Quantifying the efficacy of diquat dibromide in controlling Microcystis aeruginosa and Aphanizomenon flos-aquae in comparison to copper sulfate and potassium permanganate†
Received
6th August 2018
, Accepted 27th November 2018
First published on 28th November 2018
Abstract
Cyanobacterial harmful algal blooms (HABs) are an emerging problem worldwide, affecting many important freshwater systems. The use of chemical algaecides can provide an effective short-term mitigation measure to control HABs. In this study, the efficacy of diquat dibromide was examined under laboratory conditions to control two problematic toxin-releasing cyanobacteria, namely Aphanizomenon flos-aquae and Microcystis aeruginosa. Its performance was then compared to that of copper sulfate (CuSO4) and potassium permanganate (KMnO4), two commonly used algaecides. The results suggest that while all three algaecides were effective in controlling Aphanizomenon, the highest inhibition rates achieved were associated with the application of diquat dibromide. Aphanizomenon exhibited a half-life of 0.48 days with diquat dibromide dosages of 0.5 and 1 mg L−1. Both diquat dibromide and CuSO4 exhibited more effectiveness in controlling Microcystis aeruginosa as compared to KMnO4. Reductions achieved by applying 0.5 mg L−1 of diquat dibromide or 1 mg L−1 of CuSO4 exceeded 95% after 48 h of treatment. Nevertheless, diquat dibromide suppressed the net regrowth of Microcystis aeruginosa up to 70 h, while suppression with CuSO4 did not exceed 64 h even with the highest applied dosage. Irrespective of the algaecide and the application dosage, regrowth was observed for Microcystis aeruginosa but not for Aphanizomenon flos-aquae. Statistical models were proposed to simulate inhibition rates and estimate net algal regeneration.
Water impact
The results showed that diquat dibromide was more effective than commonly used algaecides in controlling toxin forming Microcystis aeruginosa and Aphanizomenon flos-aquae. Irrespective of the algaecide used and the dosage applied, Microcystis aeruginosa was able to reestablish itself over time.
|
1 Introduction
Anthropogenic eutrophication is a global problem affecting many important aquatic systems. Excessive nutrient loading has been a main driver for harmful algal blooms (HABs) proliferation.1–3 Cyanobacteria are the major algae type associated with the proliferation of HABs and impairments of freshwater systems.4–7Microcystis, Anabaenopsis, Aphanizomenon, Oscillatoria, Nostoc, and Planktothrix are some common genera that are known to form blooms and produce cyanotoxins.
Blooms of Micrococystis aeruginosa are a major concern, given that they can be associated with the release of microcystin, a known hepatotoxin.8,9Micrococystis blooms have been responsible for the impairment of important lake systems around the world, such as Lake Okeechobee in Florida,10 Lake Erie,11,12 and Lake Taihu in China.1,13 Another pervasive toxin producing cyanobacteria genera is Aphanizomenon flos-aquae. It is known to release cylindrospermopsins, a hepatoxin that can cause gastrointestinal damage,9,14,15 along with anatoxins and saxitoxin, which are known to affect neurological activities and can lead to death through respiratory paralysis.16,17 Several freshwater bodies in the Mediterranean region along with Lake Dianchi in China, Lake Erie, Utah Lake, and the Upper Klamath Lake in Oregon have been negatively affected by Aphanizomenon blooms.14,18–20
Mitigation measures have been tested to control excessive algal blooms including algaecides applications, mechanical mixing, food web manipulations, and/or nutrient regulation.21–30 Nutrient enrichment has been strongly linked to increased eutrophication and the stimulation of harmful algal blooms.3 Examples of the success of nutrient management in reducing HAB events include the decline of HAB events in Lake Washington and the Potomac River following the removal of sewage discharges into these systems.31,32 Similar successes have also been reported in Lake Erie, where improved wastewater treatment and the ban on phosphate detergents in the early 1980s significantly reduced HAB events.33 In 2003, the US Environmental Protection Agency concluded that managing nutrient inputs to the watershed can lead to significant reduction in HABs.34 Nevertheless, there is evidence to show that the overall effect of nutrient load reductions on harmful algal proliferation tends to be species specific.3 While controlling nutrients emerging from point and non-point sources at the river-basin level remains the most effective approach on the long term, effective implementation is often hindered by socio-economic constraints and/or lack of regulatory enforcement. As a result, short-term mitigation measures are needed. Of these measures, algaecides application remains the most commonly adopted.
Copper sulfate (CuSO4) remains to date the most commonly used algaecide in freshwater systems.35–37 It has been in use since the early 1900s, with early application recorded in the Fairmont lakes.38,39 Its wide use was promoted by the fact that it is inexpensive, accessible, and effective against cyanobacteria.5,40–44 It acts by interrupting electron transport through photosystem II, increasing oxidative stress, and competing with magnesium in the chlorophyll molecule.35,45–47 Potassium permanganate (KMnO4) is another commonly used algaecide that was promoted by its low environmental hazard.48–53 Its use has been documented across many freshwater systems including storm-water retention lakes in Canada, fish ponds in the United States, Lake Biwa in Japan, and to lakes and ponds in China.54–58 KMnO4 is an oxidant that releases hydrated manganese dioxide (MnO2), which stimulates oxidation-enhanced coagulation of surface algae cells.59–62 A less commonly used algaecide is diquat dibromide (IUPAC name: 6,7-dihydrodipyrido(1,2-a:2′,1′-c)pyrazinediium dibromide), which has been commercially available since the early 1950s.63,64 It was registered with the United States Environmental Protection Agency (USEPA) in 198665 and shown to be effective on a wide spectrum of cyanobacteria.64,66–70 It disrupts the electron transport in photosynthetic tissues by changing nicotinamide adenine dinucleotide phosphate (NADP).71,72
In this study, we examined the efficacy of diquat dibromide in controlling Microcystis aeruginosa and Aphanizomenon flos-aquae blooms through a series of laboratory-based inhibition tests conducted under different dosages. Efficacy was determined in terms of the measured decrease in chlorophyll-a concentrations over 96 hours of the inhibition test. The performance of diquat dibromide was then compared to that of CuSO4 and KMnO4. Mathematical decay models were then developed to quantify the net change in cyanobacteria over time for the three algaecides using the measured chlorophyll-a concentrations. The adopted decay models permit a temporally variable rate of change. A positive net change value indicates a net regrowth of the algae, while a negative value represents a net drop in their levels. The residual concentrations of the three algaecides at the end of the 4 day experiment were measured and compared to the World Health Organization drinking water quality standards.8,16
2 Materials and methodolgy
2.1 Sample collection
Surface water samples were collected 10 cm below the water surface of the Qaraoun reservoir, Lebanon's largest freshwater body (Fig. 1). The reservoir is hypertrophic due to excessive point and non-point pollution loading.73,74Microcystis blooms have been consistently occurring over the summer season, while Aphanizomenon blooms tend to occur between late winter and early summer.75 Cyanobacteria were collected from the reservoir during algal bloom events. Blooms are often characterized by the dominance of a single nuisance algal community.76–78 Collected samples were transported to the lab on ice and observed under the microscope to assess the dominance of the targeted species. Microscopic observations were repeated throughout the culturing (2 weeks) and inhibition tests (5 days) to ensure that no other algae type other than the one targeted was observable under the microscope. In the event that multiple species were observed, the entire batch was discarded and the inhibition tests were aborted.
