, d′) pectin: (A) size distribution/appearance; (B) encapsulation efficiency (EE%); (C) microscope images. Appearance (A) is shown after 7 days. All emulsions were prepared under the following conditions: 10% collagen peptide and 1% NaCl concentration in the inner water phase; 40% water fraction in the inner W1/O emulsions; 50% water fraction in the outer W1/O/W2 emulsions. Percent is expressed on a weight basis. Data labeled with different letters (a–d) for the results of encapsulation efficiency showed statistically significant differences (p < 0.05). | |
The EEs of the W1/O/W2 emulsions stabilized with different hydrophilic emulsifiers were 97.1% (Tween 80), 73.6% (lecithin), 85.9% (chitosan) and 26.2% (pectin) (Fig. 8B). The Tween 80-stabilized W1/O/W2 emulsion had the smallest droplet size and the highest EE. The lecithin-stabilized W1/O/W2 emulsion showed a somewhat low emulsifying ability (Fig. 8C(b)) because lecithin has a relatively lower HLB value than Tween 80, as previously described. The chitosan-stabilized W1/O/W2 emulsion had the largest droplet size. The pectin-stabilized W1/O/W2 emulsion showed the lowest EE. In comparing lecithin, chitosan and pectin, W1/O/W2 emulsions had different EE% values, although the droplet sizes were within the same order of magnitude. This could indicate that mean droplet size does not affect EE% as a single factor. Droplet size might be relevant for different emulsions of one formulation; however, when the formulation is changed, other effects must be considered. A low EE was demonstrated for the pectin-stabilized W1/O/W2 emulsion compared to proteins, which was previously shown by Frank et al. (2012).15 Furthermore, as seen by the optical microscopy images (Fig. 8C(d and d′)), the pectin-stabilized W1/O/W2 emulsions produced small droplets in the continuous water phase (W2 phase). Here, we could assume that those were O/W2 emulsion droplets. Therefore, the pectin-stabilized W1/O/W2 emulsions would have contained both W1/O/W2 emulsion droplets and O/W2 emulsion droplets. The production of the O/W2 emulsion would have led to instability of the W1/O/W2 emulsion. For these reasons, in this study, the pectin-stabilized W1/O/W2 emulsions showed low encapsulation efficiency and droplet expulsion of W1/O/W2 emulsions. Consequently, we suggest that emulsifiers with pronounced amphiphilicity are required for a stable W1/O/W2 emulsion. Furthermore, additional research will be necessary regarding the interaction between encapsulated substances and hydrophilic emulsifiers.
4. Conclusions
To evaluate whether W1/O/W2 emulsions are suitable encapsulation systems for collagen peptides (CP), emulsions were prepared with various emulsification parameters such as CP and salt (NaCl) concentration, inner water and outer water fraction and type of hydrophilic emulsifier. Inner emulsions (W1/O emulsions) loaded with CP generated significantly higher droplet sizes and the need for NaCl to stabilize the W1-droplets. This effect could result from the osmotic activity of peptides, even when higher in molecular weight, but could also result from the interfacial activity of the peptides. NaCl interacts with a lipophilic emulsifier (PGPR) and reduces interfacial tension, whereas no influence of the interaction between CP and PGPR was detected by interfacial tension measurement.
As for high EE in W1/O/W2 emulsions, small W1/O emulsion droplets are required, and W1/O emulsions were produced in the situations of lower inner water fraction and with NaCl in the W1-phase. High CP concentrations could be encapsulated to increase the bioactive loads in W1/O/W2 emulsions without destabilization. It was also shown that the EE of W1/O/W2 emulsions had a value of >80% regardless of CP concentration. Moreover, the lower the outer water fraction of the W1/O/W2 emulsion, the higher the EE in storage, indicating that an increase in CP (as active substances) load can be achieved through an increase in inner emulsion load.
Hydrophilic emulsifiers are important for inner emulsion stabilization against coalescence but also against the loss of active ingredients. We used different low- and high-molecular size hydrophilic emulsifiers. The Tween 80-stabilized W1/O/W2 emulsion exhibited the highest EE (97.3%) and the smallest droplet size (d90,3 = 37 μm), whereas the pectin-stabilized W1/O/W2 emulsion demonstrated the lowest EE (28.5%). These observations can be partially explained by the fact that emulsifiers with pronounced amphiphilicity were shown to provide better stabilization of double emulsions. However, other effects also apparently played a role and will require further in-depth research.
Conflicts of interest
There are no conflicts of interest to declare.
Acknowledgements
This work was supported by the National Research Foundation of Korea (NRF) through a grant funded by the Korean government (MSIT) (NRF-2018R1C1B6004305).
