Phosphate recovery from wastewater using calcium silicate hydrate (C-S-H): sonochemical synthesis and properties†
Received
14th September 2018
, Accepted 19th November 2018
First published on 22nd November 2018
Abstract
In this study, a novel ultrasound-assisted sol–gel method was developed for the room-temperature synthesis of single-phase calcium silicate hydrate (C-S-H). The optimum synthesis conditions and the phosphate recovery properties of C-S-H were investigated by batch experiments. Samples were characterized by scanning electron microscopy (SEM), specific surface area analysis, 29Si magic-angle spinning nuclear magnetic resonance (29Si MAS-NMR) spectroscopy, and X-ray diffraction (XRD). The results showed that ultrasound irradiation played a vital role in the rapid and effective synthesis of C-S-H at room temperature, and the Ca/Si ratio of 1.2 was the most appropriate raw material ratio. In the process of phosphate recovery, C-S-H acted as the seed crystal, the calcium ion donor, and the pH adjuster at the same time, and therefore phosphate could be recovered in one-step crystallization without adding other chemicals. The adsorption kinetics and adsorption isotherms of phosphate recovery could be well described by the pseudo-second-order model and the Langmuir model, respectively. The thermodynamic parameters indicated that the phosphate recovery process was endothermic and spontaneous. The phosphate adsorption capacity on C-S-H could reach 109.4 mg g−1 when the initial pH was 5.0 and the temperature was 298 K. Unlike the case of using Ca(OH)2, only a slight degree of carbonate inhibition was observed for the phosphate recovery by C-S-H. All the results indicate that the ultrasound-assisted sol–gel synthesis is a valuable method for C-S-H synthesis.
Water impact
Calcium silicate hydrate (C-S-H) is a promising material for phosphate recovery from wastewater, but the difficulty of synthesis limits its application. We have developed a simple sonochemical method to synthesize C-S-H at room temperature, which contributes to a larger specific surface area and better phosphate recovery performance, thus providing environmental benefits for pollution control and resource recovery.
|
1. Introduction
Phosphorus (P) is one of the essential resources for human beings. Although the world's supply of phosphate rock does not appear to be depleted immediately, the limited nature and geographical concentration of phosphorus resources still cause a common concern.1,2 On the other hand, many phosphorus resources are lost during human production and consumption,3 and these losses are considered to be the primary cause of eutrophication in water environments, thus harming water quality and ecological balance.4 Therefore, phosphate recovery from wastewater is a necessary measure to reduce environmental pollution and promote the sustainable use of phosphorus resources. The precipitation of calcium phosphate compounds, mainly including dicalcium phosphate (CaHPO4·2H2O), octacalcium phosphate (Ca8H(PO4)6·5H2O) and hydroxyapatite (Ca5(PO4)3OH), is a cost-effective method for phosphate recovery from wastewater.5 The recovered product is similar to the active ingredient of phosphate rocks and can be readily accepted by the phosphate industry.6 However, the crystallization of calcium phosphate can only occur at high supersaturation levels, which limits its application in wastewater containing a low concentration of phosphate.7 Besides, the final product of calcium phosphate crystallization is usually a slurry which is hard to separate and dewater.8
Calcium silicate hydrate (C-S-H) is the main component of Portland cement9 and a promising layered adsorbent.10 Some studies considered that C-S-H could be used as a phosphate recovery material in the biological wastewater treatment process, due to its excellent biocompatibility and biodegradability.11 In the phosphate recovery process, C-S-H acts as the seed crystal, the calcium ion donor, and the pH adjuster at the same time, and therefore it can recover phosphate in a one-step crystallization process without adding other chemicals.12 This process is less affected by carbonate, and the recovered product can be easily separated from wastewater.13 However, the artificial synthesis of C-S-H is tricky. In general, C-S-H is synthesized in an alkaline and hydrothermal environment at 170–220 °C.14 This synthesis method is sufficient to obtain a valuable product,15 but the cost is high. Some further studies suggested that C-S-H should be synthesized by a precipitation process at low temperature to save energy and simplify operation.13 However, the C-S-H synthesized by the low-temperature reaction often has a heterogeneous structure and a low specific surface area, thus impairing its usefulness. Furthermore, this material cannot even be considered as single-phase C-S-H, because there are significant structural differences between the fractions containing various Ca/Si ratios.
Ultrasound-assisted synthesis is a valuable method for preparing nanostructure inorganic materials.16 In particular, ultrasound creates a unique environment for sol–gel synthesis, leading to a more homogeneous gel. Ultrasound-assisted sol–gel synthesis is often used to prepare photocatalysts with excellent properties, such as TiO2 and ZnO.17,18 When the sol is irradiated by ultrasound, the energy can significantly increase the chemical reactivity, thereby reducing the solvent dosage and gelation time. Besides, the effect of ultrasound cavitation also helps to improve the specific surface area and pore volume.19 So far, there have been a few reports on the ultrasound-assisted sol–gel synthesis of C-S-H. The higher reactivity of this method may provide C-S-H with better physicochemical properties and phosphate recovery performance. Furthermore, the limited knowledge of the structure of C-S-H synthesized at room temperature is a serious obstacle to its further application.20 The C-S-H synthesized by the ultrasound-assisted sol–gel method may have a single phase, which creates favorable conditions for characterization and structural analysis.
