COMBI, continuous ozonation merged with biofiltration to study oxidative and microbial transformation of trace organic contaminants†
Received
19th November 2018
, Accepted 17th January 2019
First published on 17th January 2019
Abstract
Investigating the biodegradation of ozonation products of trace organic contaminants is important to further elucidate their fate and to assess the efficiency of advanced water treatment processes. In this study, a continuous ozonation merged with biofiltration (COMBI) laboratory system based on an electrochemical ozone generation method was developed. The system can be operated continuously and resource-efficiently over several months by supplying ozone doses typically used for water treatment and providing stable conditions for the establishment of microbial communities in biofiltration columns. Five trace organic contaminants, acesulfame, carbamazepine, diclofenac, dimethylsulfamide and fluoxetine, were investigated under drinking water and secondary treated wastewater ozonation conditions. After an equilibration time of three weeks, biodegradable ozonation products, for example N-nitrosodimethylamine (NDMA) and an acesulfame product, were removed in the filtration columns. Recalcitrant oxidation products such as trifluoroacetic acid (TFA) and two products of diclofenac either passed through the columns at unchanged concentration or were removed to a minor extent. The formation of a secondary biotransformation product from carbamazepine ozonation products could be also observed. In summary, the results show that the developed system is a valuable tool to investigate complex transformation processes of ozonation products during biofiltration. COMBI will simplify future ozonation-biotransformation studies and enable more comprehensive investigations with a wider range of contaminants under different conditions.
Water impact
A continuously operating ozonation biofiltration system was developed and tested in a proof-of-concept study by following the fate of ozonation products of five exemplary trace organic contaminants during both a drinking water and a wastewater effluent ozonation scenario. The resourceful and flexible lab-scale system will lead to a better understanding of complex contaminant transformation processes during advanced water treatment schemes.
|
Introduction
Trace organic contaminants (TrOCs) are a diverse class of organic compounds comprising pharmaceuticals, personal care products, hormones, pesticides and specialty chemicals that are frequently present at nanogram to microgram per liter concentrations in surface water, ground water and drinking water.1–4 The main entry pathways for TrOCs into water bodies are direct sources from agriculture, aquaculture and urban stormwater runoff,5,6 and indirectly through wastewater treatment plants.7–9 The occurrence of TrOCs in the aquatic environment poses a threat to various sensitive organisms10,11 and may adversely affect whole ecosystems.12 Furthermore, the detection of TrOCs in drinking water13,14 has raised public concerns.15,16 In 2015 the European Commission published a first watch list of emerging water contaminants with the aim to create a reliable information base on the occurrence of selected substances across the EU.17 As a consequence, more stringent measures to reduce concentrations of TrOCs in water bodies can be expected, including the widespread application of advanced water treatment approaches.
Ozone is a traditional drinking water disinfectant18 and ozonation is among the most promising technologies to degrade TrOCs during advanced wastewater treatment, water recycling and for drinking water production.19–22 Ozone attacks electron rich moieties of organic substrates such as double bonds, tertiary amines, organosulfur compounds and activated aromatic systems.23 Secondary oxidants derived from ozone decomposition, in particular hydroxyl radicals, react less selectively mainly by hydroxylation, hydrogen abstraction and electron transfer.24 At ozone doses typically applied for water treatment, primary and secondary oxidation reactions do not lead to significant mineralization but generate biodegradable assimilable organic carbon25–28 and transformation products of TrOCs.29 Some products are recalcitrant to further degradation.30 Ozonation is usually combined with a biofiltration step such as sand filtration to remove biodegradable organic carbon and to further break down transformation products.31 Ozonation can be also applied prior to natural engineered water treatment, including constructed wetlands, soil aquifer treatment and riverbank filtration.32
Biofiltration and post-ozonation engineered natural treatment stages contribute to reducing ecotoxicity indicators of the treated water, which in some cases have been found to increase after ozonation,30,33 depending on treatment conditions.34 Therefore, the combined effect of ozonation and subsequent biofiltration leads to significant reduction of the ecotoxicity of the treated water.35–40 The degradation of TrOCs during biofiltration depends on several factors, such as contaminant concentration,41 retention time,42,43 age, diversity and adaptation of the microbial community,44,45 substrate availability and composition for microbial metabolic processes,46,47 redox conditions,48,49 and temperature.50 Similar relationships during biofiltration can be expected for the removal of transformation products. However, extended studies are needed to further understand the fate of transformation products during biofiltration and to optimize removal efficiency under different conditions. A recent review concluded that the biodegradability of ozonation products of TrOCs depends on the reactive site of the target contaminant and on its reaction mechanism with ozone.51 Although ozonation products of numerous TrOCs have been identified, there are currently only a limited number of studies that investigate the biodegradability of ozonation products such as N-oxides.52–54
In the lab, ozonation of a water sample can be readily performed, while biological treatment processes following ozonation must be continuous to provide a stable and adapted microbiological community. The available studies have therefore employed batch ozonation followed by biofiltration or were carried out in pilot-scale and full-scale systems. These approaches have disadvantages because they are either laborious or require access to large infrastructure. An alternative is to perform batch biodegradation tests with ozonation products. However, the results of batch experiments might not be transferrable to continuous processes used in water and wastewater treatment. The kinetics in batch processes are different, the water matrix changes over time, and short-lifetime transformation products can only be studied through the online coupling of ozonation and biofiltration.
The goal of this study was to develop a cost-efficient continuously operating lab-scale system for the investigation of the ozonation of TrOCs and the fate of their ozonation and bio-transformation products during subsequent biological treatment steps. Two equivalent continuous ozonation systems with miniaturized electrochemical ozone generators followed by biologically active sand filtration columns were used, to test both a drinking water production scenario and a tertiary wastewater treatment scenario, which are two of the main applications of this treatment scheme. The selection of the target TrOCs was based on their diverse physicochemical properties and their relevance for drinking water (dimethylsulfamide, a pesticide metabolite, and acesulfame, an artificial sweetener), and wastewater (the pharmaceuticals carbamazepine, diclofenac, and fluoxetine). Through the analysis of literature-known transformation products the results could be compared with the ones from full-scale treatment plants and the capability of the COMBI setup could be proven.
Methods
Chemicals
All chemicals, including solvents, analytical consumables, TrOCs and ingredients for the preparation of synthetic wastewater were purchased from commercial sources. A list for TrOCs and analytical standards is provided in the ESI† (Text S2.1), including a table of molecular and structural data of parent compounds and their investigated ozonation products (Table S2†). Aqueous stock solutions were prepared from ultrapure water (resistivity >18 MΩ cm−1) from Milli-Q (Merck) or ELGA (Veolia) water purification systems. Synthetic wastewater (ESI,† Table S5) was prepared from tap water or deionized water according to OECD guidelines for synthetic sewage.55
Experimental setup
The initial small-scale column setup for studying continuous ozonation merged with biofiltration (COMBI) was designed and built at DVGW-Technologiezentrum Wasser, Germany (System 1). This setup was used to investigate dimethylsulfamide (DMS) and acesulfame (ACE) in a waterworks scenario. A similar setup was built at the University of Bath, UK (System 2) and used to investigate the fate of carbamazepine (CBZ), diclofenac (DF) and fluoxetine (FLX) in a wastewater effluent ozonation scenario.
