Mitigation of solute buildup by using a biodegradable and reusable polyelectrolyte as a draw solute in an osmotic membrane bioreactor
Received
13th August 2018
, Accepted 4th November 2018
First published on 5th November 2018
Abstract
Reverse solute flux (RSF) is a key challenge for operating osmotic membrane bioreactors (OMBRs) and can severely affect the OMBR performance due to solute accumulation in the feed side. Herein, a biodegradable polyelectrolyte (polyacrylic acid sodium salt, PAA) was investigated as a draw solute (DS) for OMBR application with a focus on the mitigation of solute build-up via biodegradation of PAA. Both activated sludge and OMBR tests confirmed that PAA can be biologically degraded. In the OMBR, a residue PAA concentration of 14.1 mg L−1 was observed in the feed solution due to a dynamic balance between PAA removal/biodegradation and continuous RSF. Compared to a non-biodegradable DS (NaCl) that had a water recovery of 3.4 ± 0.1 L and a reverse solute flux (RSF) of 9.46 ± 0.21 gMH, the PAA DS achieved a higher water recovery of 17.9 ± 0.5 L over 30 days of operation (the average water flux, 4.97 ± 0.14 LMH), a much lower RSF of 0.12 ± 0.01 gMH, and a higher nitrogen removal of 58.4 ± 1.5%. The recovery and reuse of the PAA DS were investigated with a long-term operation (150 days), which demonstrated the feasibility of reusing the PAA DS, despite some decrease in water recovery and biological treatment performance. Those results have collectively demonstrated the advantages of the PAA-based draw solute for reducing reverse-fluxed solutes towards optimized OMBR operation and applications.
Water impact
Osmotic membrane bioreactors (OMBRs) are of great interest because of their advantages in energy-efficient and low-fouling water recovery from wastewater. However, accumulation of draw solutes due to reverse solute flux can severely affect the performance of an OMBR. Herein, the problem of draw solute accumulation is addressed by using a biodegradable draw solute, resulting in enhanced water recovery and contaminant removal.
|
1. Introduction
Osmotic membrane bioreactors (OMBRs), combining biological processes with forward osmosis (FO) membrane separation, can be used to recover reusable water from wastewater.1–3 In an OMBR, water spontaneously migrates across a selective FO membrane from the mixed liquor into a concentrated draw solution under an osmotic driving force.4 The use of the FO membrane in OMBRs offers several advantages over traditional MBRs, such as low fouling propensity due to the dense and tight surface structure, low energy consumption by using a natural osmotic pressure for separation, and a high rejection rate for a wide range of contaminants.5–7 In recent years, OMBRs have been investigated for various potential applications such as removal of emerging trace organic compounds, removal of heavy metals, and recovery of nutrient or bioenergy.8–11 Pilot scale OMBRs were developed and studied for long-term operation to produce reclaimed water from municipal wastewater.12,13
Despite the great potential of OMBRs, a key challenge for a proper OMBR operation is the reverse salt transport from the draw solution, or RSF (reverse solute flux), which can result in the accumulation of draw solutes in the feed wastewater. This accumulation can cause salinity buildup in the bioreactor, which not only leads to a reduction of permeate flux due to the increased internal concentration polarization and the decreased effective osmotic driving force, but also adversely impacts the microbial activity and functional species in the bioreactor.14,15 It was reported that when the conductivity of the feed wastewater increases to 19.3 mS cm−1 due to RSF, the organic degradation in an OMBR could decrease from 96.2% to 13.9%.16 The most common method to mitigate this solute buildup is the periodic discharge of mixed liquid by adjusting the operating solid retention time (SRT) to achieve a stable salinity in the feed solution.17 However, operating an OMBR at a short SRT will limit the growth of slow-growing microorganisms (e.g., nitrite-oxidizing bacteria). An alternative strategy is to integrate a microfiltration (MF) or ultrafiltration (UF) process with OMBRs to reject suspended solids (i.e., to increase the SRT) and extract dissolved inorganic salts in the liquid from the bioreactor.5,18 For example, the buildup of salinity is intermittently controlled through an MF membrane in an osmotic membrane bioreactor–reverse osmosis system.19 However, further disposal of the extracted supernatant containing a high concentration of solutes will be challenging, and those pressure-driven membrane separation processes also require high energy input. The reverse-fluxed solutes can also be removed and recovered in a hybrid OMBR-electrodialysis (ED) system,16 but further efforts are needed to reduce energy consumption through optimizing system operation and/or using renewable energy (e.g., solar and wind).
A promising approach to address solute buildup is to use a draw solute (DS) that can be degraded by microorganisms.20,21 Previous results have shown that the acetate-based DS can be degraded by microorganisms in the OMBR and thus solute build-up was mitigated.21 Inorganic DSs such as ammonia-based solutes were also studied for mitigation of solute buildup by anammox.22 Compared to inorganic salts (i.e., NaCl), organic-based solutes such as acetate compounds could improve the characteristics of the mixed liquor in terms of sludge filterability, particle size, and biomass growth along with the degradation of soluble microbial products (SMPs).23 A variety of organic compounds with small carbon chains, such as sodium formate, sodium acetate, sodium propionate, and magnesium acetate, have been investigated in the OMBR, and the reverse-fluxed solutes could act as a carbon source for biodegradation and may also aid in nutrient removal.24 Although the solute concentration in the mixed liquor is not significantly elevated due to biodegradation, the problem of DS loss due to continuous RSF still exists and can increase the operational cost by DS replenishment. Therefore, a biodegradable DS with minimal RSF will be preferred for OMBRs.