Therefore, this study aims to develop a low-temperature method to synthesize single-phase C-S-H with good phosphate recovery performance. The recovered product can be used as a substitute for phosphate rock, or according to our previous study,21 it can also be used as a high-value material for the immobilization of heavy metals in soils. In this study, C-S-H was synthesized using an ultrasound-assisted sol–gel method at room temperature. The exact chemical formulas and structures of samples were identified through characterization and theoretical analysis. The influence of structures on the phosphate recovery performance was explored by controlling different Ca/Si ratios of raw materials. The adsorption properties of phosphate on C-S-H were investigated by batch experiments, and the effects of pH, contact time, initial concentration and temperature were considered. The adsorption kinetic model and adsorption isotherm model were fitted to the experimental data, and the adsorption mechanism was discussed.
2. Methods
2.1. Chemicals
All chemicals involved in this study were of analytical grade without further purification and bought from Sinopharm Chemical Reagent Co., Ltd. A stock solution of phosphate (150 mg L−1) was prepared by dissolving the appropriate quantity of KH2PO4·6H2O in deionized water. The simulated phosphate wastewater samples used in this study were obtained by dilution of the stock solution, and the initial pH of the wastewater was adjusted by adding 0.01 M HNO3 or 0.01 M NaOH solutions.
2.2. The synthesis of C-S-H
C-S-H was synthesized by a rapid ultrasound-assisted sol–gel method at room temperature. Firstly, a CaCl2 solution was added dropwise into a Na2SiO3 solution under ultrasound irradiation (300 W) with different Ca/Si molar ratios (0.8, 1.0, 1.2, 1.4, and 1.6) and a solid/liquid ratio of 1/8. Immediately, a white sol was yielded and gradually hardened. After 10 min, the sample was taken out and gelled in a plastic container at room temperature. Then, the gel was gently mixed in deionized water (10 g L−1) for 1 h to remove free Ca(OH)2. The suspension was filtered through a 0.45 μm membrane filter, and the cake was dried at 80 °C for 24 h in an oven. Finally, C-S-H was obtained by screening 80–150 μm powders. Besides, a control sample was prepared using a conventional sol–gel method, which followed the above steps except that it was not subjected to ultrasound.
2.3. Characterization methods
The morphology of the samples was observed by field emission scanning electron microscopy (FE-SEM) (Zeiss Ultr55, Germany). The specific surface area and pore-size distribution of the samples were determined using a N2 adsorption–desorption analyzer (JWGB BK112, China). The specific surface area was measured using the Brunauer–Emmett–Teller (BET) method, and the pore-size distribution was derived from the desorption curve using the Barrett–Joyner–Halenda (BJH) model. The total pore volume was calculated from the adsorbed amount of N2 at a relative pressure of p/p0 = 0.99. XRD patterns were obtained from an X-ray diffractometer (Bruker D8 ADVANCE, Germany) with Ni-filtered Cu Kα radiation (40 kV, 40 mA). 29Si MAS NMR spectra were obtained using a 400 MHz spectrometer (Bruker AVANCE III, Germany) at 79.5 MHz, and approximately 200 scans were accumulated with a relaxation delay of 30 s. The chemical shift of 29Si was referenced relative to tetramethylsilane (TMS). Gaussian response function deconvolution was carried out for the target peak using PeakFit software (version 4.12). The quantitative elemental concentrations were analyzed using an X-ray fluorescence spectrometer (XRF) (Shimadzu XRF-1800, Japan).
2.4. Phosphate recovery experiments
An appropriate dosage (0.6 g L−1) of C-S-H samples was added into the simulated phosphate wastewater (60 mg L−1) for phosphate recovery. An exception was that the adsorption isotherm was studied in wastewater samples with different concentrations of phosphate (from 20 to 140 mg L−1). The mixture was shaken in a thermostatic shaker at a speed of 180 rpm. After the reaction, the solution was separated from the mixture through a 0.22 μm membrane filter for further analysis. The concentration of phosphate was analyzed by the molybdenum blue method with spectrophotometers (Shimadzu UV-2550, Japan), the concentration of Ca2+ was measured with an inductively coupled plasma emission spectrometer (ICP-OES) (Agilent 720ES, USA), and the pH was monitored with a pH meter (Mettler Toledo Seven2Go, Switzerland). The phosphate removal efficiency (Re, %) and the phosphate adsorption capacity of C-S-H (Qe, mg g−1) were calculated by the following equations: | Re = (C0 − Ce)/C0 × 100% | (1) |
where C0 and Ce are the initial and equilibrium concentrations of phosphate (mg L−1), respectively. V is the volume of working solution (L), and m is the dosage of C-S-H (g).