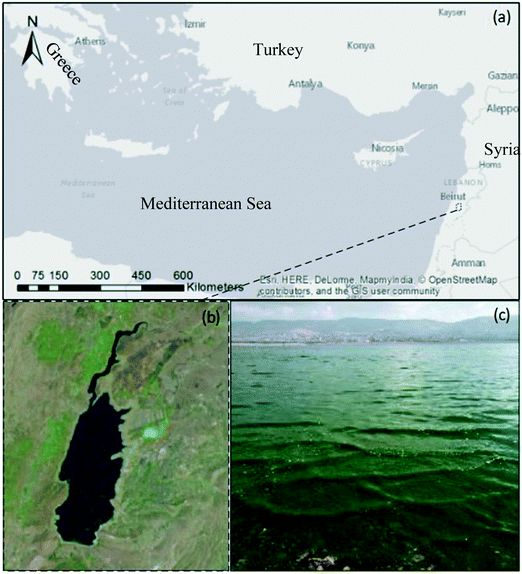 |
| Fig. 1 (a) Geographical location of the Qaraoun reservoir; (b) a Landsat 8 satellite image on July 4, 2013 showing from space a developing cyanobacteria bloom; and (c) an image captured during a Microcystis aeruginosa bloom observed on September 22, 2015. | |
2.2 Cyanobacteria culturing
Collected cyanobacteria water samples were cultured under laboratory conditions in 20 L glass containers under a 12
:
12 light
:
dark cycle with a light intensity ∼74 Lux. Cultures were enriched with nutrients, air bubbled to enhance mixing and ensure no carbon limitation, and maintained at 25 °C water temperature for Microcystis and 21 °C for Aphanizomenon.79 In an effort to ensure no nutrient limitation, an F/2 medium based on the Guillard's formulation80 of essential nutrients was used. Samples were routinely sub-cultured to achieve a cell density >1.0 × 106 cells per ml; this ensures that the algae maintains the logarithmic growth phase.79 For the algaecide inhibition experiments, the algae were extracted and diluted by adding filtered lake water to reach ∼10
000 cells per ml.79 The cell density was determined with a hemocytometer using a fluorescence microscope. Note that the culturing of the cyanobacteria under laboratory conditions guaranteed that: 1) all experiments were run with monocultures; 2) the algae were acclimatized and able to achieve a logarithmic growth phase prior to exposing them to the algaecides; and 3) that all experiments were started with the same initial cell density.
2.3 Experimental procedures
Selected algaecide concentrations represented concentrations previously used for algae control. All tested algaecide concentrations were prepared using reagent grade quality and ultra-pure water freshly provided by a water filter system (MilliQ, Millipore). Diquat dibromide‡ was prepared from analytical standard grade diquat dibromide monohydrate (SUPELCO N11816). Its efficacy was tested under two concentrations, namely 0.5 and 1 mg L−1 as diquat. High-performance liquid chromatography (HPLC) with a C18 column (provided by SUPELCO Z226033) was used to measure diquat concentrations based on USEPA method 549.2.81 When diquat concentrations were below the detection limit (<0.72 μg L−1), a solid-phase extraction81 was carried out with C8 (500 mg)–6 ml cartridges obtained from SUPELCO (52714-U). All solvents for the mobile phase and extraction procedure were HPLC grade. For CuSO4 (Sigma Aldrich 209198 ACS reagents) and KMnO4 (Merck and Co. M5080), stock solutions were used to achieve the desired chemical dosages (0.2, 0.5, 0.8, and 1 mg L−1 as CuSO4 and 1, 2, and 3 mg L−1 as KMnO4) following USEPA method 200.9.82
The experimental chambers for inhibition tests were 250 ml Erlenmeyer flasks with a cyanobacteria density of ∼10
000 cells per ml. All experiments were conducted according to the USEPA method with all chambers irradiated by florescent lamps providing 4306 Lux.83 Triplicate tests were run for each algaecide dose. In addition, cyanobacterial concentrations were monitored in three control flasks. The duration of the inhibition test was set to 96 h, with the analysis of 50 ml samples collected every 24 h. Daily analysis included pH, DO, temperature, chlorophyll-a, and cell counts. The physio-chemical analysis for the Microcystis and Aphanizomenon tests are summarized in Tables S1 and S2 in the ESI.†
Chlorophyll-a concentrations were measured by collecting samples with known volumes buffered using magnesium carbonate before being filtered through membrane filter papers. They were stored overnight at −20 °C. Chlorophyll-a was extracted in liquid chromatography grade acetone (90%) solution followed by sonification. Extracts were seeped in the acetone solution overnight and then clarified using centrifugation. Chlorophyll-a concentrations were evaluated based on absorbance readings (Standard Method 10200 (HS2))84 on a HACH DR3900 spectrophotometer.85 For cell counts and cell density, cells of Aphanizomenon flos-aquae and Microcystis aeruginosa were microscopically monitored using a Zeiss Fluorescence microscope (Axiovert 200) with an improved hemocytometer (Marienfeld), with emphasis on recording whether they were colonial or unicellular. Chlorophyll-a concentrations were used as the response endpoint to test algaecide potency. Calomeni and Rodgers86 identified chlorophyll-a as a suitable surrogate to assess algal viability.
2.4 Statistical analysis
The effect of dosage and potential changes in efficiency over time were assessed across the two cyanobacteria species. Two-way analysis of variance (ANOVA) tests were used to evaluate the potential for an interaction between dose and time. In the event that the 2-way ANOVA showed statistical significance, Tukey Honest significant differences multiple comparisons were used. Note that chlorophyll-a concentration of Microcystis and Aphanizomenon were square root-transformed to achieve normality. ANOVA and multiple comparisons analysis were conducted in the R software.87
Dose-dependent algaecide specific cyanobacterial decay models were developed to predict the rate of change in chlorophyll-a concentrations as a function of time (measured in hours) for the two cyanobacteria. Two decay model structures were considered for this purpose. The first modeled the drop in chlorophyll-a concentrations as a first order exponential decay (eqn (1)), while the second was based on a second order polynomial model (eqn (2)). Note that the later model structure allows for the occurrence of an inflection point beyond which regrowth surpasses the residual algaecidal inhibition. The adopted quadratic concave formulation has been reported to account for decreasing proportional pressure, which can ultimately lead to net regrowth.88 The exponential decay model was fist linearized and both models were fit using linear regression that minimizes the sum of squared errors. The adjusted R2 (Radj2) was used as a measure of model fit. Models were fit using the linear model lm function in R.87
| 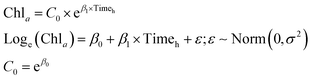 | (1) |
| Chla = β0 + β1 × Timeh + β2 × Timeh2 + ε; ε ∼ Norm(0, σ2) | (2) |
where Chl
a is the measured chlorophyll-
a concentration in μg L
−1 over time,
C0 is the initial chlorophyll-
a concentration at time
t = 0, Time is time in hours following algaecide application,
β0 represents the intercept of the model,
β1−2 are the slopes on the predictors,
ε is the model error term, and
σ is the standard error of the model. The predictive model uncertainties were quantified using a Monte Carlo simulation in the “
arm” package in R.