References
- M. C. Gómez-Guillén, B. Giménez, M. E. López-Caballero and M. P. Montero, Functional and bioactive properties of collagen and gelatin from alternative sources: A review, Food Hydrocolloids, 2011, 25, 1813–1827 CrossRef.
- R. Hartmann and H. Meisel, Food-derived peptides with biological activity: from research to food applications, Curr. Opin. Biotechnol., 2007, 18, 163–169 CrossRef CAS PubMed.
- B. H. Sarmadi and A. Ismail, Antioxidative peptides from food proteins: A review, Peptides, 2010, 31, 1949–1956 CrossRef CAS PubMed.
- B. Wang, L. Li, C.-F. Chi, J.-H. Ma, H.-Y. Luo and Y.-f. Xu, Purification and characterisation of a novel antioxidant peptide derived from blue mussel (Mytilus edulis) protein hydrolysate, Food Chem., 2013, 138, 1713–1719 CrossRef CAS PubMed.
- D. Choi, S. G. Min and Y. J. Jo, Functionality of porcine skin hydrolysates produced by hydrothermal processing for liposomal delivery system, J. Food Biochem., 2018, 42, e12464 CrossRef.
- A. Mohan, S. R. Rajendran, Q. S. He, L. Bazinet and C. C. Udenigwe, Encapsulation of food protein hydrolysates and peptides: a review, RSC Adv., 2015, 5, 79270–79278 RSC.
- Y.-J. Jo, J.-Y. Chun, Y.-J. Kwon, S.-G. Min and M.-J. Choi, Formulation development of multilayered fish oil emulsion by using electrostatic deposition of charged biopolymers, Int. J. Food Eng., 2015, 11, 31–39 CAS.
- Y.-J. Jo, J.-Y. Chun, Y.-J. Kwon, S.-G. Min, G.-P. Hong and M.-J. Choi, Physical and antimicrobial properties of trans-cinnamaldehyde nanoemulsions in water melon juice, LWT–Food Sci. Technol., 2015, 60, 444–451 CrossRef CAS.
- Y.-J. Jo and Y.-J. Kwon, Characterization of β-carotene nanoemulsions prepared by microfluidization technique, Food Sci. Biotechnol., 2014, 23, 107–113 CrossRef CAS.
- J.-Y. Chun, Y.-J. Jo, P. Bjrapha, M.-J. Choi and S.-G. Min, Antimicrobial Effect of α- or β-Cyclodextrin Complexes with Trans-Cinnamaldehyde Against Staphylococcus aureus and Escherichia coli, Drying Technol., 2015, 33, 377–383 CrossRef CAS.
- L. Salvia-Trujillo, S. H. E. Verkempinck, L. Sun, A. M. Van Loey, T. Grauwet and M. E. Hendrickx, Lipid digestion, micelle formation and carotenoid bioaccessibility kinetics: Influence of emulsion droplet size, Food Chem., 2017, 229, 653–662 CrossRef CAS PubMed.
- E. K. Silva, G. L. Zabot, M. A. Bargas and M. A. A. Meireles, Microencapsulation of lipophilic bioactive compounds using prebiotic carbohydrates: Effect of the degree of inulin polymerization, Carbohydr. Polym., 2016, 152, 775–783 CrossRef CAS PubMed.
- M. Mosquera, B. Giménez, I. M. da Silva, J. F. Boelter, P. Montero, M. C. Gómez-Guillén and A. Brandelli, Nanoencapsulation of an active peptidic fraction from sea bream scales collagen, Food Chem., 2014, 156, 144–150 CrossRef CAS PubMed.
- A. Akbarzadeh, R. Rezaei-Sadabady, S. Davaran, S. W. Joo, N. Zarghami, Y. Hanifehpour, M. Samiei, M. Kouhi and K. Nejati-Koshki, Liposome: classification, preparation, and applications, Nanoscale Res. Lett., 2013, 8, 102 CrossRef PubMed.
- K. Frank, E. Walz, V. Gräf, R. Greiner, K. Köhler and H. P. Schuchmann, Stability of anthocyanin-rich W/O/W-emulsions designed for intestinal release in gastrointestinal environment, J. Food Sci., 2012, 77, N50–N57 CrossRef PubMed.
- M. Kaimainen, S. Marze, E. Järvenpää, M. Anton and R. Huopalahti, Encapsulation of betalain into w/o/w double emulsion and release during in vitro intestinal lipid digestion, LWT–Food Sci. Technol., 2015, 60, 899–904 CrossRef CAS.
- A. Schuch, P. Deiters, J. Henne, K. Köhler and H. P. Schuchmann, Production of W/O/W (water-in-oil-in-water) multiple emulsions: droplet breakup and release of water, J. Colloid Interface Sci., 2013, 402, 157–164 CrossRef CAS PubMed.
- R. Mezzenga, B. M. Folmer and E. Hughes, Design of double emulsions by osmotic pressure tailoring, Langmuir, 2004, 20, 3574–3582 CrossRef CAS.