3. Results and discussion
3.1. The effect of the ultrasound process on C-S-H synthesis
To illustrate the necessity for an ultrasound process for the C-S-H synthesis, two samples with a Ca/Si ratio of 1.2 were synthesized by the conventional sol–gel method and ultrasound-assisted sol–gel method, respectively. The surface morphologies of the samples were identified by SEM (Fig. 1). The sample synthesized by the conventional sol–gel method consists of some packed acicular structures and some irregular structures, which may correspond to the phase with a high Ca/Si ratio and the phase with a low Ca/Si ratio, respectively.22 The structural difference in this sample is likely due to the insufficient synthesis conditions, and the result shows that the C-S-H with a specific Ca/Si ratio is not obtained as expected. In contrast, the sample synthesized by the ultrasound-assisted sol–gel method contains many warped nanosheets, and these nanosheets intersect to form a relatively homogeneous porous structure. During the formation of C-S-H, the loss of some bridging silicates results in the displacement, tilting, and rotation of the silicate chains, thus warping the nanosheets.
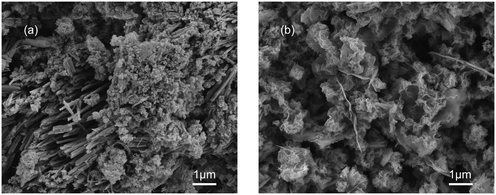 |
| Fig. 1 SEM images of C-S-H synthesized by (a) conventional sol–gel and (b) ultrasound-assisted sol–gel methods. | |
The N2 adsorption–desorption isotherms and the corresponding pore-size distribution curves of the C-S-H samples synthesized by conventional sol–gel and ultrasound-assisted sol–gel methods are presented in Fig. 2. The result shows that the ultrasound process improves the specific surface area from 17.31 m2 g−1 to 37.20 m2 g−1 (obtained by the BET method) and the total pore volume from 0.0651 cm3 g−1 to 0.1779 cm3 g−1. The N2 adsorption–desorption isotherms are close to type IV (BET classification) and the hysteresis loops at high pressures between 0.6 and 1.0 display the presence of mesopores (2–50 nm). The shape of the hysteresis loops is type H3, which is related to the slit-shaped pores formed by the aggregation of plate-like particles. In conclusion, the sample synthesized by the ultrasound-assisted sol–gel method has a better phase-purity and a richer pore structure, which is mainly caused by the effect of ultrasound cavitation.
 |
| Fig. 2 (a) N2 adsorption–desorption isotherms and (b) pore-size distribution curves of C-S-H synthesized by the conventional sol–gel method (square) and the ultrasound-assisted sol–gel method (triangle). The solid symbol and the hollow symbol in (a) represent the adsorption and desorption curves, respectively. | |
The phosphate adsorption process on the C-S-H samples synthesized by the conventional sol–gel method and ultrasound-assisted sol–gel method was investigated through contrast experiments (Fig. 3). In general, this is a multi-step process, involving bulk diffusion, boundary layer diffusion, intraparticle diffusion and finally bonding at the sites. To illustrate the effect of the sample structure on the mass transfer, the intraparticle diffusion model was used to describe the adsorption process, and the description of this model and the fitting results are shown in Table S1.† The result confirms that the adsorption process in this study shows a multi-linearity correlation, and the second stage may correspond to a slow intraparticle diffusion process. The kint,2 value of the sample synthesized by the ultrasound-assisted sol–gel method has a significant increase compared with that of the sample synthesized by the conventional sol–gel method. The reason can be attributed to the larger pore volume of C-S-H, which is consistent with the conclusion of characterization. Finally, the ultrasound process increases the maximum phosphate adsorption capacity of C-S-H by 38.6%. Therefore, the ultrasound process is a crucial condition for the effective synthesis of C-S-H at room temperature.
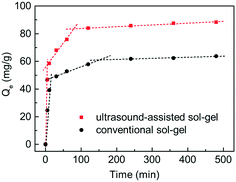 |
| Fig. 3 The intraparticle diffusion model of phosphate adsorption on C-S-H synthesized by conventional sol–gel and ultrasound-assisted sol–gel methods (the dosage is 0.6 g L−1, the initial pH is 5.0, and the temperature is 298 K). | |
3.2. The effect of Ca/Si ratios on the ultrasound-assisted sol–gel synthesis of C-S-H
To identify the phases of C-S-H synthesized by the ultrasound-assisted sol–gel method with different Ca/Si ratios of raw materials (0.8, 1.0, 1.2, 1.4, and 1.6), the XRD patterns were presented with the same scale (Fig. S1†). The result shows that the five C-S-H samples are similar and mainly composed of C-S-H(I) (JCPDS 34-0002) and calcite (JCPDS 47-1743). The formation of calcite is caused by the unavoidable contact with atmospheric carbon dioxide in the synthesis process, but its content is low. Besides, no detected reflections corresponding to Ca(OH)2 are exhibited in all five samples suggesting that the free Ca(OH)2 is removed by washing. According to the fitting of Jade 6.0 software (Materials Data Inc., USA), the crystallinities of the C-S-H samples are determined to be 21.80%, 32.60%, 38.22%, 39.58% and 41.33%, respectively, which suggest that C-S-H has a low-crystallinity structure, and increased Ca/Si ratios are associated with higher crystallinity.
The chemical compositions of the C-S-H samples were determined by XRF (Table 1). The result shows that calcium (Ca) and silicon (Si) are the prime elements of C-S-H, aside from carbon, oxygen, and hydrogen which are not detected by XRF. The content of elements was expressed in the form of oxides and the actual Ca/Si ratios of C-S-H were computed. Considering that the free Ca(OH)2 is washed away during the synthesis process as shown in the XRD patterns, the Ca/Si ratio of the raw material does not necessarily represent the Ca/Si ratio of the product, the actual Ca/Si ratios of C-S-H are relatively fixed from 0.91 to 1.17 compared with the Ca/Si ratios of raw materials from 0.8 to 1.6.
