DOI:
10.1039/C8FO01962B
(Paper)
Food Funct., 2019,
10, 333-343
Bilberry anthocyanin extract promotes intestinal barrier function and inhibits digestive enzyme activity by regulating the gut microbiota in aging rats
Received
17th October 2018
, Accepted 11th December 2018
First published on 11th December 2018
Abstract
This study was aimed at understanding potential mechanisms regarding bilberry anthocyanin extract consumption and healthy aging and the effects on intestinal barrier function and digestive enzyme activity, through regulating the gut microbiota in aging rats. Medium-dose bilberry anthocyanin extract consumption (20 mg per kg bw per day) was the optimum amount to regulate the intestinal function of aging rats. After consumption, bacteria beneficial to the intestine (Aspergillus oryzae, Lactobacillus, Bacteroides, Clostridiaceae-1, the Bacteroidales-S24-7-group and the Lachnospiraceae_NK4A136_group) were induced to grow, and harmful bacteria (Verrucomicrobia and Euryarchaeota) were inhibited. However, high-dose bilberry anthocyanin extract consumption altered some intestinally beneficial bacteria in an adverse way. There was a correlation between changes in bacterial composition and changes in short-chain fatty acids and the intestinal mucosal barrier. Bilberry anthocyanin extract consumption also decreased the activity of digestive enzymes. Our results suggest that bilberry anthocyanin extract consumption is a potential approach for assisting healthy aging.
1. Introduction
With aging, there are increasing risk factors for humans to develop various chronic diseases, such as type 2 diabetes, obesity,1 Alzheimer's disease,2 cardiovascular disorders3 and cancer.4 Indeed, aging is characterized by a weakened intestinal barrier and systemic inflammation. Although considerable time and much effort have been applied to the prevention and control of these chronic diseases, the influential mechanism for how age-growth processes affect these chronic diseases is still unclear.
In recent years, the crucial role of the gut microbiota in human health has attracted wide attention and much research. The large intestine is home to numerous microorganisms, including bacteria, which could help to maintain homeostasis and overcome many of the challenges associated with using prebiotics as a health-promoting functional ingredient. Changes in the gut microbiota composition are associated with age-associated inflammation and accompanying morbidity.4 Intestinal microbial dysbiosis over time has been considered one of the primary causes of these chronic diseases.5 Previous studies have reported that the gut microbiota (mainly consisting of Bacteroides, Firmicutes, Proteobacteria, Actinobacteria, Fusobacteria and Verrucomicrobia6,7) plays an important role in regulating oxidative stress, the immune system,8 metabolic disease9 and inflammation.10 Accordingly, modulating the gut microbiota is considered an important measure to control the processes of age-related inflammation.
Diet is a key modulator that affects the composition and establishment of gut microbiota.11,12 We are entering an era in which people can improve their health through dieting and analyzing the health effects of food via studying intestinal microbes and their metabolites. It has been reported that diet prebiotics can change the gut microbiota composition through simultaneously increasing the relative abundance of beneficial bacteria and decreasing the relative abundance of harmful bacteria in the human intestine.13
Antioxidants, including vitamins, polyphenols, bioflavonoids, carotenoids, superoxide dismutase, glutathione, and glutathione reductase, have the ability to delay aging and prevent age-related diseases through relieving oxidation.14 Bilberries contain various phenolic compounds, such as flavonoids, phenolic acids, tannins and stilbenes. The high antioxidant potential of bilberry extract can be attributed to it being rich in phenolic compounds and essential mineral constituents. Polyphenols are abundant micronutrients in our diet, and they have been shown to have protective effects on cardiovascular health and aging.15 Anthocyanin, the predominant polyphenolic group in bilberries, has various health-promoting effects. It has been broadly agreed that dietary polyphenols, especially anthocyanin, can modulate the gut microbiota.16,17 However, few in vivo experiments studying anthocyanin have been carried out due to its unstable characteristics. As a special class of flavonoid, anthocyanin is the chemical component that decides the intense color (from vivid red to blue) of a variety of fruits and vegetables, such as purple sweet potatoes, blueberries, black kerneled rice, and bilberries.18 Moreover, anthocyanin has a wide range of biologically active functionality, including anti-inflammatory, anti-oxidant, and anti-tumor, and it can reduce the risk of chronic diseases.19 It is well known that anthocyanin is not easily absorbed in the upper digestive tract, and most of it reaches the large intestinal tract.20 To the best of our knowledge, there is little information on how anthocyanin reduces the risk of aging-related chronic diseases through modulating the gut microbiota. In order to investigate the protective effects of bilberry anthocyanin extract (BA) on aging rats and its potential mechanism, the development of an in vivo aging rat model is necessary and needed.
As studies have reported, women are more vulnerable to developing various age-associated chronic diseases, have a worse quality of life, and more disease-related worries and endocrine symptoms than men;21,22 therefore, in this study, female SD rats were selected for an in vivo aging rat model. We designed a study to determine whether BA has potential anti-aging activity. The effects of BA, in terms of modulating the gut microbiota and promoting intestinal barrier function, were evaluated via high-throughput sequencing and sandwich-type enzyme immunoassay assays. The inhibitory effects of BA on digestive enzyme activity and the promoting effects of BA on short-chain fatty acids (SCFAs) were also assessed.
2. Research design and methods
2.1. HPLC-MS analysis
Bilberries (Vaccinium myrtillus L) were grown in the Da Hinggan Mountains Area, Inner Mongolia, China. Bilberries were extracted and purified according to the method previously reported by Yu et al.23 The purified anthocyanin extract was stored at −80 °C until further use. HPLC-MS experiments were carried out using an LCMS-IT-TOF spectrometer (Shimadzu, Japan) equipped with a PDA detector. PDA detection was set for 200–650 nm in ESI (+) mode. The m/z mass range was selected as 100–1000. An Agilent ZORBAX SB-C18 column (1.8 μm, 10 cm × 4.6 mm i.d.) was used. A two-phase gradient system was used with water/formic acid (97/3, v/v) and methanol as mobile phase A and mobile phase B, respectively. The experimental temperature was set at 25 °C. The gradient started with 5% mobile phase B in isocratic elution mode for 0.01 min, reaching 9% mobile phase B at 1.2 min, 16% mobile phase B at 14 min, 30% mobile phase B at 28 min, and 50% mobile phase B at 34 min. The gradient reached the initial condition at 35 min. A BA sample (3 μL) at a concentration of 1 mg mL−1 was injected into the C18 column of the HPLC system. Delivery was at a flow rate of 0.8 mL min−1.