89 Note that the use of Monte Carlo simulations helps address re-transformation biases.
90
3 Results
3.1 Descriptive statistics
3.1.1 Diquat.
Diquat was highly effective in controlling both cyanobacterial species under the tested dosages. During the first 24 h, Microcystis colonies were no longer visible under the microscope for both the 0.5 and 1 mg L−1 applications. Yet after the first 24 h, chlorophyll-a concentrations were still around 50 to 65% of their original levels for both dosages. After 48 h of treatment, unicellular Microcystis became hard to observe and inhibition approached 100%. Recovery of some unicellular cells was observed after 96 h, with measured chlorophyll-a concentrations reaching 17 and 11% of their initial concentrations for the 0.5 and 1 mg L−1 doses respectively (Fig. 2a). Measured chlorophyll-a concentrations at 48, 72, and 96 h were found to be statistically similar indicating that the net regrowth of Microcystis was slow.
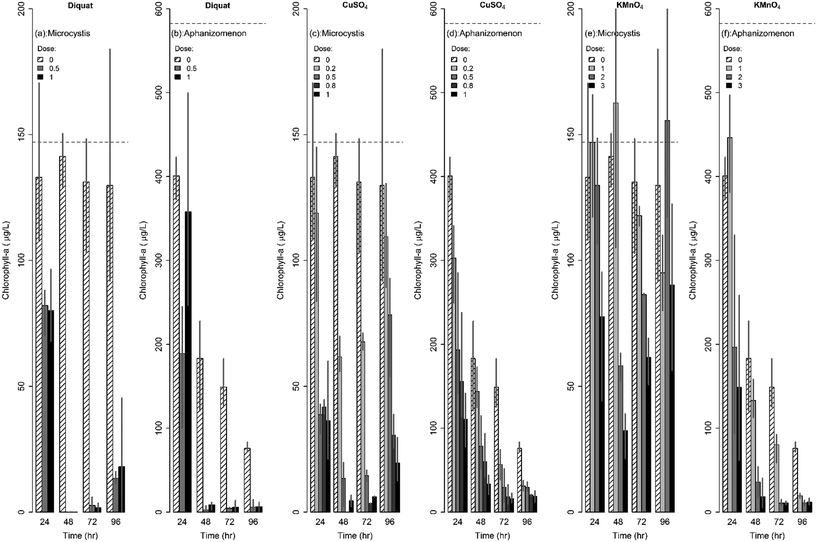 |
| Fig. 2 Changes in chlorophyll-a concentrations following algaecide treatment for Microcystis and Aphanizomenon. | |
Similarly, the effect of diquat on Aphanizomenon colonies was immediate with colonies disappearing after 24 h; the drop in chlorophyll-a concentrations ranged between 40% and 68% in the first 24 h. Nevertheless, the inhibiting action of diquat accelerated in the next 24 h whereby chlorophyll-a concentrations dropped by more than 95 to 99% after 48 to 72 h of treatment (Fig. 2b). Chlorophyll concentrations achieved at 48, 72, and 96 h were statistically similar indicating a lack of net regrowth. Note that while previous work reported 100% inhibition of Aphanizomenon at diquat dosages in excess of 0.75 mg L−1,66 our results indicated that complete inhibition can occur at a lower dosages (0.5 mg L−1).
3.1.2 CuSO4.
CuSO4 proved to be effective in controlling Microcystis across all dosages. Microscopic observations showed that Microcystis colonies broke down into individual cells within the first 24 h. Chlorophyll-a concentrations decreased on average by 20% at the lowest tested dose of 0.2 mg L−1 (Fig. 2c). Yet, reductions exceeded 75% after 24 h of treatment at higher dosages (0.5, 0.8, and 1 mg L−1). These results show that CuSO4 has a faster inhibition action as compared to the diquat concentrations used. After 48 h of being subjected to the highest dose (1 mg L−1), chlorophyll-a concentrations dropped to 5% of their original values. Similar removal efficiencies were reported by Fan et al.91 The net regrowth of Microcystis following CuSO4 application occurred earlier than diquat. Recovery following CuSO4 treatment started at 72 h versus 96 h for diquat. Moreover, the recovery rate of Microcystis following CuSO4 treatment was higher than that observed for diquat, with measured concentrations at 96 h statistically similar to those observed at 24 h (p-value = 0.99).
CuSO4 was also found to be potent in controlling Aphanizomenon flos-aquae; yet its efficacy was lower than that of diquat (Fig. 2). Under microscopic observations, the decolonization of Aphanizomenon was apparent following the first day of treatment. Reductions after 48 h of contact time did not exceed 75% of the original chlorophyll-a concentration at the lowest tested dose (0.2 mg L−1). At higher dosages, reductions reached 87%, 90% and 95% after 48 h of contact to 0.5, 0.8, and 1 mg L−1 respectively. No regrowth was evident across all tested dosages (Fig. 2d).
3.1.3 KMnO4.
KMnO4 was less effective than diquat and CuSO4 in controlling Microcystis. Overall, measured chlorophyll-a concentrations following the application at the two lower dosages (1 and 2 mg L−1) were found to be statistically similar to the control (p-values = 0.99 and 0.11 respectively). At the highest dosage of 3 mg L−1, the removal rate after 48 h did not exceed 80% on average (Fig. 2e). Chlorophyll-a concentrations appeared to rebound after 72 h and on day 4 (96 h) were statistically similar to levels in the control across all dosages (Fig. 2e). While KMnO4 proved to be ineffective in controlling Microcystis, it proved to be a good inhibitor of Aphanizomenon. Colonies of Aphanizomenon declined significantly even after 24 h of treatment across all dosages. Dosages of 2 mg L−1 and higher achieved removals in excess of 98% at 72 h. Previous studies suggested the need for a higher (5 mg L−1) dosage for controlling Aphanizomenon.57 Reductions in chlorophyll-a concentrations of more than 90% were observed after 48 h of treatment with KMnO4 dosages of 2 and 3 mg L−1. Both dosages achieved more than 98% reductions at 96 h (Fig. 2f). No net regrowth was apparent, with measured chlorophyll-a levels largely stable at 72 and 96 h (p-value = 0.17).
3.2 Modeling algaecidal potency
3.2.1
Microcystis
.
One the main features observed in this study was the ability of Microcystis to recover and regrow following algaecide application. Recovery rates and timing varied across dosages and algaecide type. The recovery of Microcystis aeruginosa post algaecide application was reported in previous studies.40,92 Yet, the dynamics of regrowth have not been discussed nor modeled. The proposed quadratic model proved to capture the dynamics of Microcystis aeruginosa, especially under high algaecide dosages (Fig. 3). Yet, an artifact of the quadratic model is its potential to predict concentrations lower than zero at high dosages. This was evident in the case of diquat and CuSO4 (Fig. 3).