Table 1 The chemical compositions of C-S-H synthesized with different Ca/Si ratios
Ideal Ca/Si ratios |
Chemical compositions |
Actual Ca/Si ratios |
The normalized area of Qn |
Average chain length |
Chemical formula |
CaO (wt%) |
SiO2 (wt%) |
Others (wt%) |
Q1 (%) |
Q2b (%) |
Q2c (%) |
0.8 |
42.96 |
50.71 |
6.33 |
0.91 |
18.43 |
23.74 |
57.83 |
10.9 |
Ca9.9(SiO3.1)10.9H4.2 |
1.0 |
46.02 |
47.08 |
6.90 |
1.05 |
12.33 |
22.17 |
65.50 |
16.2 |
Ca17.0(SiO3.1)16.2H1.6 |
1.2 |
47.06 |
45.93 |
7.01 |
1.10 |
10.42 |
25.45 |
64.13 |
19.2 |
Ca21.1(SiO3.1)19.2 |
1.4 |
47.34 |
45.34 |
7.32 |
1.12 |
9.34 |
22.06 |
68.60 |
21.4 |
Ca24.0(SiO3.0)21.4(OH)5.2 |
1.6 |
47.81 |
43.68 |
8.51 |
1.17 |
9.83 |
21.71 |
68.46 |
20.3 |
Ca23.8(SiO3.0)20.3(OH)7.0 |
In general, C-S-H is considered as a layered structure composed of silicate chains and bonding calcium (Fig. S2†). Some studies suggest that sorosilicates are original structures during the synthesis of C-S-H and then they are connected by bridging silicates.15 In this study, the symbol Qn represents a silicate tetrahedron connected to n silicate tetrahedra, and in particular, Q2b and Q2c represent a bridging silicate tetrahedron and a common silicate tetrahedron, respectively.
The structures of the C-S-H samples were investigated by 29Si MAS-NMR (Fig. 4). According to some references, three distinct peaks located at 80.2, 83.6 and 86.4 ppm correspond to Q1, Q2b, and Q2c of C-S-H, respectively.23 During the fitting procedure, peak widths in a spectrum were allowed to vary freely but with strict consistency for the same peak in different spectra. The peak areas of Q1, Q2b, and Q2c were calculated by integration with the normalized form, and the average chain lengths of silicate were calculated by the following equations:
 |
| Fig. 4 Deconvolution results (black line) and fitting results (color line) for the 29Si MAS NMR spectra of C-S-H synthesized with the Ca/Si ratios of (a) 0.8, (b) 1.0, (c) 1.2, (d) 1.4, (e) 1.6. | |
The result of 29Si MAS-NMR shows that the average chain lengths substantially increase with higher Ca/Si ratios of raw materials (Table 1), which is consistent with the analysis of crystallinity in the XRD patterns. Furthermore, the oxygen content of the silicate tetrahedron depends on the length of the silicate chain, and H+ or OH− is in addition to the neutralizing charge, thus acquiring the exact chemical formula of the C-S-H samples. The change in the chemical structures of C-S-H synthesized with different Ca/Si ratios is also supported by X-ray photoelectron spectroscopy (XPS) (Fig. S3†).24
The phosphate removal efficiency on the C-S-H samples with different Ca/Si ratios was compared by batch experiments. As shown in Fig. 5, the phosphate removal efficiency of C-S-H peaks at the Ca/Si ratio of 1.2, which can remove 86.8% of the phosphate after 2 h reaction. This result is consistent with the Ca2+ release experiment in pure water (as shown in Table S2†), indicating that the formation of calcium phosphate compounds may be the principal mechanism of phosphate recovery. Considering that the actual Ca/Si ratios of C-S-H are between 0.91 and 1.17, we suggest that the Ca/Si ratio of 1.2 is a reasonable synthesis condition for better phosphate recovery performance and atom economy.
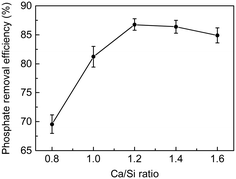 |
| Fig. 5 Phosphate recovery efficiency on C-S-H synthesized with different Ca/Si ratios (the dosage is 0.6 g L−1, the initial pH is 5.0, the contact time is 2 h, and the temperature is 298 K; error bars indicate standard deviations of triplicate measurements). | |
3.3. Phosphate adsorption kinetics at different initial pH values
To study the effect of reaction time and pH on the phosphate recovery capacity of C-S-H synthesized by the ultrasound-assisted sol–gel method with a Ca/Si ratio of 1.2, some supernatants were collected by batch experiment at different initial pH values (5.0, 7.0 and 9.0) and different contact times (from 5 to 480 min), and the residual concentrations of phosphate and the corresponding pH were determined (Fig. 6). The results were fitted to the adsorption kinetic model, and the term “adsorption” was herein used as a general term to depict the transfer of phosphate from wastewater to the surface of C-S-H.