2.2. Animals and treatment
The animal study in this experiment was approved by the National Laboratory Animal Ethics Committee of China and Tianjin University of Science and Technology (TUST2017925). All procedures conformed to the National Institutes of Health Guide for Care and Use of Laboratory Animals. The female SD rats (10 rats that were 4 months old as the young group and 50 rats that were 12 months old as the old group) were purchased from the Beijing Laboratory Animal Center of the Academy of Military Medical Sciences. Allowing access to food and water ad libitum, the experimental rats were housed in a room under 12 h/12 h light/dark cycles at 22 ± 2 °C and acclimated for 1 week prior to all studies. The basic diet, including 18% protein, 10% moisture, 8% ash specification, 5% crude fiber, 4% crude fat, 1–1.8% calcium, 0.6–1.2% phosphorus, 0.82% lysine and 0.3–0.8% salt, was purchased from the Beijing Laboratory Animal Center of the Academy of Military Medical Sciences. The rats were randomly divided into 6 experimental groups (10 in each group). The young control (YC) group and old control (AC) group were given distilled water. The rats used in the present experiment took in Catherine genistein and BA via oral gavage. In the positive control (PC) group, Catherine genistein was administered via gavage at a dose of 500 mg per kg bw per day. In the low-dose bilberry anthocyanin (LBA) group, medium-dose bilberry anthocyanin (MBA) group and high-dose bilberry anthocyanin (HBA) group, BA was gavage administered at a dose of 10 mg per kg bw per day, 20 mg per kg bw per day and 40 mg per kg bw per day, respectively. The amount of BA was based on the recommended adult human dose of about 100 mg per day. The doses taken by the three groups were 5, 10 and 20 times the recommended daily BA intake, respectively. During the 10-week experimental period, no adverse effects were observed for all rats. Then, rats were anesthetized and blood withdrawal was made through the femoral artery after 12 h of fasting. Serum samples, the cecal content, the small intestine and the colon were collected, flash frozen in liquid nitrogen, and stored at −80 °C for further use.
2.3. 16S rDNA high-throughput sequencing
Analyses and comparisons of gut microbiota were carried out using high-throughput sequencing of the 16S V4 region. 12 cecal samples coming from the AC, YC and PC groups and 15 cecal samples coming from the LBA, MBA, and HBA groups were aseptically collected, respectively. The total genomic DNA of the samples was extracted via the CTAB method. Duplication was carried out via a polymerase chain reaction (PCR) amplicon method using universal primers (515F and 806R), Phusion® High-Fidelity PCR Master Mix (New England Biolabs) and high-efficiency high-fidelity enzymes. The purification of the PCR product was performed using a GeneJET™ Gel Extraction Kit (Thermo Scientific).
Sequencing libraries were generated using an Ion Plus Fragment Library Kit 48 rxns (Thermofisher), following the manufacturer recommendations. The library quality was assessed using a Qubit@ 2.0 fluorometer (Thermo Scientific). The library was sequenced on an Ion S5™ XL platform and 400 bp/600 bp single-end reads were generated. Sequences were clustered into operational taxonomic units (OTUs) based on an OTU threshold of 97% similarity using Uparse software. The alpha-diversity, the beta-diversity and the linear discriminant analysis effect size (LEfSe) were analyzed and the phylogenetic tree was constructed.24
2.4. Determination of SCFAs
SCFAs are generated from the fermentation of carbohydrates. SCFAs in the rat cecal contents were determined via gas chromatography, equipped on an Agilent 7890A GC system with an HP-INNO WAX column (Agilent 19091N-133; 260 °C; 30 m × 250 μm × 0.25 μm; in: back SS inlet N2; out: back detector FID) and a flame ionization detector (Agilent Technologies Inc., CA, USA). Briefly, standard solutions of acetic, propionic, butyric, valeric, isobutyric, and isovaleric acids (Supelco 46975-U, Sigma) were prepared at concentrations of 0.05, 0.10, 0.15, 0.20, 0.25 and 0.30 μL mL−1, respectively. Standard curves of the SCFAs were obtained with the concentration as the abscissa (X) and the standard peak area as the ordinate (Y).25,26
0.1 g of cecal contents was added to a centrifuge tube containing 1 mL of 0.9% formic acid solution. Then the mixed solution was homogenized on a vortex for about 1 min and centrifuged for 10 min at 13
000 rpm. 800 μL of supernatant was extracted with 800 μL of ethyl acetate for 12 h and centrifuged for another 10 min at 13
000 rpm. After that, the supernatant was filtered through a 0.22 μm filter membrane and transferred to a centrifuge tube before injection into the GC instrument.
The operating procedure was performed using the conditions of an initial temperature of 100 °C for 1 min, followed by heating to 140 °C at 4 °C min−1. The signal was detected at 240 °C with an FID detector. The flow rate of H2 was 30 mL min−1, the flow rate of air was 300 mL min−1 and the flow rate of carrier gas (N2) was 20 mL min−1. Then, 1 μL of the respective standard solutions was loaded at 250 °C into the GC system.