A schematic of the setup is shown in Fig. 1. Photographs are shown in ESI,† Fig. S1 and a summary of costs for parts is listed in Table S1.† The setup consisted of an ozonation column and three post-ozonation filtration columns, feed and effluent storage tanks, a pump and an ozone generation vessel. An ozone micro-cell (Innovatec Gerätetechnik GmbH, Germany) was used to generate ozone by electrolysis of demineralized water. The cell consists of porous stainless-steel frits that are used as electrodes, which are contacted with an ion-conducting membrane (solid electrolyte of a polymer, <0.2 mm). The amount of ozone generated is determined by the number of electrolysis cells and the DC current applied. Head-space ozone, including oxygen and hydrogen as byproducts, flowed continuously via the intrinsic pressure of the electrochemical gas production through a tube connected to a sparger into the ozonation column. Water was delivered from the storage tank into the ozonation column using adjustable membrane pumps or gear pumps. The water was then gravity-fed from the ozonation column into the subsequent filtration columns.
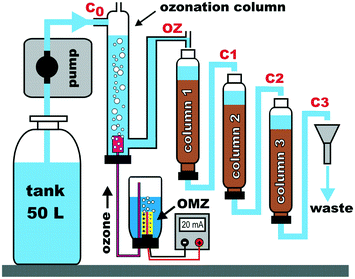 |
| Fig. 1 Schematic of the continuous small-scale ozonation/biofiltration setup. Sampling points are shown as C0, OZ, C1, C2 and C3. | |
Operational parameters
The operational parameters of both systems are summarized in Table 1. System 1 used anthracite (Everzit) as filtration medium for the first column C1, and sand from a drinking water treatment plant for columns C2 and C3. The sand had been used for several years in a sand filter after an ozone treatment, and was used in the COMBI columns without any cleaning. For System 2 water filtration sand (0.7 mm to 1.2 mm, 1.0 to 2.0 mm, Long Rake Spar, UK) was used as purchased. A 1 cm-layer of the coarser sand served as bottom support over a metal mesh in each column. System 2 was inoculated with secondary treated wastewater effluent, while System 1 was not specifically inoculated. Both systems had been operating continuously at room temperature in the presence of target trace contaminants for at least three weeks before sampling first occurred. The columns were covered with aluminum foil to prevent photolysis, and sand is a non-adsorptive filtration medium.
Table 1 Operational parameters
Parameter |
System 1 (Karlsruhe) |
System 2 (Bath) |
Ozone generation |
Ozone-microcell with 4 cell hearts (Innovatec) |
Voltage of microcell/V |
24 |
Current of microcell/mA |
10 to 200 |
Ozone output/(mg min−1) |
0.01 to 1 |
Pump |
Solenoid diaphragm pump (e.g. FMM 20, KNF) or gear pump (e.g. REGLO-Z digital, Ismatec) |
Flow rate used for long-term operation/(mL min−1) |
6 |
3 |
Diameter, length of the ozonation column/cm |
1.8, 17.5 |
2, 20 |
Volume of ozonation column/mL |
45 |
60 |
Diameter, length of each filtration column/cm |
6.5, 20 |
4, 30 |
Volume of each filtration column/mL |
660 |
375 |
Filtration medium/mm |
Everzit® N (C1) and sand from a water treatment plant (C2/C3) |
Quartz sand, 0.7 to 1.2/1.0 to 2.0 (Long Rake Spar) |
Water type |
Drinking water |
Synthetic wastewater |
Water characteristics |
pH 7.2, conductivity 610 μS cm−1, TOC ∼ 0.9 mg L−1, calcium carbonate hardness 3.2 mmol L−1 |
pH 7.4, conductivity 800 μS cm−1, TOC ∼ 7 mg L−1, TN ∼ 7.5 mg L−1 |
Target contaminants |
Dimethylsulfamide (DMS), acesulfame (ACE) |
Carbamazepine (CBZ), diclofenac (DF), fluoxetine (FLX) |
The drinking water used for operating System 1 was obtained from groundwater, which is only treated by aeration. In a single combined experiment, 100 L of feed water were spiked with the target compounds (DMS = 16 nmol L−1 and ACE = 0.6 μmol L−1 to 1 μmol L−1), and refilled weekly. Due to the persistence and high solubility of both ACE and DMS in water, no removal by degradation or significant adsorption to the feed tank was observed. Samples were collected on days 7, 24 and 97 for DMS and 24, 27 and 93 for ACE.
The synthetic wastewater for System 2 was prepared freshly three times a week according to OECD guidelines for synthetic sewage55 at 10-fold dilution to yield an initial total organic carbon concentration of 10 mg L−1 (ESI,† Table S5). The easily biodegradable organic matter contained in this mixture led to biofilm growth and occasional clogging of the first column, which was resolved by scraping or manually removing the upper sand layer. The TrOCs CBZ, DF and FLX were spiked simultaneously into the influent tank (a range of 10 L to 15 L of synthetic wastewater) at a concentration of 1 μmol L−1 to 3 μmol L−1 two weeks after continuous operation had started, to allow time for a microbial community to grow. The measured concentration in the influent tank fluctuated slightly due to the relatively large volume prepared for each refill, and sorption or slow microbial decomposition occurring in the tank. Samples were collected on days 22, 28, 42 and 54, where day 1 is the first day when trace contaminants were spiked. All samples were collected and analyzed in duplicate.
To enable detection of transformation products without pre-concentration, spiked levels of ACE, CBZ, DF and FLX were higher than those typically found in wastewater effluent.7 The microbial characterization of the sand columns was not the scope of this study, while known transformation pathways were consulted to interpret results.
Analysis
A description of analytical methods for all target compounds and their transformation products is provided in ESI† Section S2.2. Briefly, ultra high performance liquid chromatography-mass spectrometry (UHPLC-MS) analysis for CBZ, DF and FLX was performed with a Thermo Scientific Dionex UltiMate 3000 system coupled to a Bruker Daltonics maXis HD electrospray ionization quadrupole time-of-flight (ESI-QTOF) mass spectrometer. Transformation products of CBZ and DF were identified based on literature data, mass accuracy, consistent retention time and MS/MS analysis in MRM (multiple reaction monitoring) mode. Fragmentation patterns are provided in ESI,† Section S3.3. Direct injection was used for the analysis of trifluoroacetic acid (TFA), ACE and its ozonation product OP168. DMS and N-nitrosodimethylamine (NDMA) samples were pre-concentrated with solid phase extraction (SPE) prior the analysis.56 Analysis was performed on an API 5500 Q-Trap triple quadrupole mass spectrometer (Applied Biosystems/MDS Sciex Instruments, Concord, ON, Canada). TFA analysis was performed using ion exchange liquid chromatography-electrospray tandem mass spectrometry (LC-ESI-MS/MS) according to a recently developed method.57 GC analysis for NDMA was carried out with a series 6890 gas chromatograph connected to a MSD 5973 inert mass spectrometer (both Agilent, Waldbronn, Germany).
UV/vis absorption for the determination of dissolved ozone in water with the indigo method,58 and for tracer tests with fluorescein to determine hydraulic residence times (HRTs), were conducted with stationary devices (e.g. Cary 100, Agilent; FP 8200, Jasco; EVO300, Thermo Scientific) or a self-built portable LED photometer. In System 1, the dissolved ozone concentration was measured in pure water (no reactions present) by sampling the water inside the ozonation column. In System 2, the ozone dose was measured by feeding an indigo solution through the ozonation column, which captured directly the ozone transferred. More details are provided in the ESI,† Section S2.3.