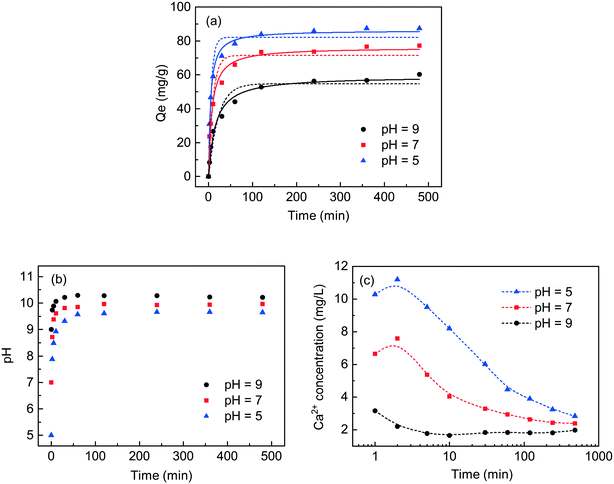 |
| Fig. 6 (a) The effect of initial pH on phosphate recovery efficiency, (b) the corresponding changes of pH, and (c) the concentration changes of Ca2+ as a function of contact time. The dashed lines represent the pseudo-first-order model, and the solid lines represent the pseudo-second-order model (the dosage of C-S-H is 0.6 g L−1, the initial concentration of phosphate is 60 mg L−1, and the temperature is 298 K). | |
The adsorption capacity increased rapidly within 120 min and the prolonged contact time did not significantly increase the phosphate adsorption capacity. The change in solution pH was monitored to observe the amount of released OH− during the adsorption process. The result shows that C-S-H as a pH adjuster can release appropriate concentrations of OH− to maintain the final pH between 9.6 and 10.2 regardless of initial pH. This observation implies the dissolution of the calcium interlayer, which causes OH− to be released into the solution. Afterward, the phosphate in wastewater combines with the exposed calcium in situ or the dissolved calcium from C-S-H to form a calcium phosphate compound, as shown in Fig. S5.†25,26 Because the release of Ca2+ is positively related to the release of OH−, the lower initial pH can achieve better phosphate recovery capacities. Finally, the elemental analysis of XRF (in terms of oxides) showed that the recovered product with the initial pH of 5.0 contained 40.65% calcium, 35.15% silicon, 18.64% phosphorus and 5.56% other substances. Compared with the initial pH of 7.0, an extra recovery of each gram of phosphate requires the addition of approximately 1.6 mmol acid. This seems to be a potential cost that needs to be properly considered in the actual application.
The kinetics of the phosphate adsorption process on C-S-H was fitted using pseudo-first-order and pseudo-second-order kinetic models. The fitting methods and the results of equilibrium data are listed in Table S3.† The correlation coefficients (R2) of the pseudo-second-order model are quite close to 1.0 (from 0.982 to 0.993), so the pseudo-second-order model can better describe the phosphate adsorption properties of C-S-H.
To observe the phase compositions of the phosphate recovery products of C-S-H at different times, the XRD patterns of a series of recovered products with the initial pH of 5.0 are obtained and shown in Fig. 7. When the contact time was 8 h, the recovered product appears to be hydroxyapatite (HAP) (JCPDS 09-0432). The result indicates that HAP began to be generated and covered the surface of C-S-H in about 8 h, and the reflections of C-S-H were hardly detectable until 24 h. However, the phosphate recovery efficiency of C-S-H already reached 83.6% at 2 h, and the FT-IR spectra proved the presence of phosphate on C-S-H (Fig. S4†), but no visible product was detected in the XRD pattern. Some studies have considered that it is tough to form HAP directly for the kinetic factor and two-step or multi-step crystallization is necessary.27
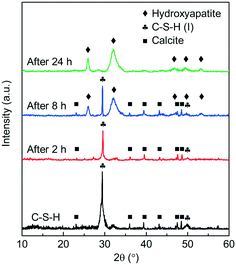 |
| Fig. 7 XRD patterns of the phosphate recovery products of C-S-H through the different reaction times. | |
The transformation from amorphous calcium phosphate (ACP) into HAP is typically considered as a simplified model in laboratory studies.28 The SEM images (Fig. 8) show that the spherical particles corresponding to ACP generated on the C-S-H surface at 2 h reaction time and the acicular structure corresponding to HAP generated on the C-S-H surface at 24 h reaction time. This observation favors the idea of ACP–HAP transformation and shows that phosphate can be rapidly recovered by forming amorphous intermediates on the surface of C-S-H, although kinetic inhibition leads to the slow formation of hydroxyapatite.