2.5. Determination of digestive enzymes
The activities of selected digestive enzymes (α-glucosidase, β-glucosidase, α-galactosidase, β-galactosidase and β-glucuronidase) released into the cecal environment were determined according to the release rate of p-nitrophenol or o-nitrophenol from their nitrophenyl glucosides.27 The substrates were as follows: p-nitrophenyl-α-D-glucopyranoside and p-nitrophenyl-β-D-glucopyranoside for α-glucosidase and β-glucosidase, respectively; p-nitrophenyl-α-D-galactopyranoside and o-nitrophenyl-β-D-galactopyranoside for α-galactosidase and β-galactosidase, respectively; and p-nitrophenyl-β-D-glucuronide for β-glucuronidase. 1 g of cecal contents was added to a centrifuge tube containing 1.5 mL of 100 mmol L−1 PBS (pH 7.0) solution and this was homogenized on a vortex for about 1 min and centrifuged for 15 min at 7211 g min−1: the reaction mixture contained 0.2 mL of supernatant and 0.3 mL of substrate solution (5 mmol L−1). The reaction mixture was incubated for 10 min at 37 °C, then 2.5 mL of 0.25 mol L−1 cold sodium carbonate was added to stop the reaction. p-Nitrophenol and o-nitrophenol were quantified at 400 nm and 420 nm, respectively. The activities of the selected digestive enzymes were expressed as μmol product formed per h per g of digesta.
2.6. The morphological observation of colon and small intestine tissue
Fresh colons and small intestines were excised quickly and fixed in 4% paraformaldehyde. Fixed tissues were embedded with paraffin and processed via hematoxylin–eosin (HE) staining. Slides were examined under light microscopy.
2.7. Enzyme-linked immunosorbent assay (ELISA)
Approximately 0.1 g of frozen colon tissue sample was weighed and homogenized in 1 mL of ice-cooled 0.9% normal saline with a Scientz-48 tissue grinder, and this was centrifuged at 3000 rpm for 10 min at 4 °C. The supernatant was collected and the levels of tumor necrosis factor α (TNF-α) and interleukin 6 (IL-6) were measured using a sandwich-type enzyme immunoassay kit (Abcam, USA), according to the manufacturer instructions.
The serum levels of lipopolysaccharides (LPS) and D-lactic acid (D-LA) were measured using a sandwich-type enzyme immunoassay kit (Nanjing Jiancheng Bioengineering Institute, China), according to the manufacturer instructions.
2.8. Statistical analysis
The statistical analyses were completed using IBM SPSS Statistics 22.0 and Qiime Software (Version 1.9.1). The t-test was performed to determine the difference between groups. P < 0.05 and P < 0.01 indicate that the differences reach the levels of statistical significance and statistical high significance, respectively.
3. Results and discussion
3.1. The identification and quantification of anthocyanin in BA via HPLC-MS methods
Chromatograms of BA were analyzed via HPLC-MS methods. Ten characteristic peaks were identified at the absorption threshold of 525 nm. The relative anthocyanin content values were determined via a peak area normalization method. 10 anthocyanins were determined in the extract by a literature comparison: delphinidin 3-O-galactoside (12.359%); delphinidin 3-O-glucoside (11.801%); cyanidin 3-O-galactoside (20.039%); cyanidin 3-O-glucoside (9.309%); petunidin 3-O-galactoside (4.462%); petunidin 3-O-glucoside (14.715%); petunidin 3-O-arabinoside (4.124%); peonidin 3-O-glucoside (7.688%); malvidin 3-O-glucoside (8.214%); and malvidin 3-O-arabinoside (3.424%), which was consistent with a previous study.28 It has been reported that of the 10 anthocyanins identified by HPLC-ESI-MS, malvidin 3-O-galactoside and malvidin 3-O-glucoside should be major anthocyanins; however, conversely to these studies, 3 new anthocyanins, delphinidin 3-O-arabinoside, cyanidin 3-O-arabinoside, and peonidin 3-O-galactoside, were identified in the study of M. C. Díaz-García et al. This may perhaps be due to different varieties or culturing conditions of anthocyanins being used.
3.2. The composition and diversity of the gut microbiota in rats with different consumption levels
BA consumption has a correlation with shifts within the gut microbiome. The alpha-diversity of gut microbiota based on the observed species is shown in Fig. 1A. No significant differences in the observed species index, richness and diversity scores were observed between the PC, LBA, MBA and HBA groups and the AC group, indicating that the uniformity, richness and complexity of the aging rat intestinal microbiota were not significantly altered after BA consumption. However, this difference was not important, since the inter-individual differences in the compositions of the intestinal microbiota were greater than the therapeutic effect. The β-diversity data (PCoA and UniFrac) from the BA consumption and control groups are shown in Fig. 1B and C. PCoA analysis showed that BA consumption did not significantly affect the complexity of the gut microbiota. The weighted UniFrac analysis showed clear clustering in the intestinal microbiota between different groups. The YC group was similar to the LBA, MBA and HBA groups in terms of the composition of the intestinal microbiota, and was different to the AC and PC groups, which indicated that BA could keep the intestines healthy through regulating the composition of the aging rat intestinal microbiota.
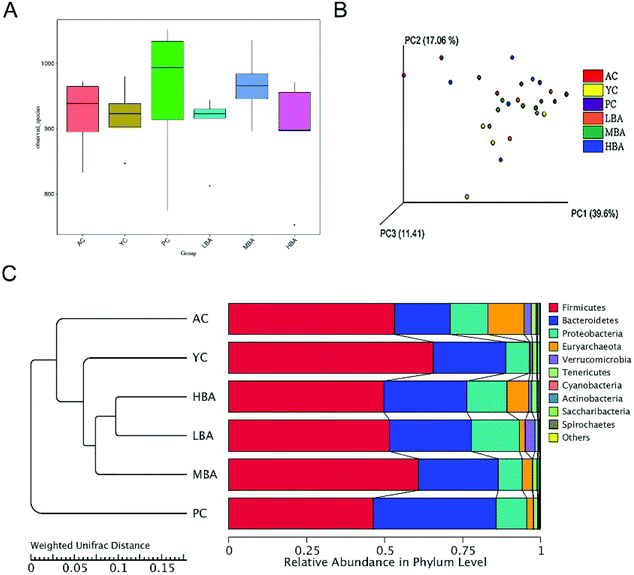 |
| Fig. 1 The age-dependency and diet-responsiveness of rat gut microbiota structures. (A) The α-diversity of gut microbiota based on the observed species. (B) The β-diversity of gut microbiota based on PCoA. (C) The β-diversity of gut microbiota based on the weighted uniFrac distance. | |
The correlation between specific consumption levels and the shift in the Firmicutes/Bacteroidetes ratio is a matter of controversy. As shown in Fig. 1C, the Firmicutes/Bacteroidetes ratio increased (2.83 to 2.99) with the age growth of rats. The predominant phylum began to shift from Firmicutes (53.18% before MBA consumption vs. 58.18% after MBA consumption) in the direction of Bacteroidetes (17.81% vs. 29.25%, respectively). Additionally, it has been reported that the Firmicutes/Bacteroidetes ratio is positively correlated with obesity.29 MBA consumption significantly reduced the Firmicutes/Bacteroidetes ratio from 2.99 to 1.99 (p < 0.01), suggesting that BA might have a potential anti-obesity effect. This result was consistent with a previous study.20 Moreover, significant decreases in the relative abundances of Verrucomicrobia (from 0.0236 to 0.0016) and Euryarchaeota (from 0.1162 to 0.0325) were identified after MBA consumption.