Results and discussion
Determination of operational range
Initial tests determined ozone contact time and HRTs. Fluorescein breakthrough curves for both systems are shown in Fig. 2A and B. Further details are provided in ESI† Section S3.1. The HRT was assumed to be equal to the time of maximum (complete) tracer breakthrough. At a flow rate of 6 mL min−1, the ozonation contact time in System 1 was 30 min and the total HRT was approximately 5 h. For System 2 the ozonation contact time at a flow rate of 3 mL min−1 was 10 min and the HRT was approximately 4 h. A wide range of operational parameters can be achieved by varying the flow rate. For instance, in System 2 a change of flow rate from 2 mL min−1 to 12 mL min−1, results in the single column HRT changing from 150 min to 15 min (Fig. S7†), with the total HRT decreasing from approximately 8 h to 1 h.
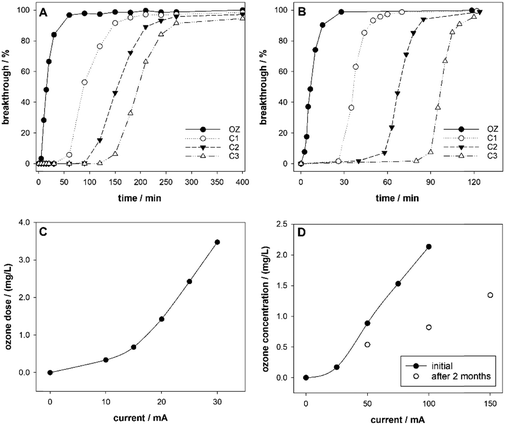 |
| Fig. 2 Fluorescein breakthrough curves for A) System 1 (flow rate of 6 mL min−1 and nitrogen flowing in the ozonation column), and B) System 2 (flow rate of 5 mL min−1, without substitute gas sparging through in the ozonation column). Ozone dose or concentration depending on the current intensity at constant flow rates of C) 6 mL min−1 in System 1, and D) 3 mL min−1 in System 2. | |
The relationship of the applied electrical current of the electrochemical cell and the ozone dose is presented in Fig. 2C. The change in ozone concentration for a single cell over time is shown in Fig. 2D. The decreasing efficiency of ozone production is due to aging of the ozone micro-cells. The difference between the two systems can be attributed to design differences, such as the length of the tubing connecting the microcell vessel and the ozonation column, the height and volume of the ozonation column, and the hydrostatic pressure which must be overcome by the gas. To further characterize the mass transfer of ozone in the system, analysis of the ozone concentration in the inlet gas and the off-gas would need to be conducted.
Long-term experiments were conducted at conditions similar to those of other ozonation–biofiltration systems (ozone dose of 1 mg L−1 to 10 mg L−1, ozonation HRT of 30 min or less, filtration HRT of 10 min to 30 min)21,52 without further optimization of the operational parameters. A longer filtration HRT was chosen to elucidate the fate of compounds that are not easily biodegradable.
Removal and transformation of trace contaminants in a drinking water treatment scenario
Dimethylsulfamide.
The oxidative transformation of DMS to NDMA during ozonation was examined as a first example. Fig. 3 shows the evolution of DMS and NDMA in the COMBI system at three sampling events during three months of continuous operation. The reactivity of DMS with ozone is important for waterworks as both DMS sorption and biological degradation during riverbank filtration are limited, while filtration over activated carbon, sand filtration, disinfection by chlorine and nanofiltration cannot completely remove DMS if present in raw waters.59 Oxidative treatment followed by a biological treatment step seems to be one of the very few promising treatment combinations for waterworks to remove DMS.59 DMS was almost completely oxidized (to below 0.2 nmol L−1, corresponding to at least 99% removal) under the applied conditions (ozone dose approx. 3 mg L−1, contact time 30 min). The reaction of DMS with ozone is slow (rate constant of 20 M−1 s−1) and leads to the formation of NDMA in the presence of bromide.60 The maximum NDMA yield is reached for bromide levels of 15 μg L−1 to 20 μg L−1 which are typical for drinking waters.60 The bromide level of the used tap water was about 35 μg L−1. During the four month experiment, the NDMA formation was reproducible, with an average molar yield of NDMA of approximately 50%. In full-scale waterworks similar DMS transformation rates of 73% to 100% were observed, while DMS to NDMA conversion rates were between 30% and 50% for spiked drinking water.59
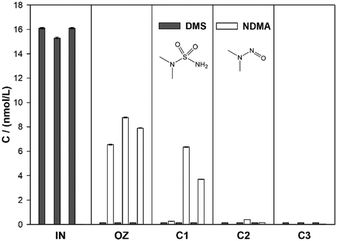 |
| Fig. 3 Conversion of DMS to NDMA by ozonation in drinking water matrix and subsequent degradation in biologically active sand columns in the COMBI set-up. The samples were taken on days 7, 24 and 97. | |
Only traces of NDMA were detected after the water had passed column 2, while NDMA was absent (below 0.03 nmol L−1) in the effluent of column 3 (total HRT of approximately 5 h). NDMA has been shown to be biodegradable in sand filtration59 and managed aquifer recharge.61 The high removal observed in this study demonstrates the presence of a well-developed microbial community in the sand columns. Overall, both DMS and NDMA concentrations were below the detection limit in the final effluent of the system.
Acesulfame (ACE).
The transformation of ACE to OP168 by ozone and its subsequent fate were also examined (Fig. 4). ACE reacts with ozone with a rate constant of 88 M−1 s−1,62 according to the Criegee mechanism, leading to ozonation products such as ACE OP170 and to a minor extent ACE OP168.63 ACE was almost completely removed (at least 97% removal) under the applied conditions (ozone dose approx. 3 mg L−1, contact time 30 min). OP168 was chosen for further investigation. As the ozonation products of ACE can be further oxidized, the yield at the effluent of the ozonation column (approximately 50% on the first two sampling days) may represent only a fraction of the initially formed OP168. However, the yield on the last sampling day was almost 100%.
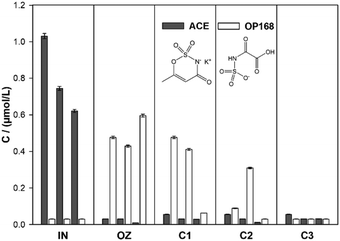 |
| Fig. 4 Conversion of ACE to OP168 in drinking water matrix by ozonation and subsequent degradation in biologically active sand columns in the COMBI set-up. The samples were taken on days 24, 27 and 93. | |
No further removal of unreacted residual ACE during column passage occurred. ACE was recently reported to be biodegradable during activated sludge treatment64,65 but has also been shown to persist in wastewater treatment, including riverbank filtration.66,67 No biodegradation occurred over several months of operation and we suggest that the necessary biological community was absent. Breakthrough of OP168 through columns 1 and 2 was observed during the first two sampling events, but OP168 was not detected in the effluent of column 3 (concentration below 0.03 μmol L−1). This indicates that OP168 is biodegradable. Overall, removal of OP168 was highest at the last sampling date, which could be due to the maturation of the microbial community leading to an improved ability to degrade the transformation product. The structurally related compound ACE OP170 can be removed with activated carbon filtration, likely as a result of biodegradation.63 The fate of ACE OP168 in sand filtration has not been investigated before to the knowledge of the authors.
Removal and transformation of trace contaminants in a wastewater effluent ozonation scenario
Carbamazepine (CBZ).