Polyelectrolyte DSs such as polyacrylamide and polyethylenimine have been studied and evaluated in FO systems, and generally require energy-demanding membrane separation to produce the final water product.25,26 A candidate DS is the commercial and nontoxic polymer poly(acrylic acid) (PAA) which is pH-responsive and has carboxylic segments to change its affinity with water by using an acid/base. PAA can produce a high osmotic pressure in the form of alkali metal or ammonium salts.27 Previous studies have investigated PAA as a DS in an FO system to produce high water flux with very low RSF, and employed a combination of pH adjustment and microfiltration (MF) to achieve efficient recovery.28,29 It has been reported that a small amount of PAA–Na could be used by microorganisms as a substrate in activated sludge.30 Thus, the properties of high water flux, low RSF, and biodegradability make PAA a potentially suitable DS for OMBRs. The specific objectives of this study were to (1) confirm the biodegradation of PAA in an activated sludge system and OMBR; (2) examine the mitigation of reverse-fluxed solutes via biodegradation of PAA; (3) evaluate the OMBR performance with the PAA DS, compared to that of a non-biodegradable DS (NaCl); and (4) investigate the recovery and reuse of the PAA DS in a long-term operation of the OMBR (∼150 days).
2. Materials and methods
2.1 OMBR set up
A submerged OMBR system was set up (Fig. 1) and consisted of two pieces of a cellulose triacetate (CTA) FO membrane (Aquaporin A/S, Denmark) with a total surface area (S) of 0.005 m2, creating a middle draw chamber of 15 mL. The FO membrane had a guaranteed pH tolerance of 2–12 as suggested by its manufacturer.31 The active layer of the membrane was oriented toward the feed solution, while the support side facing the draw solution to minimize fouling. Plastic mesh was installed to hold the membrane and prevent potential membrane swelling. The FO cell was submerged in a 1 L plastic beaker containing the feed solution (synthetic wastewater), which was supplemented daily to maintain a constant liquid volume of 500 mL (a calculated HRT of 12.5 h). The synthetic wastewater was intended to mimic municipal wastewater containing (per L of deionized water): sodium acetate, 125 ± 8 mg (100 ± 6 mg COD); NH4Cl, 100 ± 4 mg (26 ± 2 mg N); MgSO4, 15 ± 1 mg; CaCl2, 20 ± 1 mg; NaHCO3, 100 ± 8 mg; KH2PO4, 3.2 ± 0.2 mg; K2HPO4, 6.8 ± 0.3 mg (2.0 ± 0.1 mg P); and trace elements, 1 mL.32 The feed compartment was inoculated with 50 mL of sludge from an anaerobic digester (6.0 g L−1 mixed liquid suspended solids) of a local wastewater treatment plant (Pepper's Ferry Wastewater Treatment Authority, Radford, VA, USA).
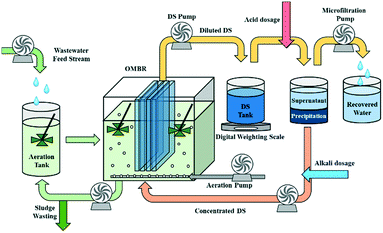 |
| Fig. 1 Schematic diagram of an osmotic membrane bioreactor (OMBR) using biodegradable PAA as a draw solute. | |
The feed solution was aerated with air at a flow rate of 100 mL min−1 to provide both aeration and mixing (dissolved oxygen, 2.0 mg L−1), and additional mixing was provided by magnetic stirring (mixed liquor suspended solids, 1.8 g L−1; mixed liquor volatile suspended solids, 1.3 g L−1). The PAA draw solution was prepared by dissolving 2000 PAA–Na DS (sodium salt, powder, Polysciences, Inc., Warrington, PA, USA) in DI water to achieve 100 g of alkaline solution at three different concentrations. When being applied to the OMBR, the draw solution was recirculated at a flow rate of 50 mL min−1. A 2000 mL flask placed on a digital balance was used to monitor the water transported through the FO membrane into the draw chamber. To control fouling, the FO membrane was cleaned by physical flushing with DI water at the end of each batch test, and the membrane was reused for the next batch test. Both draw and feed samples were collected every 24 h for water quality analysis.