After MBA consumption, significant increases in the relative abundances of Lactobacillus and Bacteroides and a decrease in Methanobrevibacter were observed, as shown in Fig. 2. However, LBA and HBA consumption altered the gut microbiota in an adverse way. Generally, Lactobacillus is beneficial to the host by means of creating a barrier against the colonization of pathogens in the cecum. As a result, MBA might inhibit the growth of pathogens by promoting the growth of certain specific bacteria. This result was similar to a previous report about how L. ruthenicum anthocyanin increased the relative abundance of Bacteroides.20
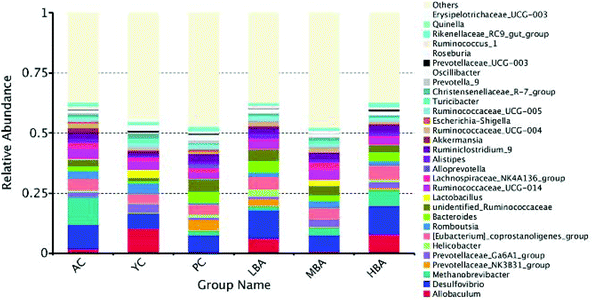 |
| Fig. 2 The differences between gut microbiota at the genus-level in rats induced by different consumption levels. | |
MBA might have a potential anti-inflammatory effect by promoting the growth of Bacteroidales-S24-7-group and Lachnospiraceae_NK4A136_group. Family-level heat map analysis suggests that MBA consumption significantly increases the relative abundance of Clostridiaceae-1, Bacteroidaceae, Bacteroidales-S24-7-group, Lachnospiraceae and Prevotellaceae (Fig. 3). LEfSe analysis suggested that a relatively high proportion of Methanobacteria.Methanobacteriales.Methanobacteriaceae.Methanobrevibacter was observed in the AC group, Bacilli.Lactobacillales.Lactobacillaceae.Lactobacillus was observed in the YC group and Lachnospiraceae.Lachnospiraceae_NK4A136_group was observed in the YC and MBA groups (Fig. 4A–D). Bacteroidales-S24-7-group and Lachnospiraceae_NK4A136_group are considered to be intestinal probiotics with a negative correlation with intestinal inflammation.30,31 However, there are no significant changes in the LBA and HBA groups.
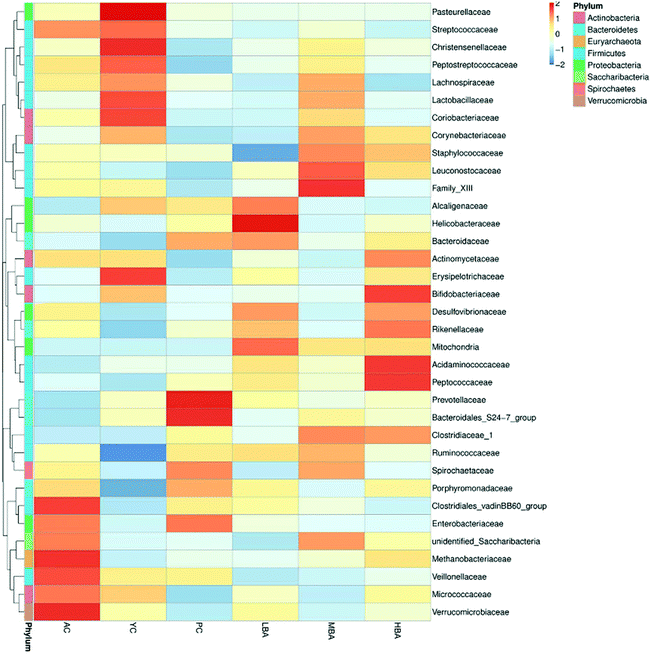 |
| Fig. 3 The differences between gut microbiota at the family-level in rats induced by different consumption levels. | |
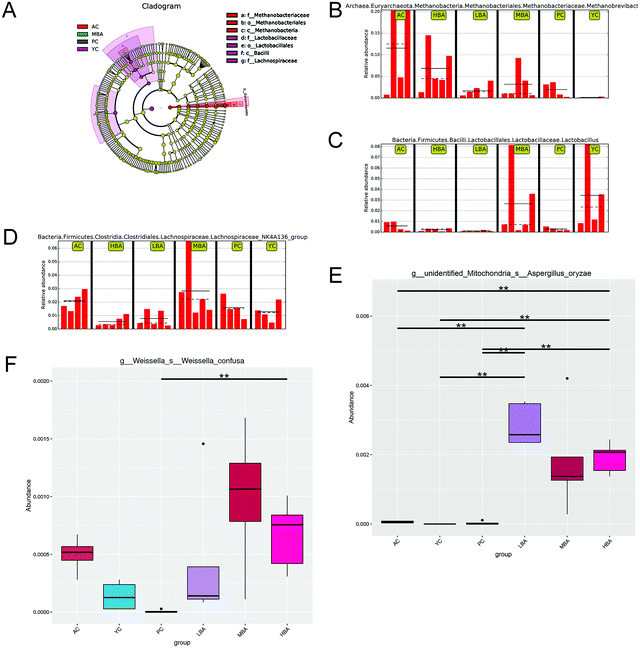 |
| Fig. 4 The differences in gut microbiota as a result of age growth and BA consumption were identified using LEfSe and MetaStat. (A) LEfSe results for gut microbiota based on a cladogram. (B, C and D) LEfSe results for gut microbiota based on the LDA Score (log 10). (E and F) The MetaStat-based results for gut microbiota. ** indicates a highly significant difference (P < 0.01). | |
Therefore, BA might ameliorate the structure of the intestinal microbiota by promoting the growth of gut probiotics and inhibiting the growth of spoilage. At the species level, MetaStat analysis suggested that MBA consumption significantly increased the relative abundance of Aspergillus oryzae (P < 0.01) and Weissella confuse (Fig. 4E and F). Aspergillus oryzae has been reported to be positively correlated with intestinal microbial balance.32 It directly participates in intestinal barrier function and promotes the growth of gut probiotics. Weissella confuse has the highest β-galactosidase activity and inhibiting function of spoilage bacteria. Zhang et al. reported that the fermentation of Weissella confusa could improve the inhibitory effects of quercetin on some human cancer cells.33
3.3. Effects of BA on SCFAs in rat cecal contents