At ozone concentrations of 1 mg L−1 to 2 mg L−1 and a contact time of 10 min in the ozonation column more than 99% of CBZ (C0 = 2.5 μmol L−1 ± 0.2 μmol L−1) was removed (final concentration below 0.03 μmol L−1). CBZ reacts with ozone at the double bond of its heterocyclic centre with a rate constant equal to 3 × 105 M−1 s−1.68 The main ozonation product is BQM (1-(2-benzaldehyde)-4-hydro-(1H,3H)-quinazoline-2-one).69 Minor ozonation products are BaQD (1-(2-benzoic acid)-(1H,3H)-quinazoline-2,4-one), BQD (1-(2-benzaldehyde)-(1H,3H)-quinazoline-2,4-one)69 and BaQM (1-(2-benzoic acid)-4-hydro-(1H,3H)-quinazoline-2-one).70
Fig. 5 shows the evolution of the transformation products BQM and BaQD after ozonation at four sampling events during two months of continuous operation. Results are shown semi-quantitatively because analytical standards were not available. The variation in the formation of BQM and BaQD during ozonation on the four sampling days is shown in the ESI,† Fig. S9. General trends were consistent over the observation period despite fluctuations in the concentration of BQM and BaQD after the filtration column passage. BQM concentrations decreased continuously during passage through the filtration columns, in agreement with a previous study.70 BQM removal occurred predominantly in the first column, while consecutive columns had modest additional effect. The high rate of BQM removal in the first column can be ascribed to an increased biological activity in the first few centimetres of the filter sand. The biological activity is slightly enhanced by additional oxygen following the decomposition of ozone,32,71 and also by the higher availability of biodegradable TOC after ozonation. Although the redox conditions were not measured, oxygen concentrations slightly above atmospheric equilibrium can be expected at the top of the first column. The increased biological activity in the first column was also evident by biofilm formation and occasional clogging during operation.
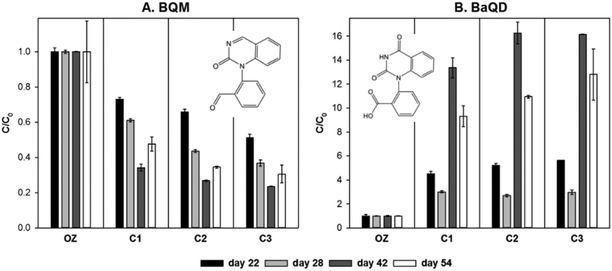 |
| Fig. 5 Evolution of carbamazepine transformation products BQM (A) and BaQD (B) during passage through the sand columns on four different days. The ratio C/C0 was calculated by dividing each signal (peak area of target compound/peak area of internal standard) by the average signal after ozonation. | |
Overall removal of BQM during column passage was between 50% and 75%, which is high considering the HRT of 4 hours and shows that BQM is readily biodegradable, in contrast to its parent compound CBZ. Improved BQM removal towards later sampling dates could be due to the adaptation of the microbial community.72 Removal by adsorption was considered negligible, since the system was equilibrated for 3 weeks before sampling occurred and sand is a non-adsorptive filtration medium. An adsorption experiment with the parent compound CBZ showed no retardation in comparison to the tracer fluorescein or loss due to abiotic processes (Fig. S8†). In addition, the ozonation products of CBZ have been shown to be less adsorptive to activated carbon than the parent compound.73
Toxicity studies suggest that increased chromosomal damage of test organisms induced by ozonated CBZ solutions can be partially attributed to the formation of BQM.74 The results presented here indicate that BQM is readily biodegradable and unlikely to persist in surface water or groundwater.
BaQD concentration increased or remained unchanged during passage through the filtration columns. Higher BaQD formation roughly corresponded with higher removal of BQM, indicating that BaQD was microbially generated from BQM and other ozonation products of CBZ. BaQD can be formed directly by ozonation or by consecutive microbial transformation of ozonation products of CBZ and structurally similar compounds.70,75 BaQD has been found to be slowly biodegradable and persistent in sand filtration experiments with an HRT of 12 days.70 In a pilot scale wastewater treatment plant, partial removal of BaQD was achieved during GAC filtration but not during passage through a clay biofilter.76
BaQD has been detected in wastewater effluent, surface water, groundwater and drinking water21,75–77 and has potentially ecotoxicological relevance.78 The results of this study indicate that microbial transformation during biofiltration is a more important formation pathway of BaQD than ozonation itself. Monitoring BaQD in addition to BQM is important to fully understand the fate of CBZ during ozonation and subsequent treatment processes.
Diclofenac (DF).
Under the applied conditions (C0(DF) = 2.7 μmol L−1 ± 0.1 μmol L−1, β0(ozone) = 1 mg L−1 to 2 mg L−1, contact time = 10 min) DF was removed to more than 99% during ozonation (final concentration below 0.03 μmol L−1). DF has a high reaction rate constant with ozone (106 M−1 s−1), due to the presence of two aromatic amino groups that are deprotonated at neutral pH (pKa = 4).68 The main ozonation products of DF are DF-IQ (diclofenac-2,5-iminoquinone), OH-DF (5-hydroxydiclofenac) and 2,6-dichloroaniline, while other minor ozonation products have also been detected.79,80 Both DF-IQ and OH-DF have been found as microbial degradation products of DF in activated sludge.81 This study focussed on the fate of DF-IQ and OH-DF during column passage after ozonation. Other known DF ozonation products such as 2,6-dichloroaniline were either not detected or were only found in traces.
As shown in Fig. 6, both DF-IQ and OH-DF were persistent during column passage. A slightly decreasing trend was observed for DF-IQ, while for OH-IF a slightly increasing trend was found. Biological and abiotic processes might affect the equilibrium between these two compounds,82 while DF-IQ has also been shown to adsorb on sediment.83 However, experiments with higher initial concentrations of DF would be required to yield sufficient amounts of DF-IQ and OH-DF to investigate subtle concentration changes. In ozonation experiments with DF in deionized water, a maximum yield of 2.7% for DF-IQ and 4.5% for OH-IF on a molar basis was found, respectively.79
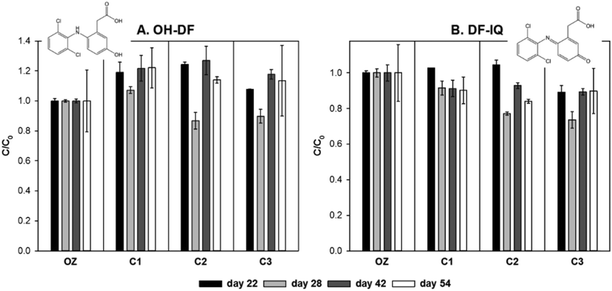 |
| Fig. 6 Evolution of diclofenac transformation products OH-DF (A) and DF-IQ (B) during passage through the sand columns on four different days. The ratio C/C0 was calculated by dividing each signal (peak area of target compound/peak area of internal standard) by the average signal after ozonation. | |
The observed persistence of ozonation products of DF is in agreement with experiments in moving bed biofilm reactors (MBBRs), where the removal of DF-IQ reached 37% and that of OH-DF 27% after incubation for 150 h.84 Therefore, a longer filtration residence time might be necessary for the degradation of DF-IQ and OH-DF. The results show that sand filtration which is commonly employed after ozonation might not be a sufficient barrier to remove the main ozonation products of diclofenac.
Fluoxetine (FLX).
FLX was chosen for investigation because it has recently been identified as a precursor of TFA in wastewater and drinking water treatment processes.57 The removal of FLX during ozonation at a concentration of C0(FLX) = 1.2 ± 0.1 μmol L−1 and β0(ozone) = 1 mg L−1 to 2 mg L−1, a contact time of 10 minutes and a pH of 7.5 was 70% to 95% (Fig. 7). The ozonation rate constant of FLX is pH dependent, due to the presence of an amine moiety which is deprotonated at higher pH (pKa = 10) and therefore more reactive. Several ozonation products of fluoxetine are known.33 TFA was targeted as a major ozonation product of fluoxetine. Other known transformation products of FLX were either not detected or only found at trace levels. The formation of TFA during ozonation varied from 8% to 26% on a molar base. Despite this variation, higher TFA formation correlated with higher FLX removal (Fig. 7).