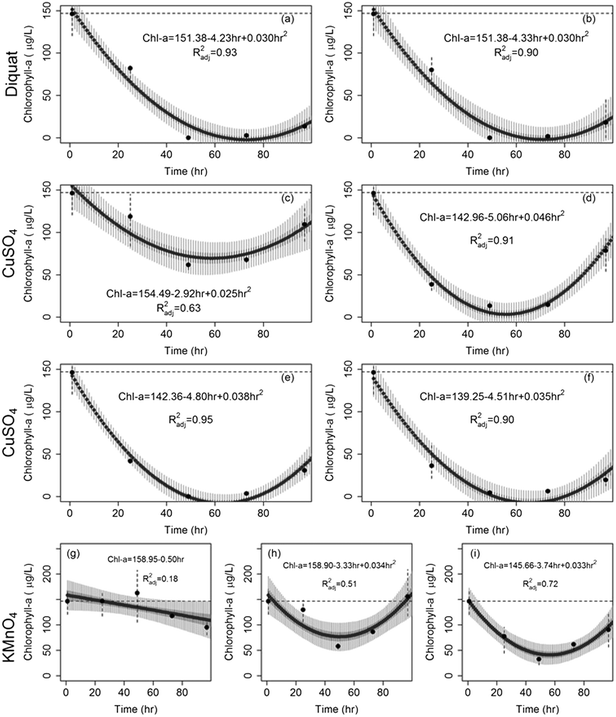 |
| Fig. 3 Drop in chlorophyll-a concentration of Microcystis after the application of (a) diquat = 0.5 mg L−1, (b) diquat = 1 mg L−1, (c) CuSO4 = 0.2 mg L−1, (d) CuSO4 = 0.5 mg L−1, (e) CuSO4 = 0.8 mg L−1, (f) CuSO4 = 1 mg L−1, (g) KMnO4 = 1 mg L−1, (h) KMnO4 = 2 mg L−1, and (i) KMnO4 = 3 mg L−1. The dashed horizontal line shows initial conditions; solid circles represent median concentrations; dashed vertical lines show the range of observed concentrations. Solid thick vertical grey segments and solid light grey vertical segments represent the 50% and 95% model predicted chlorophyll-a concentrations respectively. | |
The rates at which Microcystis concentrations dropped across the three algaecides under the different dosages are shown in Fig. 4. Diquat had the lowest Microcystis recovery rate among all tested algaecides, thus potentially requiring the least frequent application (Fig. 4a). Net regrowth tended to occur at least after 70 h of treatment. As for CuSO4, the model predicted that regrowth will exceed inhibition between 55 and 64 h following treatment across the four tested dosages (Fig. 4b). Given the fact that Microcystis aeruginosa exhibited resilience against KMnO4 treatment at the lower dosages, it was difficult to separate the inhibition phase from regrowth. This manifested in the absence of a second order regression term in the algae dynamics model for the 1 mg L−1 dose (Fig. 4c). At the 2 and 3 mg L−1 dosages, the KMnO4 treatment did show evidence of an initial net inhibition phase that was followed by a new recovery phase (Fig. 3 and 4c). Clearly, the use of KMnO4 is not suitable for the control of Microcystis aeruginosa blooms.
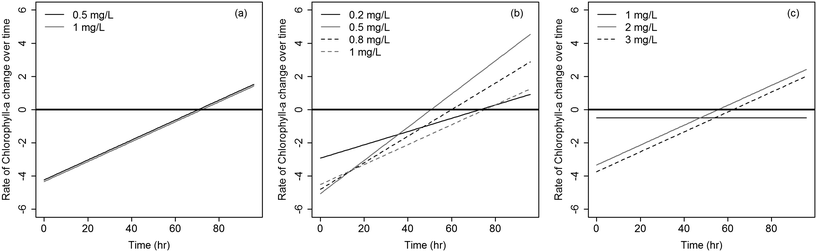 |
| Fig. 4 Rates of inhibition and recovery of Microcystis over time for (a) diquat; (b) CuSO4; and (c) KMnO4. Rates below the solid black horizontal line (y = 0) indicates dominance of inhibition with a net decrease in concentrations, while rates above (y = 0) represent dominant regrowth dynamics with a net increase in concentrations. | |
3.2.2
Aphanizomenon
.
Given the absence of net regrowth following treatment across the three tested algaecides, the first order decay models were suitable to explain the change in Aphanizomenon over time (Fig. 5). Diquat exhibited the highest chlorophyll-a decay rate of all three algaecides, with a rate of 1.44 per day for both dosages. The decay rate for KMnO4 was 0.96 per day across the three tested dosages, while the rate for CuSO4 ranged between 0.72 and 0.96 per day depending on the dosage used. Thus while the applied dosages of KMnO4 and CuSO4 proved to be effective in suppressing the Aphanizomenon levels, the use of diquat was able to achieve a similar suppression in a shorter time interval. As can be seen in Fig. 5, the adopted first order decay models had a tendency to underestimate the initial drop in chlorophyll-a concentrations across the applied diquat dosages. This was also true under the high dosages of CuSO4 and KMnO4. The mismatch between the model and the data indicates that the initial (first 48 hours) removal rates appear to be faster than what can be predicted by the first order decay model, which assumes that the rate is temporally invariant.
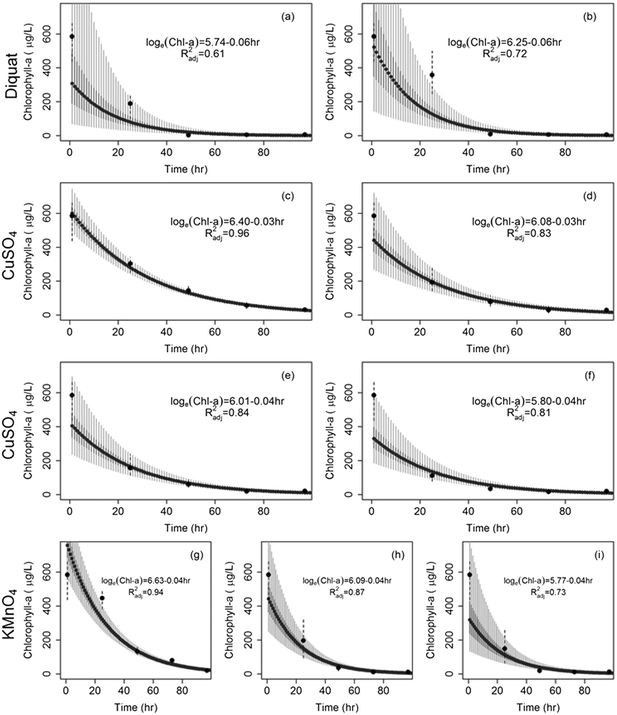 |
| Fig. 5 Drop in chlorophyll-a concentration of Aphanizomenon after the application of (a) diquat = 0.5 mg L−1, (b) diquat = 1 mg L−1, (c) CuSO4 = 0.2 mg L−1, (d) CuSO4 = 0.5 mg L−1, (e) CuSO4 = 0.8 mg L−1, (f) CuSO4 = 1 mg L−1, (g) KMnO4 = 1 mg L−1, (h) KMnO4 = 2 mg L−1, and (i) KMnO4 = 3 mg L−1. The dashed horizontal line represents initial conditions; black solid circles represent average measured concentrations; dashed vertical lines show the range of observed concentration (minimum and maximum). Solid thick vertical grey segments and solid light grey vertical segments represent the 50% and 95% model predicted chlorophyll-a concentrations respectively. | |
4 Conclusions
This study established the efficacy of diquat as an efficient chemical algaecide that can be used to control two important nuisance cyanobacteria that are known to form HABs and produce toxins. The results showed that diquat and CuSO4 outperformed KMnO4 in controlling Microcystis aeruginosa and both were able to inhibit and retard its regrowth. Microcystis aeruginosa levels after 72 h of algaecidal treatment with the highest tested dosage of diquat (1 mg L−1) were found to be statistically lower that the levels achieved with the highest tested dose of CuSO4 (1 mg L−1). Microcystis levels after 96 h of treatment rebounded across all algaecides. Yet, the lowest average chlorophyll-a concentrations achieved after 96 h of contact time with the highest dosage across the three algaecides was found to be associated with diquat (8.9 μg L−1 of chlorophyll-a as compared to 16.4 and 92.1 μg L−1 for CuSO4 and KMnO4 respectively); yet these levels were not found to be statistically different from the levels achieved with the 1 mg L−1 CuSO4 treatment at the 95% confidence level due to variability observed between the triplicates. KMnO4 proved to be ineffective in controlling Microcystis; yet it performed satisfactory with Aphanizomenon. While the applied dosages of KMnO4 and CuSO4 proved to be effective in suppressing Aphanizomenon, the use of diquat was able to achieve similar suppressions in a shorter time interval. As such, the results underscore the need for proper algae characterization during a bloom event prior to algaecide selection and application. While the efficacy of diquat has been shown to vary by species (e.g.ref. 64 and 68), previous studies have shown that CuSO4 tends to have a broad spectrum of action.40,93,94 Changes in diversity can have a cascading effect on the freshwater food web.35 The study also showed that the algaecidal impact on Microcystis aeruginosa was best described by a concave quadratic decay model that is capable of capturing the effect of decreasing proportional pressures. This is in contrast to the first order decay model structure that to a large extent captured the Aphanizomenon flos-aquae dynamics over time.