The effect of BA on SCFAs in the cecal contents is shown in Fig. 5A. A significant decrease in the amounts of acetic acid, propionic acid and butyric acid in the cecal contents with the aging of rats was identified (P < 0.01). In general, BA consumption could significantly promote the generation of SCFAs (acetic acid, propionic acid and butyric acid) via the fermentation of carbohydrates in the digestive tract (P < 0.01).
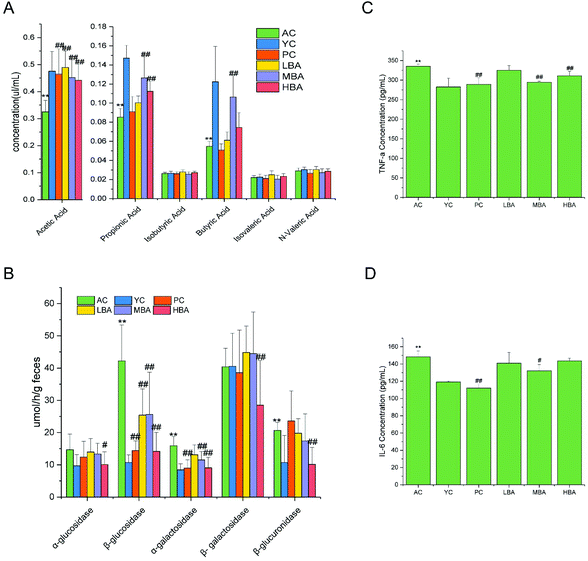 |
| Fig. 5 BA consumption alters gut bacteria fermentation and cecal contents digestive enzyme activity in aging rats. (A) SCFA production in intestinal digesta. (B) Cecal contents digestive enzyme activity. (C) The level of TNF-α in colon tissue. (D) The level of IL-6 in colon tissue. Data are presented as mean ± standard deviation (n = 10). * indicates a significant difference compared with YC, and # indicates a significant difference compared with AC (P < 0.05). ** indicates a highly significant difference with respect to YC, and ## indicates a highly significant difference with respect to AC (P < 0.01). | |
According to the results of correlated research in recent years, BA might promote the conversion of lactate into butyrate via gut microbial fermentation in rats through modulating the growth of starch-utilizing and butyrate-producing bacteria.5 This promotion was consistent with changes in starch-utilizing bacteria (Lactobacillus) and butyrate-producing bacteria (Lachnospiraceae and Ruminococcaceae) in the cecal contents. In general, BA could promote the generation of SCFAs via regulating the intestinal microbes. Therefore, the targeted restoration of these SCFA producers might present a novel ecological approach to managing the inflammatory state of the elderly, which is consistent with a previous study.34
3.4. Effects of BA on digestive enzyme activities
BA consumption could significantly decrease the activities of the digestive enzymes in the cecal contents. The modulation of microbial activity was indicated through changes in the digestive enzyme activities in the cecal contents, as shown in Fig. 5B. Compared with the YC group, the activities of the digestive enzymes β-glucosidase, β-galactosidase and β-glucuronidase released into the cecal environment in the AC group were significantly increased (P < 0.01). The addition of BA extract to the diet significantly decreased the activities of the digestive enzymes α-glucosidase, β-glucosidase, α-galactosidase, β-galactosidase and β-glucuronidase in the cecum (P < 0.05).
3.5. Effects of BA on intestinal mucosal barrier function in rats
The effects of BA on rat intestinal mucosal morphology are shown in Fig. 6A and B. During the process of rat aging, the level of inflammation in the colon is increased, while inflammatory factors could be restored to normal levels with MBA consumption. LBA and HBA consumption rendered more severe the morphological features of colitis. The shortening of the small intestine, the shedding of villi, the structural destruction of tight junctions, as well as abnormal desmosomes were observed for the AC, PC and LBA groups. The intestines of rats in the MBA group displayed smooth villi and normal glands. This result suggested that MBA consumption could restore the damaged mucosa to some degree.