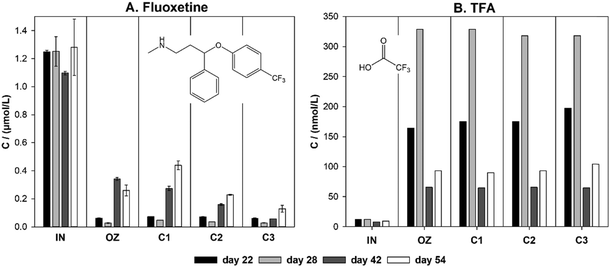 |
| Fig. 7 Evolution of fluoxetine and TFA during passage through the sand columns. Error bars for fluoxetine refer to the standard deviation of duplicate samples. For TFA, one sample was analysed for each sampling point on each day. | |
A small amount of TFA (approximately 10 nmol L−1) was present in the influent, likely due to the presence of TFA in the tap water that was used to prepare the synthetic wastewater. A similar amount was formed due to the ozonation of other matrix components, based on the analysis of samples that were not spiked with FLX. The formation of TFA is likely mostly due to reactions mediated by OH-radicals, rather than direct reaction with ozone, considering the electron-withdrawing effect of the trifluoromethyl substituent of the aromatic ring.
Little to no removal of unreacted FLX was observed during passage through the sand columns. Minor changes in the concentration of FLX during its passage through sand filters might be due to ionic interactions with silica sand,85 since the silica surface is negatively charged at circumneutral pH,86 while FLX is a positively charged amine. The concentration of TFA was stable during passage through the sand filters. Evidence supporting both the persistence87,88 and the biodegradability of TFA89,90 can be found in the literature. In general, microbial defluorination is difficult to occur due to the low reduction potential of the C–F bond.91 Results are in agreement with a recent study, where no removal of TFA was observed at three different waterworks that used filtration over biologically active or adsorptive media.57 Overall, TFA that is formed during ozonation of fluoxetine will likely persist during subsequent sand filtration.
Conclusions
A continuously operating laboratory system (COMBI) was developed to investigate the ozonation of TrOCs in water coupled with subsequent biologically active sand filtration. The system was used for both a drinking water treatment scenario and an advanced wastewater treatment scenario for five selected TrOCs and included fate analysis of ozonation products. After three weeks of operation, microbial degradation processes occurred in the filtration columns, while removal further increased over time. The microbial community is expected to be different in the two systems, as a result of the different filtration media and substrate compositions, although this was not further examined in this study.
Moderate to high removal was observed for the main ozonation product of carbamazepine, an ozonation product of acesulfame, as well as for NDMA, produced via ozonation through its precursor DMS. On the other hand, an ozonation product of carbamazepine, two ozonation products of diclofenac, and TFA from ozonation of fluoxetine persisted microbial degradation. Good agreement with the results of large-scale and pilot-scale studies was found,21,57,59 implying that the developed experimental setup can offer reliable predictions.
The developed system is a useful tool to provide predictions on the fate of ozonation products for different treatment conditions and process configurations. The COMBI system has a small footprint, while the total cost of parts for a complete system is approximately 660 € (ESI,† Table S1). Based on these attributes COMBI will simplify studies on ozonation–biofiltration, ultimately leading to a better understanding of complex contaminant transformation processes during advanced water treatment schemes.
Conflicts of interest
There are no conflicts to declare.
Acknowledgements
We would like to acknowledge Paula Brendel and Beat Schmutz for assistance in the lab and Shaun Reeksting for assistance with analysis. GAZ was supported by a University of Bath research scholarship and an EPSRC funded integrated PhD studentship in Sustainable Chemical Technologies (EP/L016354/1). Start-up infrastructure funding by the Faculty of Engineering & Design for JW research group is appreciated. This research was supported by a Royal Society equipment grant (RG2016-150544): Clean water technologies – Low-dosed oxidants to improve low-energy natural engineered water treatment systems.
Notes and references
- C. J. Houtman, Emerging contaminants in surface waters and their relevance for the production of drinking water in Europe, J. Integr. Environ. Sci., 2010, 7, 271–295 CrossRef.
- S. R. Hughes, P. Kay and L. E. Brown, Global synthesis and critical evaluation of pharmaceutical data sets collected from river systems, Environ. Sci. Technol., 2013, 47, 661–677 CrossRef CAS PubMed.
- M. Stuart, D. Lapworth, E. Crane and A. Hart, Review of risk from potential emerging contaminants in UK groundwater, Sci. Total Environ., 2012, 416, 1–21 CrossRef CAS PubMed.
- M. S. Fram and K. Belitz, Occurrence and concentrations of pharmaceutical compounds in groundwater used for public drinking-water supply in California, Sci. Total Environ., 2011, 409, 3409–3417 CrossRef CAS PubMed.
- A. Pal, K. Y. Gin, A. Y. Lin and M. Reinhard, Impacts of emerging organic contaminants on freshwater resources: review of recent occurrences, sources, fate and effects, Sci. Total Environ., 2010, 408, 6062–6069 CrossRef CAS PubMed.
- D. J. Lapworth, N. Baran, M. E. Stuart and R. S. Ward, Emerging organic contaminants in groundwater: A review of sources, fate and occurrence, Environ. Pollut., 2012, 163, 287–303 CrossRef CAS PubMed.
- B. Petrie, J. Youdan, R. Barden and B. Kasprzyk-Hordern, Multi-residue analysis of 90 emerging contaminants in liquid and solid environmental matrices by ultra-high-performance liquid chromatography tandem mass spectrometry, J. Chromatogr. A, 2016, 1431, 64–78 CrossRef CAS PubMed.
- P. Schröder, B. Helmreich, B. Škrbić, M. Carballa, M. Papa, C. Pastore, Z. Emre, A. Oehmen, A. Langenhoff, M. Molinos, J. Dvarioniene, C. Huber, K. P. Tsagarakis, E. Martinez-Lopez, S. M. Pagano, C. Vogelsang and G. Mascolo, Status of hormones and painkillers in wastewater effluents across several European states—considerations for the EU watch list concerning estradiols and diclofenac, Environ. Sci. Pollut. Res., 2016, 23, 12835–12866 CrossRef PubMed.
- N. H. Tran, M. Reinhard and K. Y.-H. Gin, Occurrence and fate of emerging contaminants in municipal wastewater treatment plants from different geographical regions-a review, Water Res., 2018, 133, 182–207 CrossRef CAS PubMed.
- W. Sanchez, W. Sremski, B. Piccini, O. Palluel, E. Maillot-Marechal, S. Betoulle, A. Jaffal, S. Ait-Aissa, F. Brion, E. Thybaud, N. Hinfray and J. M. Porcher, Adverse effects in wild fish living downstream from pharmaceutical manufacture discharges, Environ. Int., 2011, 37, 1342–1348 CrossRef CAS PubMed.
- T. Brodin, S. Piovano, J. Fick, J. Klaminder, M. Heynen and M. Jonsson, Ecological effects of pharmaceuticals in aquatic systems—impacts through behavioural alterations, Philos. Trans. R. Soc., B, 2014, 369, 1–10 CrossRef PubMed.
- S. González, R. López-Roldán and J.-L. Cortina, Presence and biological effects of emerging contaminants in Llobregat River basin: A review, Environ. Pollut., 2012, 161, 83–92 CrossRef PubMed.