- S. M. Neumann, U. S. van der Schaaf and H. P. Karbstein, Investigations on the relationship between interfacial and single droplet experiments to describe instability mechanisms in double emulsions, Colloids Surf., A, 2018, 553, 464–471 CrossRef CAS.
- A. K. L. Oppermann, M. Renssen, A. Schuch, M. Stieger and E. Scholten, Effect of gelation of inner dispersed phase on stability of (w1/o/w2) multiple emulsions, Food Hydrocolloids, 2015, 48, 17–26 CrossRef CAS.
- N. Garti, Double emulsions—scope, limitations and new achievements, Colloids Surf., A, 1997, 123–124, 233–246 CrossRef CAS.
- M. Matos, G. Gutiérrez, J. Coca and C. Pazos, Preparation of water-in-oil-in-water (W1/O/W2) double emulsions containing trans-resveratrol, Colloids Surf., A, 2014, 442, 69–79 CrossRef CAS.
- I. M. Krieger and T. J. Dougherty, A mechanism for non-Newtonian flow in suspensions of rigid spheres, Trans. Soc. Rheol., 1959, 3, 137–152 CAS.
- M. A. Farah, R. C. Oliveira, J. N. Caldas and K. Rajagopal, Viscosity of water-in-oil emulsions: Variation with temperature and water volume fraction, J. Pet. Sci. Eng., 2005, 48, 169–184 CrossRef CAS.
-
U. S. van der Schaaf and H. P. Karbstein, Fabrication of nanoemulsions by rotor-stator emulsification, in Nanoemulsions, ed. S. M. Jafari and D. J. McClements, Academic Press, 2018, ch. 6, pp. 141–174, DOI:10.1016/B978-0-12-811838-2.00006-0.
- A. L. Márquez, A. Medrano, L. A. Panizzolo and J. R. Wagner, Effect of calcium salts and surfactant concentration on the stability of water-in-oil (w/o) emulsions prepared with polyglycerol polyricinoleate, J. Colloid Interface Sci., 2010, 341, 101–108 CrossRef PubMed.
- S. M. Neumann, I. Scherbej, U. S. van der Schaaf and H. P. Karbstein, Investigations on the influence of osmotic active substances on the structure of water in oil emulsions for the application as inner phase in double emulsions, Colloids Surf., A, 2018, 538, 56–62 CrossRef CAS.
- A. Pawlik, P. W. Cox and I. T. Norton, Food grade duplex emulsions designed and stabilised with different osmotic pressures, J. Colloid Interface Sci., 2010, 352, 59–67 CrossRef CAS PubMed.
- F. Wolf, K. Koehler and H. P. Schuchmann, Stabilization of Water Droplets in Oil with PGPR for Use in Oral and Dermal Applications, J. Food Process Eng., 2013, 36, 276–283 CrossRef CAS.
- T. Schmidts, D. Dobler, C. Nissing and F. Runkel, Influence of hydrophilic surfactants on the properties of multiple W/O/W emulsions, J. Colloid Interface Sci., 2009, 338, 184–192 CrossRef CAS PubMed.
- M. Malmsten, Ellipsometry and reflectometry for studying protein adsorption, Surfactant Sci. Ser., 2003, 110, 539–582 CAS.
- A. T. Florence and D. Whitehill, Some features of breakdown in water-in-oil-in-water multiple emulsions, J. Colloid Interface Sci., 1981, 79, 243–256 CrossRef CAS.
- Y. Saiki, C. A. Prestidge and R. G. Horn, Effects of droplet deformability on emulsion rheology, Colloids Surf., A, 2007, 299, 65–72 CrossRef CAS.
- T. Schmidts, D. Dobler, P. Schlupp, C. Nissing, H. Garn and F. Runkel, Development of multiple W/O/W emulsions as dermal carrier system for oligonucleotides: Effect of additives on emulsion stability, Int. J. Pharm., 2010, 398, 107–113 CrossRef CAS PubMed.
-
D. J. McClements, Food emulsions : principles, practices, and techniques, CRC Press, Boca Raton, 2015 Search PubMed.
-
S. Friberg, K. Larsson and J. Sjoblom, Food emulsions, CRC Press, Boca Raton, 2003 Search PubMed.
- L. Mao, J. Yang, D. Xu, F. Yuan and Y. Gao, Effects of homogenization models and emulsifiers on the physicochemical properties of β-carotene nanoemulsions, J. Dispersion Sci. Technol., 2010, 31, 986–993 CrossRef CAS.
Footnotes |
† Electronic supplementary information (ESI) available. See DOI: 10.1039/c8fo02467g |
‡ These authors contributed equally to this article. |
|
This journal is © The Royal Society of Chemistry 2019 |