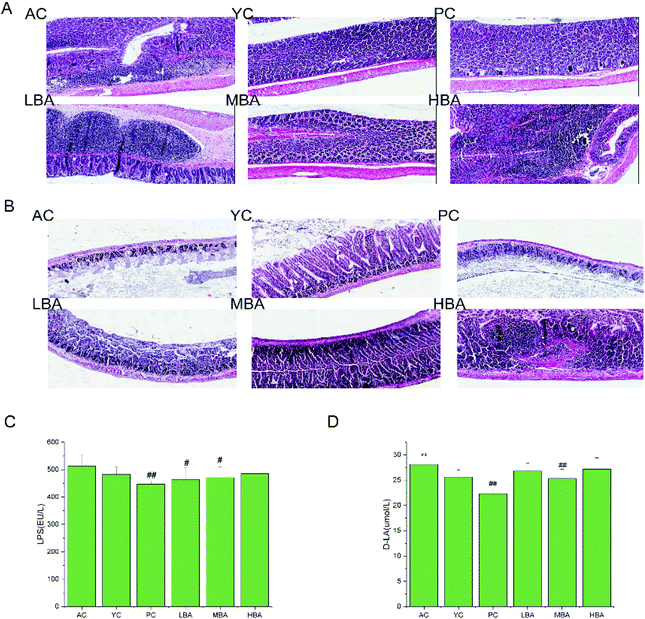 |
| Fig. 6 BA consumption alters the intestinal barrier function of aging rats. (A) Microscopic images of the colon. (B) Microscopic images of the small intestine. (C) The level of LPS in serum. (D) The level of D-LA in serum. | |
The indicators of TNF-α and IL-6 in rat colon tissue are identified in Fig. 5C and D. The ELISA results showed that during the process of rat aging, the levels of TNF-α and IL-6 in the colon are increased. MBA consumption could significantly decrease the levels of colon TNF-α and IL-6 (P < 0.05). We discovered that the changing patterns of TNF-α and IL-6 were similar to the changes in rat intestinal mucosal morphology.
The indicators of intestinal mucosal barrier function in rat serum are identified in Fig. 6C and D. The ELISA results show that MBA consumption could significantly increase the level of serum D-LA with age in rats (P < 0.01). The levels of D-LA in the PC and MBA groups and the levels of LPS in the PC, LBA and MBA groups became lower after the consumption of MBA than in the AC group (P < 0.01). The increase in the release of D-LA from the intestine to serum indicated that age growth could promote intestinal damage and increased intestinal permeability, while MBA consumption could alleviate injuries to intestinal barrier function.
The elderly are known to have an altered gut microbiota composition. One major consequence of altered microbial compositions is the diminished integrity of the intestinal barrier. As a result of the reduced intestinal barrier, the microbiota compositions in the host epithelium and sub-epithelial elements might become compromised. D-LA is a metabolite product coming from the fermentation of intestinal microbes. When the permeability of intestinal mucosa increases, D-LA produced by intestinal microbes can enter the blood through the intestinal mucosa. Therefore, the level of serum D-LA is a major indicator for evaluating intestinal barrier function and intestinal mucosal permeability.35,36 A weakened intestinal barrier allows for the leakage of LPS produced by Gram-negative bacteria through the barrier into systemic circulation. A high level of LPS could cause endotoxemia and systemic inflammation, therefore worsening metabolic disease. During the process of rat aging, the levels of D-LA and LPS in serum are increased, while BA consumption could restore inflammatory factors to normal levels through reducing the levels of D-LA and LPS. This phenomenon was consistent with changes in the cecal contents with respect to starch-utilizing bacteria (Aspergillus oryzae) and SCFAs. Similar findings were noted in db/db mice after the administration of butyrate.37
Overall, the structural determinants for the inhibition of digestive enzymes by BA may be related to structure–activity relationships of natural anthocyanin. BA promoted the generation of SCFAs (acetic acid, propionic acid and butyric acid) through regulating the intestinal microbial flora. The SCFAs not only served as energy sources for intestinal mucosa, but also played a crucial role in maintaining the integrity of the intestinal barrier. Bio-antagonism occurs between gut microbiota, and the normal gut microbiota forms an intestinal mucosal barrier through adhering, colonizing and multiplying. Through antagonism, BA repels intestinal mucosal invasions by harmful bacteria to achieve complex and dynamic equilibrium between the human body and the gut microbiota, thereby staging an anti-aging intervention.
4. Conclusions
In this study, BA was successfully applied to promoting intestinal barrier function via modulating the gut microbiota. MBA consumption (20 mg per kg body weight) created the optimum conditions for regulating the intestinal function of aging rats. After MBA consumption, the growth of bacteria beneficial to the intestines, such as Lactobacillus, bacteroides and allobaculum, was induced and the growth of bacteria harmful to the intestine (Verrucomicrobia and Euryarchaeota) was inhibited, which promoted the acetic acid, propionic acid and butyric acid content. However, HBA consumption altered some bacteria beneficial to the intestines in an adverse way. The promotion of SCFAs (acetic acid, propionic acid and butyric acid) provided energy sources for intestinal epithelium cells and maintained the function of the intestinal mucosal barrier. Bilberry anthocyanin extract consumption also decreased the activity of digestive enzymes. In brief, BA has promising prospects for exploitation due to its value in improving healthy aging through regulating gut microbiota and enhancing intestinal function.
Abbreviations
BA | Bilberry anthocyanin extract |
YC | Young control |
AC | Old control |
PC | Positive control |
LBA | Low-dose bilberry anthocyanin |
MBA | Medium-dose bilberry anthocyanin |
HBA | High-dose bilberry anthocyanin |
SCFAs | Short-chain fatty acids |
OTUs | Operational taxonomic units |
LEfSe | Linear discriminant analysis effect size |
ELISA | Enzyme-linked immunosorbent assay |
TNF-α | Tumor necrosis factor α |
IL-6 | Interleukin 6 |
LPSs | lipopolysaccharides |
D-LA |
D-Lactic acid |
Conflicts of interest
The authors declare no competing financial interests.
Acknowledgements
The Tianjin One Belt And One Road Technological Innovation Project (18PTZWHZ00080), Tianjin Science and Technology Fund Planning Project (18ZYPTJC00020), National Natural Science Foundation of China (31501475) and Research Project of the Tianjin Education Commission (2017ZD02) are gratefully acknowledged.
References
- D. G. A. Burton and R. G. A. Faragher, Obesity and type-2 diabetes as inducers of premature cellular senescence and ageing, Biogerontology, 2018, 1–13 Search PubMed.