Note that this study did not explore the confounding effects that ambient environmental conditions may have on the effectiveness of the three tested algaecides. In the case of diquat, the presence of high levels of suspended particulates in the water column can cause diquat to bind to these sediments, reducing its bioavailability.63,95 For CuSO4, the degree of chelation in the water column can affect its residence time and thus may change its efficacy.42,43,96,97 As for KMnO4, increased hardness of the water has been linked to better algal removal rates.59 Future work should also examine the scalability and transferability of the results to the lake level, where algal densities can be more than two orders of magnitude higher (∼1–2 million cells per ml) than those set by the EPA algal inhibition test guidelines.83
While algaecide applications were shown to be effective, in the absence of real mitigation measures that aim to reduce the true promoters of algal blooms, repeated application of algaecides may result in reduced efficacy over time due to the development of algaecide-resistant mutant strains. Several studies have reported the development of resistant strains of nuisance algal species following exposure to water contaminants.46,98,99 As such, initiating an algaecide application program in a given water body should only be a short-term measure that cannot be a permanent replacement of enacting long-lasting watershed-level management actions. Moreover, while there is no reported health concerns regarding the residual levels of the algaecides in the treated water, the possibility of toxin release upon cell lysis following the use of algaecides is a serious concern that needs attention.92,100,101 Tests conducted on the residuals levels of copper, permanganate, and diquat after 96 h of treatment (summarized in the online ESI†), showed that all concentrations, irrespective of dose, were within the standards set for drinking water.8 Yet, the long-term ecotoxicity associated with these residual levels of algaecides may be of concern. Ecological stresses have been documented even at low dosages of copper102,103 and diquat.104
Conflicts of interest
There are no conflicts to declare.
Acknowledgements
This study was supported through the United States Agency for International Development through the USAID-NSF PEER initiative (grant # AID-OAA-A-I1-00012) and the American University of Beirut University Research Board. The manuscript was extracted from the thesis of Sara Dia titled “Efficacy of three algaecides on algal blooms in hypereutrophic lakes” that was submitted to the American University of Beirut in fulfillment of the requirements of her MS Degree on June 2016 (https://scholarworks.aub.edu.lb/handle/10938/11127). A presentation of the work was conducted at the Water Pollution 2016 conference, Venice, Italy.
References
- H. W. Paerl and J. T. Scott, Throwing fuel on the fire: synergistic effects of excessive nitrogen inputs and global warming on harmful algal blooms, Environ. Sci. Technol., 2010, 44, 7756–7758 CrossRef CAS PubMed.
- M. Leira, G. Chen, C. Dalton, K. Irvine and D. Taylor, Patterns in freshwater diatom taxonomic distinctness along an eutrophication gradient, Freshwater Biol., 2009, 54, 1–14 CrossRef CAS.
- D. M. Anderson, P. M. Glibert and J. M. Burkholder, Harmful algal blooms and eutrophication: nutrient sources, composition, and consequences, Estuaries, 2002, 25, 704–726 CrossRef.
- G. M. Hallegraeff, A review of harmful algal blooms and their apparent global increase, Phycologia, 1993, 32, 79–99 CrossRef.
-
I. Chorus and J. Bartram, Toxic cyanobacteria in water: A guide to their public health consequences, monitoring and management, Spon Press, 1999 Search PubMed.
- J. Heisler, P. M. Glibert, J. M. Burkholder, D. M. Anderson, W. Cochlan, W. C. Dennison, Q. Dortch, C. J. Gobler, C. A. Heil and E. Humphries, Eutrophication and harmful algal blooms: a scientific consensus, Harmful Algae, 2008, 8, 3–13 CrossRef CAS PubMed.
-
H. K. Hudnell, Cyanobacterial harmful algal blooms: state of the science and research needs, Springer Science & Business Media, 2008 Search PubMed.
-
WHO, Guidelines for drinking-water quality: recommendations, World Health Organization, 2004 Search PubMed.
- I. R. Falconer, Is there a human health hazard from microcystins in the drinking water supply?, Acta Hydrochim. Hydrobiol., 2005, 33, 64–71 CrossRef CAS.
- J. Beaver and K. Havens, Seasonal and spatial variation in zooplankton community structure and their relation to possible controlling variables in Lake Okeechobee, Freshwater Biol., 1996, 36, 45–56 CrossRef.
- M. A. Doblin, K. J. Coyne, J. M. Rinta-Kanto, S. W. Wilhelm and F. C. Dobbs, Dynamics and short-term survival of toxic cyanobacteria species in ballast water from NOBOB vessels transiting the Great Lakes—implications for HAB invasions, Harmful Algae, 2007, 6, 519–530 CrossRef CAS.
- A. M. Michalak, E. J. Anderson, D. Beletsky, S. Boland, N. S. Bosch, T. B. Bridgeman, J. D. Chaffin, K. Cho, R. Confesor and I. Daloğlu, Record-setting algal bloom in Lake Erie caused by agricultural and meteorological trends consistent with expected future conditions, Proc. Natl. Acad. Sci. U. S. A., 2013, 110, 6448–6452 CrossRef CAS.
- H. W. Paerl and T. G. Otten, Harmful cyanobacterial blooms: causes, consequences, and controls, Microb. Ecol., 2013, 65, 995–1010 CrossRef CAS PubMed.
- E. Vardaka, M. Moustaka-Gouni, C. M. Cook and T. Lanaras, Cyanobacterial blooms and water quality in Greek waterbodies, J. Appl. Phycol., 2005, 17, 391–401 CrossRef.
- I. Ohtani, R. E. Moore and M. T. Runnegar, Cylindrospermopsin: a potent hepatotoxin from the blue-green alga Cylindrospermopsis raciborskii, J. Am. Chem. Soc., 1992, 114, 7941–7942 CrossRef CAS.
-
WHO, Guidelines for drinking-water quality, World Health Organization, 2011, vol. 216, pp. 303–304 Search PubMed.
- H. W. Paerl, R. S. Fulton, P. H. Moisander and J. Dyble, Harmful freshwater algal blooms, with an emphasis on cyanobacteria, Sci. World J., 2001, 1, 76–113 CrossRef CAS PubMed.