| Ca2+ + HPO2−4 = CaHPO4 | (5) |
| 6CaHPO4 + 4Ca2+ + 80H− = Ca10(PO4)6(OH)2 + 6H2O | (6) |
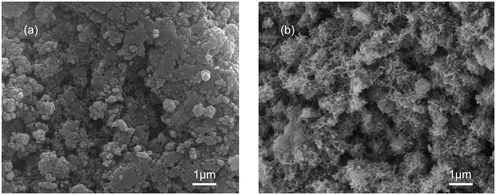 |
| Fig. 8 SEM images of the phosphate recovery product of C-S-H after 2 h (a) and 24 h reaction (b). | |
3.4. Phosphate adsorption isotherms at different temperatures
The effect of temperature was studied at 288 K, 298 K, and 308 K, respectively, and the adsorption capacities of C-S-H were determined at different initial phosphate concentrations (Fig. 9). The Langmuir and Freundlich isotherm models are used to describe and analyze the phosphate adsorption on C-S-H. The fitting methods and the results of equilibrium data are listed in Table S4,† and the Langmuir isotherm model shows a better fit than the Freundlich isotherm model. The Langmuir model can describe a uniform distribution of active sites on the surface and the monolayer coverage of the phosphate occurring on the surface of C-S-H after adsorption, because the Langmuir model assumes that the bulk phases and surfaces of homogeneous sorbents exhibit an ideal behavior with all the adsorption sites being identical and energy being equivalent.
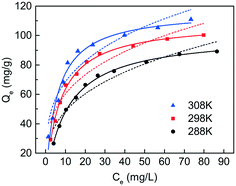 |
| Fig. 9 Adsorption isotherms of phosphate on C-S-H at different temperatures (the dosage is 0.6 g L−1, the initial pH is 5.0 and the contact time is 2 h; the solid lines represent the Langmuir model, and the dashed lines represent the Freundlich model). | |
The adsorption capacities are highest at T = 308 K and lowest at T = 288 K, indicating that the phosphate adsorption by C-S-H is promoted at high temperature. Thermodynamic parameters including the enthalpy change (ΔH0), entropy change (ΔS0) and free energy change (ΔG0) of the phosphate adsorption process can be calculated from temperature-dependent adsorption isotherms. K0 can be calculated by plotting ln
Kdversus Ce and extrapolating Ce to zero. ΔH0 and ΔS0 are calculated from the slope and intercept of the plots of ln
K0versus 1/T.
| ΔG0 = −RT ln K0 | (7) |
| 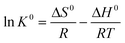 | (8) |
where
R (8.314 J mol
−1 K
−1) is the universal gas constant,
T is the temperature in Kelvin and
K0 is the adsorption equilibrium constant. The calculation results of the thermodynamic parameters are listed in
Table 2.
Table 2 Thermodynamic parameters for phosphate adsorption on C-S-H
Adsorbent |
T (K) |
ΔH0 (kJ mol−1) |
ΔS0 (J mol−1 K−1) |
ΔG0 (kJ mol−1) |
C-S-H |
288 |
26.69 |
164.7 |
−20.74 |
298 |
|
|
−22.39 |
308 |
|
|
−24.04 |
The value of ΔH0 is 26.69 kJ mol−1, which indicates that the phosphate adsorption process is endothermic. The positive value of ΔS0 indicates an increase in the randomness of the reaction process. The negative value of ΔG0 indicates that the phosphate recovery process is spontaneous under the experimental conditions. The ΔG0 decreases as the reaction temperature (T) increases, indicating that the high temperature is more favorable for the reaction.
According to the fitting of the Langmuir model, the Qm can reach 109.4 mg g−1, when the initial pH is 5.0 and the temperature is 298 K, which is better than the relevant materials in the literature (Table 3). The result implies that the ultrasound-assisted sol–gel method can replace the existing synthesis method of C-S-H and would meet the requirements for the recovery and reuse of phosphorus resources.
Table 3 The phosphate removal capacities on various adsorbents in the literature
Materials |
Removal capacity (mg g−1) |
pH |
Temperature (K) |
Reference |
C-S-H |
109.4 |
5.0 |
298 |
This study |
C-S-H |
24.5 |
5.0 |
298 |
13
|
C-S-H |
31.1 |
7.5 |
298 |
29
|
C-S-H |
48.6 |
7.6 |
298 |
30
|
3.5. Effect of carbonate anions on phosphate recovery by C-S-H
The presence of carbonate can inhibit the activity of Ca2+, thus weakening the phosphate removal performance.31 Since the actual wastewater often contains a high concentration of carbonate, it is necessary to examine the effect of carbonate on the phosphate removal efficiency of C-S-H. As shown in Fig. 10, the phosphate removal by Ca(OH)2 was strongly inhibited by the increasing concentration of carbonate in wastewater. When the concentration of carbonate was 2.0 g L−1, the phosphate removal efficiency of Ca(OH)2 decreased by 55.6% compared with the blank control group. By contrast, only slight inhibition was observed on C-S-H. When the concentration of carbonate was 2.0 g L−1, the phosphate removal efficiency of C-S-H decreased by 24.4%. The reason can be explained by the higher alkalinity of Ca(OH)2, which increases the proportion of CO32− species in the carbonate and promotes the formation of calcium carbonate precipitates.