- K. L. Klarich, N. C. Pflug, E. M. DeWald, M. L. Hladik, D. W. Kolpin, D. M. Cwiertny and G. H. LeFevre, Occurrence of Neonicotinoid Insecticides in Finished Drinking Water and Fate during Drinking Water Treatment, Environ. Sci. Technol. Lett., 2017, 4, 168–173 CrossRef CAS.
- M. Huerta-Fontela, M. T. Galceran and F. Ventura, Occurrence and removal of pharmaceuticals and hormones through drinking water treatment, Water Res., 2011, 45, 1432–1442 CrossRef CAS PubMed.
- M. Schriks, M. B. Heringa, M. M. E. van der Kooi, P. de Voogt and A. P. van Wezel, Toxicological relevance of emerging contaminants for drinking water quality, Water Res., 2010, 44, 461–476 CrossRef CAS PubMed.
- V. de Jesus Gaffney, C. M. M. Almeida, A. Rodrigues, E. Ferreira, M. J. Benoliel and V. V. Cardoso, Occurrence of pharmaceuticals in a water supply system and related human health risk assessment, Water Res., 2015, 72, 199–208 CrossRef CAS PubMed.
-
European Commission, Implementing decision (EU) 2015/495 of 20 March 2015 establishing a watch list of substances for Union-wide monitoring in the field of water policy pursuant to Directive 2008/105/EC of the European Parliament and of the Council, 2015.
-
C. Gottschalk, J. A. Libra and A. Saupe, Ozonation of water and waste water: A practical guide to understanding ozone and its applications, John Wiley & Sons, 2009 Search PubMed.
- R. I. L. Eggen, J. Hollender, A. Joss, M. Schärer and C. Stamm, Reducing the Discharge of Micropollutants in the Aquatic Environment: The Benefits of Upgrading Wastewater Treatment Plants, Environ. Sci. Technol., 2014, 48, 7683–7689 CrossRef CAS PubMed.
- J. Hollender, S. G. Zimmermann, S. Koepke, M. Krauss, C. S. McArdell, C. Ort, H. Singer, U. Von Gunten and H. Siegrist, Elimination of organic micropollutants in a municipal wastewater treatment plant upgraded with a full-scale post-ozonation followed by sand filtration, Environ. Sci. Technol., 2009, 43, 7862–7869 CrossRef CAS PubMed.
- M. Bourgin, B. Beck, M. Boehler, E. Borowska, J. Fleiner, E. Salhi, R. Teichler, U. von Gunten, H. Siegrist and C. S. McArdell, Evaluation of a full-scale wastewater treatment plant upgraded with ozonation and biological post-treatments: Abatement of micropollutants, formation of transformation products and oxidation by-products, Water Res., 2018, 129, 486–498 CrossRef CAS PubMed.
- J. Blackbeard, J. Lloyd, M. Magyar, J. Mieog, K. G. Linden and Y. Lester, Demonstrating organic contaminant removal in an ozone-based water reuse process at full scale, Environ. Sci.: Water Res. Technol., 2016, 2, 213–222 RSC.
-
C. Von Sonntag and U. Von Gunten, Chemistry of ozone in water and wastewater treatment, IWA publishing, 2012 Search PubMed.
-
C. von Sonntag, Free-radical-induced DNA damage and its repair, Springer, 2006 Search PubMed.
- M. K. Ramseier, A. Peter, J. Traber and U. von Gunten, Formation of assimilable organic carbon during oxidation of natural waters with ozone, chlorine dioxide, chlorine, permanganate, and ferrate, Water Res., 2011, 45, 2002–2010 CrossRef CAS PubMed.
- J. E. Drewes and M. Jekel, Behavior of DOC and AOX using advanced treated wastewater for groundwater recharge, Water Res., 1998, 32, 3125–3133 CrossRef CAS.
- A. A. Yavich, K. H. Lee, K. C. Chen, L. Pape and S. J. Masten, Evaluation of biodegradability of NOM after ozonation, Water Res., 2004, 38, 2839–2846 CrossRef CAS PubMed.
- J. Schumacher, Y. Z. Pi and M. Jekel, Ozonation of persistent DOC in municipal WWTP effluent for groundwater recharge, Water Sci. Technol., 2004, 49, 305–310 CrossRef CAS PubMed.
- S. Merel, S. Lege, J. E. Yanez Heras and C. Zwiener, Assessment of N-Oxide Formation during Wastewater Ozonation, Environ. Sci. Technol., 2017, 51, 410–417 CrossRef CAS PubMed.
- L. Schlüter-Vorberg, C. Prasse, T. A. Ternes, H. Mückter and A. Coors, Toxification by Transformation in Conventional and Advanced Wastewater Treatment: The Antiviral Drug Acyclovir, Environ. Sci. Technol. Lett., 2015, 2, 342–346 CrossRef.
- D. Gerrity, M. Arnold, E. Dickenson, D. Moser, J. D. Sackett and E. C. Wert, Microbial community characterization of ozone-biofiltration systems in drinking water and potable reuse applications, Water Res., 2018, 135, 207–219 CrossRef CAS PubMed.
- I. Zucker, H. Mamane, H. Cikurel, M. Jekel, U. Hübner and D. Avisar, A hybrid process of biofiltration of secondary effluent followed by ozonation and short soil aquifer treatment for water reuse, Water Res., 2015, 84, 315–322 CrossRef CAS PubMed.
- Y. Zhao, G. Yu, S. Chen, S. Zhang, B. Wang, J. Huang, S. Deng and Y. Wang, Ozonation of antidepressant fluoxetine and its metabolite product norfluoxetine: Kinetics, intermediates and toxicity, Chem. Eng. J., 2017, 316, 951–963 CrossRef CAS.
- M. Petala, P. Samaras, A. Zouboulis, A. Kungolos and G. P. Sakellaropoulos, Influence of ozonation on the in vitro mutagenic and toxic potential of secondary effluents, Water Res., 2008, 42, 4929–4940 CrossRef CAS PubMed.
- M. Bundschuh, R. Pierstorf, W. H. Schreiber and R. Schulz, Positive effects of wastewater ozonation displayed by in situ bioassays in the receiving stream, Environ. Sci. Technol., 2011, 45, 3774–3780 CrossRef CAS PubMed.
- M. Misik, S. Knasmueller, F. Ferk, M. Cichna-Markl, T. Grummt, H. Schaar and N. Kreuzinger, Impact of ozonation on the genotoxic activity of tertiary treated municipal wastewater, Water Res., 2011, 45, 3681–3691 CrossRef CAS PubMed.
- D. Altmann, H. Schaar, C. Bartel, D. L. Schorkopf, I. Miller, N. Kreuzinger, E. Mostl and B. Grillitsch, Impact of ozonation on ecotoxicity and endocrine activity of tertiary treated wastewater effluent, Water Res., 2012, 46, 3693–3702 CrossRef CAS PubMed.
- D. Stalter, A. Magdeburg, M. Weil, T. Knacker and J. Oehlmann, Toxication or detoxication? In vivo toxicity assessment of ozonation as advanced wastewater treatment with the rainbow trout, Water Res., 2010, 44, 439–448 CrossRef CAS PubMed.
- A. Magdeburg, D. Stalter and J. Oehlmann, Whole effluent toxicity assessment at a wastewater treatment plant upgraded with a full-scale post-ozonation using aquatic key species, Chemosphere, 2012, 88, 1008–1014 CrossRef CAS PubMed.