- J. D. Hoffman, I. Parikh, S. J. Green, G. Chlipala, R. P. Mohney, M. Keaton, B. Bauer, A. M. S. Hartz and A. Lin, Age Drives Distortion of Brain Metabolic, Vascular and Cognitive Functions, and the Gut Microbiome, Front. Aging Neurosci., 2017, 9, 1–14 Search PubMed.
- H. Shakeri, K. Lemmens, A. B. Gevaert, G. R. Y. De Meyer and V. F. M. Segers, Cellular senescence links aging and diabetes in cardiovascular disease, Am. J. Physiol.: Heart Circ. Physiol., 2018, 315, H448–H462 CrossRef CAS PubMed.
- N. Thevaranjan, A. Puchta, C. Schulz, A. Naidoo, J. C. Szamosi, C. P. Verschoor, D. Loukov, L. P. Schenck, J. Jury, K. P. Foley, J. D. Schertzer, M. J. Larché, D. J. Davidson, E. F. Verdú, M. G. Surette and D. M. E. Bowdish, Age-Associated Microbial Dysbiosis Promotes Intestinal Permeability, Systemic Inflammation, and Macrophage Dysfunction, Cell Host Microbe, 2017, 21, 455–466 CrossRef CAS.
- A. M. Valdes, L. Walter, E. Segal and T. D. Spector, Role of the gut microbiota in nutrition and health, BMJ [Br. Med. J.], 2018, 361, 36–44 Search PubMed.
- R. Tojo, A. Suarez, M. G. Clemente, C. G. de Los Reyes-Gavilan, A. Margolles, M. Gueimonde and P. Ruas-Madiedo, Intestinal microbiota in health and disease: Role of bifidobacteria in gut homeostasis, World J. Gastroenterol., 2014, 20, 15163–15176 CrossRef PubMed.
- L. Vitetta, D. Briskey, H. Alford, S. Hall and S. Coulson, Probiotics, prebiotics and the gastrointestinal tract in health and disease, Inflammopharmacology, 2014, 22, 135–154 CrossRef PubMed.
- H. Zhang, J. B. Sparks, S. V. Karyala, R. Settlage and X. M. Luo, Host adaptive immunity alters gut microbiota, ISME J., 2015, 9, 770–781 CrossRef CAS PubMed.
- D. Rothschild, O. Weissbrod, E. Barkan, A. Kurilshikov, T. Korem, D. Zeevi, P. I. Costea, A. Godneva, I. N. Kalka, N. Bar, S. Shilo, D. Lador, A. V. Vila, N. Zmora, M. Pevsner-Fischer, D. Israeli, N. Kosower, G. Malka, B. C. Wolf, T. Avnit-Sagi, M. Lotan-Pompan, A. Weinberger, Z. Halpern, S. Carmi, J. Fu, C. Wijmenga, A. Zhernakova, E. Elinav and E. Segal, Environment dominates over host genetics in shaping human gut microbiota, Nature, 2018, 555, 210–215 CrossRef CAS PubMed.
- K. Atarashi, W. Suda, C. Luo, T. Kawaguchi, I. Motoo, S. Narushima, Y. Kiguchi, K. Yasuma, E. Watanabe, T. Tanoue, C. A. Thaiss, M. Sato, K. Toyooka, H. S. Said, H. Yamagami, S. A. Rice, D. Gevers, R. C. Johnson, J. A. Segre, K. Chen, J. K. Kolls, E. Elinav, H. Morita, R. J. Xavier, M. Hattori and K. Honda, Ectopic colonization of oral bacteria in the intestine drives T(H)1 cell induction and inflammation, Science, 2017, 358, 359–365 CrossRef CAS PubMed.
- S. J. Clements and S. R. Carding, Diet, the intestinal microbiota, and immune health in aging, Crit. Rev. Food Sci., 2018, 58, 651–661 CrossRef PubMed.
- S. H. Ng, M. Stat, M. Bunce and L. W. Simmons, The influence of diet and environment on the gut microbial community of field crickets, Ecol. Evol., 2018, 8, 4704–4720 CrossRef CAS PubMed.
- C. Antza, S. Stabouli and V. Kotsis, Gut microbiota in kidney disease and hypertension, Pharmacol. Res., 2018, 130, 198–203 CrossRef CAS PubMed.
- A. C. Mayta-Apaza, E. Pottgen, J. De Bodt, N. Papp, D. Marasini, L. Howard, L. Abranko, T. Van de Wiele, S. Lee and F. Carbonero, Impact of tart cherries polyphenols on the human gut microbiota and phenolic metabolites in vitro and in vivo, J. Nutr. Biochem., 2018, 59, 160–172 CrossRef CAS PubMed.
- S. Khurana, K. Venkataraman, A. Hollingsworth, M. Piche and T. C. Tai, Polyphenols: Benefits to the Cardiovascular System in Health and in Aging, Nutrients, 2013, 5, 3779–3827 CrossRef CAS PubMed.
- G. Jamar, D. Estadella and L. P. Pisani, Contribution of anthocyanin-rich foods in obesity control through gut microbiota interactions, BioFactors, 2017, 43, 507–516 CrossRef CAS PubMed.
- A. Faria, I. Fernandes, S. Norberto, N. Mateus and C. Calhau, Interplay between Anthocyanins and Gut Microbiota, J. Agric. Food Chem., 2014, 62, 6898–6902 CrossRef CAS PubMed.
- D. Li, P. Wang, Y. Luo, M. Zhao and F. Chen, Health benefits of anthocyanins and molecular mechanisms: Update from recent decade, Crit. Rev. Food Sci., 2017, 57, 1729–1741 CrossRef CAS PubMed.
- L. Xie, H. Su, C. Sun, X. Zheng and W. Chen, Recent advances in understanding the anti-obesity activity of anthocyanins and their biosynthesis in microorganisms, Trends Food Sci. Technol., 2018, 72, 13–24 CrossRef CAS.