- Y. Liu, W. Chen, D. Li, Y. Shen, G. Li and Y. Liu, First report of aphantoxins in China—waterblooms of toxigenic Aphanizomenon flos-aquae in Lake Dianchi, Ecotoxicol. Environ. Saf., 2006, 65, 84–92 CrossRef CAS PubMed.
- A. E. Strong, Remote sensing of algal blooms by aircraft and satellite in Lake Erie and Utah Lake, Remote Sens. Environ., 1974, 3, 99–107 CrossRef.
- W. W. Carmichael, C. Drapeau and D. M. Anderson, Harvesting of Aphanizomenon flos-aquae Ralfs ex Born. & Flah. Var. flos-aquae (Cyanobacteria) from Klamath Lake for human dietary use, J. Appl. Phycol., 2000, 12, 585–595 CrossRef.
- J. Huisman, P. van Oostveen and F. J. Weissing, Species dynamics in phytoplankton blooms: incomplete mixing and competition for light, Am. Nat., 1999, 154, 46–68 CrossRef.
- J. Huisman, J. Sharples, J. M. Stroom, P. M. Visser, W. E. A. Kardinaal, J. M. Verspagen and B. Sommeijer, Changes in turbulent mixing shift competition for light between phytoplankton species, Ecology, 2004, 85, 2960–2970 CrossRef.
- P. Visser, B. Ibelings, B. Van Der Veer, J. Koedood and R. Mur, Artificial mixing prevents nuisance blooms of the cyanobacterium Microcystis in Lake Nieuwe Meer, the Netherlands, Freshwater Biol., 1996, 36, 435–450 CrossRef.
- B. W. Ibelings, B. Kroon and L. R. Mur, Acclimation of photosystem II in a cyanobacterium and a eukaryotic green alga to high and fluctuating photosynthetic photon flux densities, simulating light regimes induced by mixing in lakes, New Phytol., 1994, 128, 407–424 CrossRef CAS.
- V. H. Smith and D. W. Schindler, Eutrophication science: where do we go from here?, Trends Ecol. Evol., 2009, 24, 201–207 CrossRef PubMed.
-
E. Jeppesen, J. Peder Jensen, M. Søndergaard, T. Lauridsen, L. Junge Pedersen and L. Jensen, in Shallow Lakes 95: Trophic Cascades in Shallow Freshwater and Brackish Lakes, ed. L. Kufel, A. Prejs and J. I. Rybak, Springer Netherlands, Dordrecht, 1997, pp. 151–164, DOI:10.1007/978-94-011-5648-6_17.
- M. T. Dokulil and K. Teubner, Cyanobacterial dominance in lakes, Hydrobiologia, 2000, 438, 1–12 CrossRef CAS.
- J. J. Elser, The pathway to noxious cyanobacteria blooms in lakes: the food web as the final turn, Freshwater Biol., 1999, 42, 537–543 CrossRef.
- A. Herrero, A. M. Muro-Pastor and E. Flores, Nitrogen control in cyanobacteria, J. Bacteriol., 2001, 183, 411–425 CrossRef CAS.
- T. D. Geer, A. J. Calomeni, C. M. Kinley, K. J. Iwinski and J. H. Rodgers, Predicting In Situ Responses of Taste-and Odor-Producing Algae in a Southeastern US Reservoir to a Sodium Carbonate Peroxyhydrate Algaecide Using a Laboratory Exposure-Response Model, Water, Air, Soil Pollut., 2017, 228, 53 CrossRef.
- W. Edmondson, Phosphorus, nitrogen, and algae in Lake Washington after diversion of sewage, Science, 1970, 169, 690–691 CrossRef CAS.
- N. Jaworski, Retrospective study of the water-quality issues of the upper potomac estuary, Rev. Aquat. Sci., 1990, 3, 11–40 Search PubMed.
-
W. Ashworth, The Late, Great Lakes: An Environmental History, Wayne State University Press, 1986, ISBN-10: 0814318878 Search PubMed.
- J. Heisler, P. M. Glibert, J. M. Burkholder, D. M. Anderson, W. Cochlan, W. C. Dennison, Q. Dortch, C. J. Gobler, C. A. Heil, E. Humphries, A. Lewitus, R. Magnien, H. G. Marshall, K. Sellner, D. A. Stockwell, D. K. Stoecker and M. Suddleson, Eutrophication and harmful algal blooms: A scientific consensus, Harmful Algae, 2008, 8, 3–13 CrossRef CAS.
- D. Jančula and B. Maršálek, Critical review of actually available chemical compounds for prevention and management of cyanobacterial blooms, Chemosphere, 2011, 85, 1415–1422 CrossRef PubMed.
- L. E. Brand, W. G. Sunda and R. R. Guillard, Reduction of marine phytoplankton reproduction rates by copper and cadmium, J. Exp. Mar. Biol. Ecol., 1986, 96, 225–250 CrossRef CAS.
- A.-H. Le Jeune, M. Charpin, V. Deluchat, J.-F. Briand, J.-F. Lenain, M. Baudu and C. Amblard, Effect of copper sulphate treatment on natural phytoplanktonic communities, Aquat. Toxicol., 2006, 80, 267–280 CrossRef CAS PubMed.
- M. J. Hanson and H. G. Stefan, Side effects of 58 years of copper sulfate treatment of the Fairmont Lakes, Minnesota, J. Am. Water Resour. Assoc., 1984, 20, 889–900 CrossRef CAS.
-
G. T. Moore and K. F. Kellerman, Copper as an algicide and disinfectant in water supplies, US Government Printing Office, 1905 Search PubMed.
- J. Fan, L. Ho, P. Hobson and J. Brookes, Evaluating the effectiveness of copper sulphate, chlorine, potassium permanganate, hydrogen peroxide and ozone on cyanobacterial cell integrity, Water Res., 2013, 47, 5153–5164 CrossRef CAS PubMed.
- J. F. Elder and A. J. Horne, Copper cycles and CuSO4 algicidal capacity in two California lakes, Environ. Manage., 1978, 2, 17–30 CrossRef CAS.
- A. Calomeni, J. H. Rodgers and C. M. Kinley, Responses of Planktothrix agardhii and Pseudokirchneriella subcapitata to Copper Sulfate (CuSO4· 5H2O) and a Chelated Copper Compound (Cutrine®-Ultra), Water, Air, Soil Pollut., 2014, 225, 2231 CrossRef.
- K.-P. Tsai, Management of Target Algae by Using Copper-Based Algaecides: Effects of Algal Cell Density and Sensitivity to Copper, Water, Air, Soil Pollut., 2016, 227, 238 CrossRef.
- E. A. Crafton, J. Glowczewski, D. W. Ott and T. J. Cutright, In situ field trial to evaluate the efficacy of Cutrine Ultra to manage a cyanobacteria population in a drinking water source, Environ. Sci.: Water Res. Technol., 2018, 4, 863–871 RSC.
- P. Pandey, C. Singh and S. Singh, Cu uptake in a cyanobacterium: fate of selected photochemical reactions, Curr. Microbiol., 1992, 24, 35–39 CrossRef CAS.
- L. Garcia-Villada, M. Rico, M. Altamirano, L. Sánchez-Martin, V. López-Rodas and E. Costas, Occurrence of copper resistant mutants in the toxic cyanobacteria Microcystis aeruginosa: characterisation and future implications in the use of copper sulphate as algaecide, Water Res., 2004, 38, 2207–2213 CrossRef CAS PubMed.