- Z. Yan, Y. Zhang, H. Yuan, Z. Tian and M. Yang, Fish larval deformity caused by aldehydes and unknown byproducts in ozonated effluents from municipal wastewater treatment systems, Water Res., 2014, 66, 423–429 CrossRef CAS PubMed.
- K. M. Onesios-Barry, D. Berry, J. B. Proescher, I. A. Sivakumar and E. J. Bouwer, Removal of pharmaceuticals and personal care products during water recycling: microbial community structure and effects of substrate concentration, Appl. Environ. Microbiol., 2014, 80, 2440–2450 CrossRef PubMed.
- L. Paredes, E. Fernandez-Fontaina, J. M. Lema, F. Omil and M. Carballa, Understanding the fate of organic micropollutants in sand and granular activated carbon biofiltration systems, Sci. Total Environ., 2016, 551–552, 640–648 CrossRef CAS PubMed.
- M. Escola Casas and K. Bester, Can those organic micro-pollutants that are recalcitrant in activated sludge treatment be removed from wastewater by biofilm reactors (slow sand filters)?, Sci. Total Environ., 2015, 506–507, 315–322 CrossRef CAS PubMed.
- T. L. Zearley and R. S. Summers, Removal of trace organic micropollutants by drinking water biological filters, Environ. Sci. Technol., 2012, 46, 9412–9419 CrossRef CAS PubMed.
- S. Kim, K. Rossmassler, C. D. Broeckling, S. Galloway, J. Prenni and S. K. De Long, Impact of inoculum sources on biotransformation of pharmaceuticals and personal care products, Water Res., 2017, 125, 227–236 CrossRef CAS PubMed.
- K. M. Onesios and E. J. Bouwer, Biological removal of pharmaceuticals and personal care products during laboratory soil aquifer treatment simulation with different primary substrate concentrations, Water Res., 2012, 46, 2365–2375 CrossRef CAS PubMed.
- M. Alidina, D. Li, M. Ouf and J. E. Drewes, Role of primary substrate composition and concentration on attenuation of trace organic chemicals in managed aquifer recharge systems, J. Environ. Manage., 2014, 144, 58–66 CrossRef CAS PubMed.
- S. Banzhaf, K. Nödler, T. Licha, A. Krein and T. Scheytt, Redox-sensitivity and mobility of selected pharmaceutical compounds in a low flow column experiment, Sci. Total Environ., 2012, 438, 113–121 CrossRef CAS PubMed.
- B. Baumgarten, J. Jährig, T. Reemtsma and M. Jekel, Long term laboratory column experiments to simulate bank filtration: Factors controlling removal of sulfamethoxazole, Water Res., 2011, 45, 211–220 CrossRef CAS PubMed.
- M. Alidina, J. Shewchuk and J. E. Drewes, Effect of temperature on removal of trace organic chemicals in managed aquifer recharge systems, Chemosphere, 2015, 122, 23–31 CrossRef CAS PubMed.
- U. Hübner, U. von Gunten and M. Jekel, Evaluation of the persistence of transformation products from ozonation of trace organic compounds – A critical review, Water Res., 2015, 68, 150–170 CrossRef PubMed.
- G. Knopp, C. Prasse, T. A. Ternes and P. Cornel, Elimination of micropollutants and transformation products from a wastewater treatment plant effluent through pilot scale ozonation followed by various activated carbon and biological filters, Water Res., 2016, 100, 580–592 CrossRef CAS PubMed.
- A. F. Bollmann, W. Seitz, C. Prasse, T. Lucke, W. Schulz and T. Ternes, Occurrence and fate of amisulpride, sulpiride, and lamotrigine in municipal wastewater treatment plants with biological treatment and ozonation, J. Hazard. Mater., 2016, 320, 204–215 CrossRef CAS.
- I. Zucker, H. Mamane, A. Riani, I. Gozlan and D. Avisar, Formation and degradation of N-oxide venlafaxine during ozonation and biological post-treatment, Sci. Total Environ., 2018, 619–620, 578–586 CrossRef CAS PubMed.
-
OECD, OECD Guideline for the Testing of Chemicals. Section 3, Test No. 303, 2001 Search PubMed.
- C. Lee, C. Schmidt, J. Yoon and U. von Gunten, Oxidation of N-Nitrosodimethylamine (NDMA) Precursors with Ozone and Chlorine Dioxide: Kinetics and Effect on NDMA Formation Potential, Environ. Sci. Technol., 2007, 41, 2056–2063 CrossRef CAS.
- M. Scheurer, K. Nodler, F. Freeling, J. Janda, O. Happel, M. Riegel, U. Muller, F. R. Storck, M. Fleig, F. T. Lange, A. Brunsch and H. J. Brauch, Small, mobile, persistent: Trifluoroacetate in the water cycle - Overlooked sources, pathways, and consequences for drinking water supply, Water Res., 2017, 126, 460–471 CrossRef CAS PubMed.
- H. Bader and J. Hoigné, Determination of ozone in water by the indigo method, Water Res., 1981, 15, 449–456 CrossRef CAS.
- C. K. Schmidt and H.-J. Brauch, N,N-Dimethylsulfamide as Precursor for N-Nitrosodimethylamine (NDMA) Formation upon Ozonation and its Fate During Drinking Water Treatment, Environ. Sci. Technol., 2008, 42, 6340–6346 CrossRef CAS PubMed.
- U. v. Gunten, E. Salhi, C. K. Schmidt and W. A. Arnold, Kinetics and Mechanisms of N-Nitrosodimethylamine Formation upon Ozonation of N,N-Dimethylsulfamide-Containing Waters: Bromide Catalysis, Environ. Sci. Technol., 2010, 44, 5762–5768 CrossRef PubMed.
- B. M. Patterson, M. M. Pitoi, A. J. Furness, T. P. Bastow and A. J. McKinley, Fate of N-Nitrosodimethylamine in recycled water after recharge into anaerobic aquifer, Water Res., 2012, 46, 1260–1272 CrossRef CAS PubMed.
- H.-P. Kaiser, O. Köster, M. Gresch, P. M. J. Périsset, P. Jäggi, E. Salhi and U. von Gunten, Process Control For Ozonation Systems: A Novel Real-Time Approach, Ozone: Sci. Eng., 2013, 35, 168–185 CrossRef CAS.
- M. Scheurer, M. Godejohann, A. Wick, O. Happel, T. A. Ternes, H.-J. Brauch, W. K. L. Ruck and F. T. Lange, Structural elucidation of main ozonation products of the artificial sweeteners cyclamate and acesulfame, Environ. Sci. Pollut. Res., 2012, 19, 1107–1118 CrossRef CAS PubMed.
- S. Castronovo, A. Wick, M. Scheurer, K. Nodler, M. Schulz and T. A. Ternes, Biodegradation of the artificial sweetener acesulfame in biological wastewater treatment and sandfilters, Water Res., 2017, 110, 342–353 CrossRef CAS PubMed.
- S. Kahl, S. Kleinsteuber, J. Nivala, M. van Afferden and T. Reemtsma, Emerging Biodegradation of the Previously Persistent Artificial Sweetener Acesulfame in Biological Wastewater Treatment, Environ. Sci. Technol., 2018, 52, 2717–2725 CrossRef CAS PubMed.
- I. J. Buerge, H.-R. Buser, M. Kahle, M. D. Müller and T. Poiger, Ubiquitous Occurrence of the Artificial Sweetener Acesulfame in the Aquatic Environment: An Ideal Chemical Marker of Domestic Wastewater in Groundwater, Environ. Sci. Technol., 2009, 43, 4381–4385 CrossRef CAS PubMed.