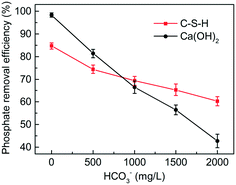 |
| Fig. 10 Effects of carbonate on phosphate removal by C-S-H and Ca(OH)2 (the dosage is 0.6 g L−1, the initial pH is 5.0, the contact time is 2 h, and the temperature is 298 K; error bars indicate standard deviations of triplicate measurements). | |
4. Conclusion
In this study, a simple ultrasound-assisted sol–gel method was developed for the synthesis of C-S-H, and it was proved to be an effective method to solve the difficulty of low-temperature synthesis of C-S-H. The synthesized C-S-H has great potential for phosphate recovery from wastewater because of its low cost, exact chemistry structure, and good phosphate recovery performance. The results indicated that ultrasound irradiation has a significant effect on the structural characteristics and phosphate recovery performance of C-S-H, and the Ca/Si ratio of 1.2 is chosen as the optimum synthesis ratio. The synthesized C-S-H can efficiently recover phosphate over a wide range of initial pH, and the recovery efficiency is only slightly affected by carbonate. The C-S-H could recover most phosphate from wastewater in 2 h by forming amorphous calcium phosphate. The adsorption kinetics and adsorption isotherms of phosphate recovery could be well described by the pseudo-second-order model and the Langmuir model, respectively. The thermodynamic parameters indicated that the phosphate recovery process was endothermic and spontaneous. The removal capacities of phosphate on C-S-H could reach 109.4 mg g−1. The recovered product can be used as a substitute for phosphate rock, and it may be used as a phosphate fertilizer or soil conditioner.
Conflicts of interest
There are no conflicts to declare.
Acknowledgements
This work was funded by the National Natural Science Foundation of China (No. 51678421, No. 41571301, and No. 21777120).
References
- B. K. Mayer, L. A. Baker, T. H. Boyer, P. Drechsel, M. Gifford, M. A. Hanjra, P. Parameswaran, J. Stoltzfus, P. Westerhoff and B. E. Rittmann, Total Value of Phosphorus Recovery, Environ. Sci. Technol., 2016, 50, 6606–6620 CrossRef CAS PubMed.
- E. Desmidt, K. Ghyselbrecht, Y. Zhang, L. Pinoy, B. Van der Bruggen, W. Verstraete, K. Rabaey and B. Meesschaert, Global Phosphorus Scarcity and Full-Scale P-Recovery Techniques: A Review, Crit. Rev. Environ. Sci. Technol., 2015, 45, 336–384 CrossRef CAS.
- O. F. Schoumans, W. J. Chardon, M. E. Bechmann, C. Gascuel-Odoux, G. Hofman, B. Kronvang, G. H. Rubaek, B. Ulen and J. M. Dorioz, Mitigation options to reduce phosphorus losses from the agricultural sector and improve surface water quality: A review, Sci. Total Environ., 2014, 468, 1255–1266 CrossRef PubMed.
- S. R. Carpenter, Phosphorus control is critical to mitigating eutrophication, Proc. Natl. Acad. Sci. U. S. A., 2008, 105, 11039–11040 CrossRef CAS PubMed.
- T. Tervahauta, R. D. van der Weijden, R. L. Flemming, L. H. Leal, G. Zeeman and C. J. N. Buisman, Calcium phosphate granulation in anaerobic treatment of black water: A new approach to phosphorus recovery, Water Res., 2014, 48, 632–642 CrossRef CAS PubMed.
- L. H. Peng, H. L. Dai, Y. F. Wu, Y. H. Peng and X. W. Lu, A comprehensive review of phosphorus recovery from wastewater by crystallization processes, Chemosphere, 2018, 197, 768–781 CrossRef CAS PubMed.
- M. Hermassi, C. Valderrama, J. Dosta, J. L. Cortina and N. H. Batis, Evaluation of hydroxyapatite crystallization in a batch reactor for the valorization of alkaline phosphate concentrates from wastewater treatment plants using calcium chloride, Chem. Eng. J., 2015, 267, 142–152 CrossRef CAS.
- J. A. Marshall, B. J. Morton, R. Muhlack, D. Chittleborough and C. W. Kwong, Recovery of phosphate from calcium-containing aqueous solution resulting from biochar-induced calcium phosphate precipitation, J. Cleaner Prod., 2017, 165, 27–35 CrossRef CAS.
- N. Yoobanpot, P. Jamsawang and S. Horpihulsuk, Strength behavior and microstructural characteristics of soft clay stabilized with cement kiln dust and fly ash residue, Appl. Clay Sci., 2017, 141, 146–156 CrossRef CAS.
- W. Guan and X. Zhao, Fluoride recovery using porous calcium silicate hydrates via spontaneous Ca2+ and OH- release, Sep. Purif. Technol., 2016, 165, 71–77 CrossRef CAS.
- K. Okano, S. Miyamaru, Y. Yamamoto, M. Kunisada, H. Takano, M. Toda, K. Honda and H. Ohtake, A mobile pilot-scale plant for in situ demonstration of phosphorus recovery from wastewater using amorphous calcium silicate hydrates, Sep. Purif. Technol., 2016, 170, 116–121 CrossRef CAS.
- C. G. Lee, P. J. J. Alvarez, H. G. Kim, S. Jeong, S. Lee, K. B. Lee, S. H. Lee and J. W. Choi, Phosphorous recovery from sewage sludge using calcium silicate hydrates, Chemosphere, 2018, 193, 1087–1093 CrossRef CAS PubMed.
- K. Okano, M. Uemoto, J. Kagami, K. Miura, T. Aketo, M. Toda, K. Honda and H. Ohtake, Novel technique for phosphorus recovery from aqueous solutions using amorphous calcium silicate hydrates (A-CSHs), Water Res., 2013, 47, 2251–2259 CrossRef CAS PubMed.