- M. Gledhill, M. Nimmo, S. J. Hill and M. T. Brown, The toxicity of copper (II) species to marine algae, with particular reference to macroalgae, J. Phycol., 1997, 33, 2–11 CrossRef CAS.
- T. Hall, J. Hart, B. Croll and R. Gregory, Laboratory-scale investigations of algal toxin removal by water treatment, Water Environ. J., 2000, 14, 143–149 CrossRef CAS.
- J.-J. Chen, H.-H. Yeh and I.-C. Tseng, Effect of ozone and permanganate on algae coagulation removal–Pilot and bench scale tests, Chemosphere, 2009, 74, 840–846 CrossRef CAS PubMed.
- P. Roccaro, C. Barone, G. Mancini and F. Vagliasindi, Removal of manganese from water supplies intended for human consumption: a case study, Desalination, 2007, 210, 205–214 CrossRef CAS.
- H. Kemp, R. Fuller and R. Davidson, Potassium permanganate as an algicide, Journal, 1966, 58, 255–263 CAS.
- D. Ellis, C. Bouchard and G. Lantagne, Removal of iron and manganese from groundwater by oxidation and microfiltration, Desalination, 2000, 130, 255–264 CrossRef CAS.
- E. Rodríguez, G. D. Onstad, T. P. Kull, J. S. Metcalf, J. L. Acero and U. von Gunten, Oxidative elimination of cyanotoxins: comparison of ozone, chlorine, chlorine dioxide and permanganate, Water Res., 2007, 41, 3381–3393 CrossRef PubMed.
- J. Ma and W. Liu, Effectiveness and mechanism of potassium ferrate (VI) preoxidation for algae removal by coagulation, Water Res., 2002, 36, 871–878 CrossRef CAS PubMed.
- H. Zhao, L. Wang, H. Zhang, X. Wu, B. Zhao and F. Han, Effect of potassium permanganate dosing position on the performance of coagulation/ultrafiltration combined process, Chin. J. Chem. Eng., 2018, 26, 89–95 CrossRef.
- M. Yagi, M. Kajino, U. Matsuo, K. Ashitani, T. Kita and T. Nakamura, Odor problems in lake Biwa, Water Sci. Technol., 1983, 15, 311 CrossRef CAS.
- H. G. Peterson, S. E. Hrudey, I. A. Cantin, T. R. Perley and S. L. Kenefick, Physiological toxicity, cell membrane damage and the release of dissolved organic carbon and geosmin by Aphanizomenon flos-aquae after exposure to water treatment chemicals, Water Res., 1995, 29, 1515–1523 CrossRef CAS.
-
A. M. Lazur and R. P. E. Yanong, The Use of Potassium Permanganate in Fish Ponds, University of Florida, Gainesville, FL, 2013 Search PubMed.
- J.-J. Chen and H.-H. Yeh, The mechanisms of potassium permanganate on algae removal, Water Res., 2005, 39, 4420–4428 CrossRef CAS PubMed.
- S. Dash, S. Patel and B. K. Mishra, Oxidation by permanganate: synthetic and mechanistic aspects, Tetrahedron, 2009, 65, 707–739 CrossRef CAS.
- H.-Q. Wang, T.-G. Mao, B.-D. Xi, L.-Y. Zhang and Q.-H. Zhou, KMnO4 pre-oxidation for Microcystis aeruginosa removal by a low dosage of flocculant, Ecol. Eng., 2015, 81, 298–300 CrossRef.
- B. Liu, F. Qu, W. Chen, H. Liang, T. Wang, X. Cheng, H. Yu, G. Li and B. Van der Bruggen, Microcystis aeruginosa-laden water treatment using enhanced coagulation by persulfate/Fe(II), ozone and permanganate: Comparison of the simultaneous and successive oxidant dosing strategy, Water Res., 2017, 125, 72–80 CrossRef CAS PubMed.
- J. Clayton and F. Matheson, Optimising diquat use for submerged aquatic weed management, Hydrobiologia, 2010, 656, 159–165 CrossRef.
- E. J. Phlips, P. Hansen and T. Velardi, Effect of the herbicide diquat on the growth of microalgae and cyanobacteria, Bull. Environ. Contam. Toxicol., 1992, 49, 750–756 CrossRef CAS.
-
Wisconsin Department of Natural Resources, Diquat Chemical Fact Sheet, Wisconsin Department of Natural Resources, Madison, WI, 2012 Search PubMed.
- H. G. Peterson, C. Boutin, P. A. Martin, K. E. Freemark, N. J. Ruecker and M. J. Moody, Aquatic phyto-toxicity of 23 pesticides applied at expected environmental concentrations, Aquat. Toxicol., 1994, 28, 275–292 CrossRef CAS.
- S. M. Bartell, K. R. Campbell, C. M. Lovelock, S. K. Nair and J. L. Shaw, Characterizing aquatic ecological risks from pesticides using a diquat dibromide case study III. Ecological process models, Environ. Toxicol. Chem., 2000, 19, 1441–1453 CrossRef CAS.
- K. K. Schrader and C. S. Tucker, Evaluation of diquat as a potential algicide for controlling the musty-odor-producing cyanobacterium, Oscillatoria perornata, in catfish aquaculture ponds, J. Appl. Aquaculture, 2003, 14, 149–154 CrossRef.
- A. Melendez, R. Kepner Jr, J. Balczon and J. Pratt, Effects of diquat on freshwater microbial communities, Arch. Environ. Contam. Toxicol., 1993, 25, 95–101 CrossRef CAS.
- M. E. DeLorenzo, G. I. Scott and P. E. Ross, Toxicity of pesticides to aquatic microorganisms: a review, Environ. Toxicol. Chem., 2001, 20, 84–98 CrossRef CAS PubMed.
-
G. Jager, Herbicides, Chemistry of Pesticides, Wiley, New York, 1983, pp. 322–392 Search PubMed.
- G. Zweig, N. Shavit and M. Avron, Diquat (1, 1′-ethylene-2, 2′-dipyridylium dibromide) in photoreactions of isolated chloroplasts, Biochim. Biophys. Acta, Biophys. Incl. Photosynth., 1965, 109, 332–346 CrossRef CAS.
-
USAID, Litani water quality management project: rapid review report, Development Alternatives, Inc. (DAI), 2005 Search PubMed.
-
ELARD, Business Plan For Combating Pollution of The Qaraoun Lake, Report LB-EQM-UND-CPQ-10, 2011 Search PubMed.
- A. Atoui, H. Hafez and K. Slim, Occurrence of toxic cyanobacterial blooms for the first time in Lake Karaoun, Lebanon, Water Environ. J., 2013, 27, 42–49 CrossRef CAS.
- M. Bouvy, N. Ba, S. Ka, S. Sane, M. Pagano and R. Arfi, Phytoplankton community structure and species assemblage succession in a shallow tropical lake (Lake Guiers, Senegal), Aquat. Microb. Ecol., 2006, 45, 147–161 CrossRef.
- H. W. Paerl and C. S. Tucker, Ecology of blue-green algae in aquaculture ponds, J. World Aquacult. Soc., 1995, 26, 109–131 CrossRef.
- H. Tsukada, S. Tsujimura and H. Nakahara, Seasonal succession of phytoplankton in Lake Yogo over 2 years: effect of artificial manipulation, Limnology, 2006, 7, 3–14 CrossRef CAS.