- M. Scheurer, F. R. Storck, H.-J. Brauch and F. T. Lange, Performance of conventional multi-barrier drinking water treatment plants for the removal of four artificial sweeteners, Water Res., 2010, 44, 3573–3584 CrossRef CAS PubMed.
- M. M. Huber, S. Canonica, G.-Y. Park and U. von Gunten, Oxidation of Pharmaceuticals during Ozonation and Advanced Oxidation Processes, Environ. Sci. Technol., 2003, 37, 1016–1024 CrossRef CAS PubMed.
- D. C. McDowell, M. M. Huber, M. Wagner, U. von Gunten and T. A. Ternes, Ozonation of Carbamazepine in Drinking Water: Identification and Kinetic Study of Major Oxidation Products, Environ. Sci. Technol., 2005, 39, 8014–8022 CrossRef CAS PubMed.
- U. Hübner, B. Seiwert, T. Reemtsma and M. Jekel, Ozonation products of carbamazepine and their removal from secondary effluents by soil aquifer treatment – Indications from column experiments, Water Res., 2014, 49, 34–43 CrossRef PubMed.
- K. Hellauer, D. Mergel, A. Ruhl, J. Filter, U. Hübner, M. Jekel and J. Drewes, Advancing Sequential Managed Aquifer Recharge Technology (SMART) Using Different Intermediate Oxidation Processes, Water, 2017, 9, 221 CrossRef.
- C. Bertelkamp, A. R. Verliefde, K. Schoutteten, L. Vanhaecke, J. Vanden Bussche, N. Singhal and J. P. van der Hoek, The effect of redox conditions and adaptation time on organic micropollutant removal during river bank filtration: A laboratory-scale column study, Sci. Total Environ., 2016, 544, 309–318 CrossRef CAS PubMed.
- K. Schoutteten, T. Hennebel, E. Dheere, C. Bertelkamp, D. J. De Ridder, S. Maes, M. Chys, S. W. H. Van Hulle, J. Vanden Bussche, L. Vanhaecke and A. R. D. Verliefde, Effect of oxidation and catalytic reduction of trace organic contaminants on their activated carbon adsorption, Chemosphere, 2016, 165, 191–201 CrossRef CAS PubMed.
- Y. Han, M. Ma, N. Li, R. Hou, C. Huang, Y. Oda and Z. Wang, Chlorination, chloramination and ozonation of carbamazepine enhance cytotoxicity and genotoxicity: Multi-endpoint evaluation and identification of its genotoxic transformation products, J. Hazard. Mater., 2018, 342, 679–688 CrossRef CAS PubMed.
- E. Kaiser, C. Prasse, M. Wagner, K. Broder and T. A. Ternes, Transformation of oxcarbazepine and human metabolites of carbamazepine and oxcarbazepine in wastewater treatment and sand filters, Environ. Sci. Technol., 2014, 48, 10208–10216 CrossRef CAS PubMed.
- E. Brezina, C. Prasse, J. Meyer, H. Muckter and T. A. Ternes, Investigation and risk evaluation of the occurrence of carbamazepine, oxcarbazepine, their human metabolites and transformation products in the urban water cycle, Environ. Pollut., 2017, 225, 261–269 CrossRef CAS PubMed.
- Z. Li, H. Fenet, E. Gomez and S. Chiron, Transformation of the antiepileptic drug oxcarbazepine upon different water disinfection processes, Water Res., 2011, 45, 1587–1596 CrossRef CAS PubMed.
- A. Azais, J. Mendret, G. Cazals, E. Petit and S. Brosillon, Ozonation as a pretreatment process for nanofiltration brines: Monitoring of transformation products and toxicity evaluation, J. Hazard. Mater., 2017, 338, 381–393 CrossRef CAS PubMed.
- M. M. Sein, M. Zedda, J. Tuerk, T. C. Schmidt, A. Golloch and C. v. Sonntag, Oxidation of Diclofenac with Ozone in Aqueous Solution, Environ. Sci. Technol., 2008, 42, 6656–6662 CrossRef CAS PubMed.
- A. D. Coelho, C. Sans, A. Aguera, M. J. Gomez, S. Esplugas and M. Dezotti, Effects of ozone pre-treatment on diclofenac: intermediates, biodegradability and toxicity assessment, Sci. Total Environ., 2009, 407, 3572–3578 CrossRef CAS PubMed.
- T. Kosjek, D. Zigon, B. Kralj and E. Heath, The use of quadrupole-time-of-flight mass spectrometer for the elucidation of diclofenac biotransformation products in wastewater, J. Chromatogr. A, 2008, 1215, 57–63 CrossRef CAS PubMed.
- I. Forrez, M. Carballa, K. Verbeken, L. Vanhaecke, T. Ternes, N. Boon and W. Verstraete, Diclofenac oxidation by biogenic manganese oxides, Environ. Sci. Technol., 2010, 44, 3449–3454 CrossRef CAS PubMed.
- J. Groning, C. Held, C. Garten, U. Claussnitzer, S. R. Kaschabek and M. Schlomann, Transformation of diclofenac by the indigenous microflora of river sediments and identification of a major intermediate, Chemosphere, 2007, 69, 509–516 CrossRef PubMed.
- H. El-Taliawy, M. E. Casas and K. Bester, Removal of ozonation products of pharmaceuticals in laboratory Moving Bed Biofilm Reactors (MBBRs), J. Hazard. Mater., 2018, 347, 288–298 CrossRef CAS PubMed.
- J. Tolls, Sorption of Veterinary Pharmaceuticals in Soils: A Review, Environ. Sci. Technol., 2001, 35, 3397–3406 CrossRef CAS PubMed.
- M. Kosmulski, The pH-Dependent Surface Charging and the Points of Zero Charge, J. Colloid Interface Sci., 2002, 253, 77–87 CrossRef CAS PubMed.
- J. A. Benesch, M. S. Gustin, G. R. Cramer and T. M. Cahill, Investigation of effects of trifluoroacetate on vernal pool ecosystems, Environ. Toxicol. Chem., 2002, 21, 640–647 CrossRef CAS PubMed.
- D. A. Ellis, M. L. Hanson, P. K. Sibley, T. Shahid, N. A. Fineberg, K. R. Solomon, D. C. Muir and S. A. Mabury, The fate and persistence of trifluoroacetic and chloroacetic acids in pond waters, Chemosphere, 2001, 42, 309–318 CrossRef CAS PubMed.
- P. T. Visscher, C. W. Culbertson and R. S. Oremland, Degradation of trifluoroacetate in oxic and anoxic sediments, Nature, 1994, 369, 729–731 CrossRef CAS.
- B. Kim, M. Suidan, T. Wallington and X. Du, Biodegradability of trifluoroacetic acid, Environ. Eng. Sci., 2000, 17, 337–342 CrossRef CAS.
- J. C. Boutonnet, P. Bingham, D. Calamari, C. d. Rooij, J. Franklin, T. Kawano, J.-M. Libre, A. McCul-loch, G. Malinverno, J. M. Odom, G. M. Rusch, K. Smythe, I. Sobolev, R. Thompson and J. M. Tiedje, Environmental Risk Assessment of Trifluoroacetic Acid, Hum. Ecol. Risk Assess., 1999, 5, 59–124 CrossRef CAS.
Footnote |
† Electronic supplementary information (ESI) available: Detailed description of analytical techniques and additional experimental data. See DOI: 10.1039/c8ew00855h |
|
This journal is © The Royal Society of Chemistry 2019 |
Click here to see how this site uses Cookies. View our privacy policy here.