- Y. Yan, Y. Peng, J. Tang, J. Mi, L. Lu, X. Li, L. Ran, X. Zeng and Y. Cao, Effects of anthocyanins from the fruit of Lycium ruthenicum Murray on intestinal microbiota, J. Funct. Foods, 2018, 48, 533–541 CrossRef CAS.
- Z. Liu, L. Han, X. Wang, Q. Feng and T. M. Gill, Disability Prior to Death Among the Oldest-Old in China., J. Gerontol., Ser. A, 2018, 73, 1701–1707 CrossRef PubMed.
- A. C. Milanetto, E. Nordenström, A. Sundlöv and M. Almquist, Health-Related Quality of Life After Surgery for Small Intestinal Neuroendocrine Tumours, World J. Surg., 2018, 42, 3231–3239 CrossRef PubMed.
- Y. Yang, X. Yuan, Y. Xu and Z. Yu, Purification of Anthocyanins from Extracts of Red Raspberry Using Macroporous Resin, Int. J. Food Prop., 2015, 18, 1046–1058 CrossRef CAS.
- P. M. Miranda, G. De Palma, V. Serkis, J. Lu, M. P. Louis-Auguste, J. L. McCarville, E. F. Verdu, S. M. Collins and P. Bercik, High salt diet exacerbates colitis in mice by decreasing Lactobacillus levels and butyrate production, Microbiome, 2018, 6, 57–74 CrossRef PubMed.
- B. Fotschki, J. Juśkiewicz, A. Jurgoński, K. Kołodziejczyk, J. Milala, M. Kosmala and Z. Zduńczyk, Anthocyanins in Strawberry Polyphenolic Extract Enhance the Beneficial Effects of Diets with Fructooligosaccharides in the Rat Cecal Environment, PLoS One, 2016, 11, e149081 CrossRef.
- H. Zeng, C. Huang, S. Lin, M. Zheng, C. Chen, B. Zheng and Y. Zhang, Lotus Seed Resistant Starch Regulates Gut Microbiota and Increases Short-Chain Fatty Acids Production and Mineral Absorption in Mice, J. Agric. Food Chem., 2017, 65, 9217–9225 CrossRef CAS PubMed.
- J. Juśkiewicz, Z. Zduńczyk, E. Żary-Sikorska, B. Król, J. Milala and A. Jurgoński, Effect of the dietary polyphenolic fraction of chicory root, peel, seed and leaf extracts on caecal fermentation and blood parameters in rats fed diets containing prebiotic fructans, Br. J. Nutr., 2011, 105, 710–720 CrossRef PubMed.
- Z. Haining and M. Yongkun, Optimisation of High Hydrostatic Pressure Assisted Extraction of Anthocyanins from Rabbiteye Blueberry Pomace, Czech J. Food Sci., 2017, 35, 180–187 CrossRef.
- C. Chang, C. Lin, C. Lu, J. Martel, Y. Ko, D. M. Ojcius, S. Tseng, T. Wu, Y. M. Chen, J. D. Young and H. Lai, Ganoderma lucidum reduces obesity in mice by modulating the composition of the gut microbiota (vol 6, 7489, 2015), Nat. Commun., 2017, 8, 1–17 CrossRef CAS PubMed.
- J. Chen, B. Kang, Q. Jiang, M. Han, Y. Zhao, L. Long, C. Fu and K. Yao, Alpha-Ketoglutarate in Low-Protein Diets for Growing Pigs: Effects on Cecal Microbial Communities and Parameters of Microbial Metabolism, Front. Microbiol., 2018, 9, 1–14 CrossRef PubMed.
- L. Zhao, Q. Zhang, W. Ma, F. Tian, H. Shen and M. Zhou, A combination of quercetin and resveratrol reduces obesity in high-fat diet-fed rats by modulation of gut microbiota, Food Funct., 2017, 8, 4644–4656 RSC.
- K. Lee, H. S. Lillehoj and G. R. Siragusa, Direct-Fed Microbials and Their Impact on the Intestinal Microflora and Immune System of Chickens, J. Poultry Sci., 2010, 47, 106–114 CrossRef.
- Z. Zhang, X. Peng, N. Zhang, L. Liu, Y. Wang and S. Ou, Cytotoxicity comparison of quercetin and its metabolites from in vitro fermentation of several gut bacteria, Food Funct., 2014, 5, 2152–2157 RSC.
- L. Zhao, F. Zhang, X. Ding, G. Wu, Y. Y. Lam, X. Wang, H. Fu, X. Xue, C. Lu, J. Ma, L. Yu, C. Xu, Z. Ren, Y. Xu, S. Xu, H. Shen, X. Zhu, Y. Shi, Q. Shen, W. Dong, R. Liu, Y. Ling, Y. Zeng, X. Wang, Q. Zhang, J. Wang, L. Wang, Y. Wu, B. Zeng, H. Wei, M. Zhang, Y. Peng and C. Zhang, Gut bacteria selectively promoted by dietary fibers alleviate type 2 diabetes, Science, 2018, 359, 1151–1156 CrossRef CAS PubMed.
- D. A. Winer, H. Luck, S. Tsai and S. Winer, The Intestinal Immune System in Obesity and Insulin Resistance, Cell Metab., 2016, 23, 413–426 CrossRef.
- A. Wallis, M. Ball, S. McKechnie, H. Butt, D. P. Lewis and D. Bruck, Examining clinical similarities between myalgic encephalomyelitis/chronic fatigue syndrome and D-lactic acidosis: a systematic review, J. Transl. Med., 2017, 15, 129–150 CrossRef PubMed.
- Y. Xu, C. Gao, H. Guo, W. Zhang, W. Huang, S. Tang, W. Gan, Y. Xu, H. Zhou and Q. Zhu, Sodium butyrate supplementation ameliorates diabetic inflammation in db/db mice, J. Endocrinol., 2018, 238, 231–244 CAS.
|
This journal is © The Royal Society of Chemistry 2019 |
Click here to see how this site uses Cookies. View our privacy policy here.