-
USEPA, Short-term Methods for Estimating the Chronic Toxicity of Effluents and Receiving waters to Freshwater Organisms, United States Environmental Protection Agency, U.S. Environmental Protection Agency Office of Water (4303T), 1200 Pennsylvania
Avenue, NW Washington, DC 20460, 2002 Search PubMed.
-
R. R. Guillard, in Culture of marine invertebrate animals, Springer, 1975, pp. 29–60 Search PubMed.
-
J. Hodgeson, W. Bashe and J. W. Eichelberger, Method 549.2: Determination of Diquat and Paraquat in Drinking Water by Liquid-solid Extraction and High Performance Liquid Chromatography with Ultraviolet Detection, United States Environmental Protection Agency, Office of Research and Development, National Exposure Research Laboratory, 1997 Search PubMed.
-
J. Creed, T. Martin and J. O'Dell, Method 200.9 determination of trace elements by stabilized temperature graphite furnace atomic absorption, revision 2.2, 1994 Search PubMed.
-
P. A. Lewis, D. J. Klemm, J. M. Lazorchak, T. J. Norberg-King, W. H. Peltier and M. A. Heber, Short-term methods for estimating the chronic toxicity of effluents and receiving waters to freshwater organisms, US Environmental Protection Agency, Environmental Monitoring Systems Laboratory, 1994 Search PubMed.
-
E. W. Rice, L. Bridgewater, F. Water Environment, A. American Water Works and A. American Public Health, Standard methods for the examination of water and wastewater, American Public Health Association, Washington, D.C, 2012 Search PubMed.
-
A. Eaton and M. Franson, Standard Methods for the Examination of Water and Wastewater, American Water Works Association & Water Environment Federation, Washington, DC, 2005 Search PubMed.
- A. J. Calomeni and J. H. Rodgers, Evaluation of the utility of six measures for algal (Microcystis aeruginosa, Planktothrix agardhii and Pseudokirchneriella subcapitata) viability, Ecotoxicol. Environ. Saf., 2015, 111, 192–198 CrossRef CAS PubMed.
-
R Core Team, R: A Language and Environment for Statistical Computing, R Foundation for Statistical Computing, Vienna, Austria, 2015 Search PubMed.
- M. Di Fonzo, B. Collen and G. M. Mace, A new method for identifying rapid decline dynamics in wild vertebrate populations, Ecol. Evol., 2013, 3, 2378–2391 CrossRef PubMed.
-
A. Gelman, Y.-S. Su, M. Yajima, J. Hill, M. G. Pittau and J. Kerman, arm: Data analysis using regression and multilevel/hierarchical models. 2015, URL https://CRAN. R-project. org/package= arm, R package version, 2016, vol. 1, pp. 9–3 Search PubMed.
-
S. S. Qian, Environmental and ecological statistics with R, CRC Press, 2016 Search PubMed.
- J. Fan, R. Daly, P. Hobson, L. Ho and J. Brookes, Impact of potassium permanganate on cyanobacterial cell integrity and toxin release and degradation, Chemosphere, 2013, 92, 529–534 CrossRef CAS.
- D. I. Greenfield, A. Duquette, A. Goodson, C. J. Keppler, S. H. Williams, L. M. Brock, K. D. Stackley, D. White and S. B. Wilde, The effects of three chemical algaecides on cell numbers and toxin content of the cyanobacteria Microcystis aeruginosa and Anabaenopsis sp, Environ. Manage., 2014, 54, 1110–1120 CrossRef.
- E. C. de Oliveira-Filho, R. M. Lopes and F. J. R. Paumgartten, Comparative study on the susceptibility of freshwater species to copper-based pesticides, Chemosphere, 2004, 56, 369–374 CrossRef CAS.
- M. J. Hanson and H. G. Stefan, Side effects of 58 years of copper sulfate treatment of the fairmont lakes, MINNESOTA 1, J. Am. Water Resour. Assoc., 1984, 20, 889–900 CrossRef CAS.
- J. H. J. Rodgers, B. M. Johnson and W. M. Bishop, Comparison of Three Algaecides for Controlling the Density of Prymnesium parvum, J. Am. Water Resour. Assoc., 2010, 46, 153–160 CrossRef CAS.
- C. Murray-Gulde, J. Heatley, A. Schwartzman and J. Rodgers Jr, Algicidal effectiveness of clearigate, cutrine-plus, and copper sulfate and margins of safety associated with their use, Arch. Environ. Contam. Toxicol., 2002, 43, 19–27 CrossRef CAS PubMed.
-
D. A. Salam, Interfaculty Graduate Environmental Sciences, Dissertation/Thesis, E. American University of Beirut. Faculty of and P. Architecture, 2006 Search PubMed.
- E. Costas, E. Carrillo, L. M. Ferrero, M. Agrelo, L. Garcia-Villada, J. Juste and V. López-Rodas, Mutation of algae from sensitivity to resistance against environmental selective agents: the ecological genetics of Dictyosphaerium chlorelloides (Chlorophyceae) under lethal doses of 3-(3, 4-dichlorophenyl)-1, 1-dimethylurea herbicide, Phycologia, 2001, 40, 391–398 CrossRef.
- V. Lopez-Rodas, M. A. R. Agrelo, E. Carrillo, L. M. Ferrero, A. Larrauri, L. MartÍN-Otero and E. Costas, Resistance of microalgae to modern water contaminants as the result of rare spontaneous mutations, Eur. J. Phycol., 2001, 36, 179–190 CrossRef.
- S. Zhou, Y. Shao, N. Gao, Y. Deng, J. Qiao, H. Ou and J. Deng, Effects of different algaecides on the photosynthetic capacity, cell integrity and microcystin-LR release of Microcystis aeruginosa, Sci. Total Environ., 2013, 463, 111–119 CrossRef PubMed.
- G. J. Jones and P. T. Orr, Release and degradation of microcystin following algicide treatment of a Microcystis aeruginosa bloom in a recreational lake, as determined by HPLC and protein phosphatase inhibition assay, Water Res., 1994, 28, 871–876 CrossRef CAS.
- Y. E. Roman, K. A. C. De Schamphelaere, L. T. H. Nguyen and C. R. Janssen, Chronic toxicity of copper to five benthic invertebrates in laboratory-formulated sediment: Sensitivity comparison and preliminary risk assessment, Sci. Total Environ., 2007, 387, 128–140 CrossRef CAS PubMed.
- W. Sanchez, O. Palluel, L. Meunier, M. Coquery, J.-M. Porcher and S. Aït-Aïssa, Copper-induced oxidative stress in three-spined stickleback: relationship with hepatic metal levels, Environ. Toxicol. Pharmacol., 2005, 19, 177–183 CrossRef CAS.
- M.-A. Coutellec, G. Delous, J.-P. Cravedi and L. Lagadic, Effects of the mixture of diquat and a nonylphenol polyethoxylate adjuvant on fecundity and progeny early performances of the pond snail Lymnaea stagnalis in laboratory bioassays and microcosms, Chemosphere, 2008, 73, 326–336 CrossRef CAS.
Footnotes |
† Electronic supplementary information (ESI) available. See DOI: 10.1039/c8ew00532j |
‡ Diquat dibromide will be referred to as diquat in the remaining of the manuscript |
|
This journal is © The Royal Society of Chemistry 2019 |