- W. Guan, F. Y. Ji, D. X. Fang, Y. Cheng, Z. Y. Fang, Q. K. Chen and P. Yan, Porosity formation and enhanced solubility of calcium silicate hydrate in hydrothermal synthesis, Ceram. Int., 2014, 40, 1667–1674 CrossRef CAS.
- S. Grangeon, F. Claret, C. Roosz, T. Sato, S. Gaboreau and Y. Linard, Structure of nanocrystalline calcium silicate hydrates: insights from X-ray diffraction, synchrotron X-ray absorption and nuclear magnetic resonance, J. Appl. Crystallogr., 2016, 49, 771–783 CrossRef CAS PubMed.
- B. Banerjee, Recent developments on ultrasound assisted catalyst-free organic synthesis, Ultrason. Sonochem., 2017, 35, 1–14 CrossRef CAS PubMed.
- C. Bogatu, D. Perniu, C. Sau, O. Iorga, M. Cosnita and A. Duta, Ultrasound assisted sol-gel TiO2 powders and thin films for photocatalytic removal of toxic pollutants, Ceram. Int., 2017, 43, 7963–7969 CrossRef CAS.
- Y. G. Peng, J. L. Ji, X. Y. Zhao, H. X. Wan and D. J. Chen, Preparation of ZnO nanopowder by a novel ultrasound assisted non-hydrolytic sol-gel process and its application in photocatalytic degradation of CI Acid Red 249, Powder Technol., 2013, 233, 325–330 CrossRef CAS.
- Q. G. Feng, H. D. Cai, H. Y. Lin, S. Y. Qin, Z. Liu, D. C. Ma and Y. Y. Ye, Synthesis and structural characteristics of high surface area TiO2 aerogels by ultrasonic-assisted sol-gel method, Nanotechnology, 2018, 29, 075702 CrossRef.
- I. G. Richardson, The calcium silicate hydrates, Cem. Concr. Res., 2008, 38, 137–158 CrossRef CAS.
- Z. H. Zhang, X. J. Wang, H. Wang and J. F. Zhao, Removal of Pb(II) from aqueous solution using hydroxyapatite/calcium silicate hydrate (HAP/C-S-H) composite adsorbent prepared by a phosphate recovery process, Chem. Eng. J., 2018, 344, 53–61 CrossRef CAS.
- S. Papatzani, K. Paine and J. Calabria-Holley, A comprehensive review of the models on the nanostructure of calcium silicate hydrates, Constr. Build. Mater., 2015, 74, 219–234 CrossRef.
- A. Trapote-Barreira, L. Porcar, J. Cama, J. M. Soler and A. J. Allen, Structural changes in C-S-H gel during dissolution: Small-angle neutron scattering and Si-NMR characterization, Cem. Concr. Res., 2015, 72, 76–89 CrossRef CAS.
- L. Black, K. Garbev, P. Stemmermann, K. R. Hallam and G. C. Allen, Characterisation of crystalline C-S-H phases by X-ray photoelectron spectroscopy, Cem. Concr. Res., 2003, 33, 899–911 CrossRef CAS.
- C. Ebbert, G. Grundmeier, N. Buitkamp, A. Kroger, F. Messerschmidt and P. Thissen, Toward a microscopic understanding of the calcium-silicate-hydrates/water interface, Appl. Surf. Sci., 2014, 290, 207–214 CrossRef CAS.
- H. Tsuji and S. Fujii, Phosphate recovery by generating hydroxyapatite via reaction of calcium eluted from layered double hydroxides, Appl. Clay Sci., 2014, 99, 261–265 CrossRef CAS.
- Z. Zyman, D. Rokhmistrov and V. Glushko, Structural changes in precipitates and cell model for the conversion of amorphous calcium phosphate to hydroxyapatite during the initial stage of precipitation, J. Cryst. Growth, 2012, 353, 5–11 CrossRef CAS.
- H. H. Pan, X. Y. Liu, R. K. Tang and H. Y. Xu, Mystery of the transformation from amorphous calcium phosphate to hydroxyapatite, Chem. Commun., 2010, 46, 7415–7420 RSC.
- S. Ding, D. Fang, Z. Pang, B. Luo, L. Kuang, H. Wang, Q. Zhang, Q. Shen and F. Ji, Immobilization of powdery calcium silicate hydrate via PVA covalent cross-linking process for phosphorus removal, Sci. Total Environ., 2018, 645, 937–945 CrossRef CAS PubMed.
- W. Guan, F. Y. Ji, Q. K. Chen, P. Yan and Q. Zhang, Preparation and phosphorus recovery performance of porous calcium-silicate-hydrate, Ceram. Int., 2013, 39, 1385–1391 CrossRef CAS.
- X. D. Cao and W. Harris, Carbonate and magnesium interactive effect on calcium phosphate precipitation, Environ. Sci. Technol., 2008, 42, 436–442 CrossRef CAS.
Footnote |
† Electronic supplementary information (ESI) available. See DOI: 10.1039/c8ew00643a |
|
This journal is © The Royal Society of Chemistry 2019 |
Click here to see how this site uses Cookies. View our privacy policy here.