2.2 Experimental procedure
The experiment was conducted at room temperature (21 ± 1 °C) with a centralized air conditioning system, and involved three parts: biodegradability of PAA and alleviation of solute buildup, comparison to a non-biodegradable DS, and long-term operation of the OMBR with recovery and reuse of the PAA DS. To verify the biodegradation of PAA by aerobic organisms (mixed liquor suspended solids, 2.1 g L−1; mixed liquor volatile suspended solids, 1.5 g L−1; dissolved oxygen, 2.0 mg L−1), a 2 L continuous-flow activated sludge system using 2000 PAA–Na as the sole substrate was operated for 30 days: during days 4–10, PAA was injected daily at 50 mg d−1, and on day 11 the PAA dosage was stopped; the PAA concentration was monitored throughout 30 days. The biodegradation of PAA was further examined in the OMBR that was compared to an FO system (no microorganisms). To make the comparison with a non-biodegradable DS in the OMBR, NaCl with a concentration of 10 wt% and PAA with three concentrations (i.e., 10 wt%, 20 wt%, and 30 wt%) at two SRTs (i.e., 10 d and 20 d) were systematically studied to examine water recovery and treatment performance from the synthetic wastewater. Under the optimized operating conditions, the OMBR was operated with the reused PAA DS for five cycles (a total of 150 d) to examine the key performance parameters such as water flux, pollutant removal, and energy consumption. The first cycle used the fresh PAA DS and the following four cycles used the recovered PAA DS. During this stage, a dose of acid and alkali was used to achieve PAA DS regeneration for reuse in an energy efficient way after each batch test (30 d of operation), following the procedure reported by a previous study.28
2.3 Measurement and analysis
The concentrations of NH4+-N, total nitrogen (TN), and PO43−-P were measured by using a DR/890 colorimeter (HACH Co., Ltd., USA) according to the manufacturer's instruction. The organic carbon content was determined using a TOC-VCSN (Shimadzu, Japan). The TOC degradation rate (mg L−1 d−1) was calculated using the TOC concentration change over the time interval. The concentration of PAA was analyzed using a size exclusion chromatographer (Waters Co., USA) and a FTIR spectrometer (Mettler-Toledo LLC, USA). The PAA degradation rate (RPAA, mg L−1 d−1) was determined by the PAA concentration change between the theoretical value and measured value over the time interval Δt, and the theoretical PAA concentration (CPAA,F, mg L−1) in the feed side was calculated as: | 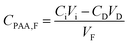 | (1) |
| 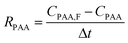 | (2) |
where Ci and CF are the initial and final PAA concentrations, respectively, over a predetermined time Δt. Vi and VD are the initial and final draw volumes, respectively. VF is the final feed volume (L). CPAA is the measured PAA concentration in the feed side (mg L−1). The osmotic pressure was estimated as:where π is the osmotic pressure (atm), i is the dimensionless van 't Hoff factor, M is the molarity, R is the gas constant (0.082057 L atm K−1 mol−1), and T is the absolute temperature (K).33 The water flux, JW (L m−2 h−1, or LMH), was determined by measuring the weight change of the draw solution versus time using an electronic balance (Scort Pro, Ohous, USA) at a 30 s interval that was controlled by the software LoggerPro: |  | (4) |
where Δm (g) is the volume change of the draw solution over the time interval Δt, and A (m2) is the effective membrane surface area. Water recovery was the weight change of the draw solution after a period of operation time (e.g., for the batch test with an operation time of 30 d). The reverse solute flux, JS (g m−2 h−1, or gMH), was determined by converting the total organic carbon of the feed solution per unit membrane area and unit time: | 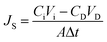 | (5) |
According to the mass balance of the solute in the feed solution, the sum of the initial mass of the solute in the feed side and the reverse-fluxed solute from the draw side is equal to the solute mass in the supernatant and that passing through the FO membrane into the draw side, as described by the following equation:
| Minf + Mrsf = Meff + Mout | (6) |
where
Minf is the initial mass of the solute in the feed solution,
Mrsf is the mass of the solute migrating to the feed solution,
Mout is the mass of the solute moving to the draw solution, and
Meff is the final mass of solute in the feed solution. Due to the low RSF of the PAA DS,
Mout is assumed to be equal to zero. Thus, the terms in
eqn (6) can be rearranged using the concentration, volume, water flux, and reverse salt flux as follows:
| 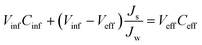 | (7) |
If the SRT is much greater than the HRT, eqn (7) can be simplified to:22
| 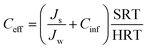 | (8) |
The electric power used by the membrane recovery process (Pmembrane, kW) was estimated as:
where
Qp is the permeate flow rate for membrane separation (m
3 s
−1), and
P is the pressure at the module inlet (Pa). The power requirement for the recirculation pump system (
Ppumping, kW) was estimated as:
|  | (10) |
where
Q is the flow rate for pump recirculation (m
3 s
−1),
γ is the specific weight of water (9800 N m
−3), and
h is the hydraulic pressure head (0.02 m). The power consumption by diffused aeration (
Paeration, kW) was estimated as:
|  | (11) |
where
P1 is the standard atmospheric pressure (101
![[thin space (1/6-em)]](https://www.rsc.org/images/entities/char_2009.gif)
325 Pa),
P2 is the blower inlet pressure (Pa),
T is the air temperature (294 K),
ζ is the blower efficiency (0.8),
λ is the aerator constant (1.4), and
ρ0 is the air density under standard conditions (1.29 kg m
−3). The total energy consumption (
E, kW h m
−3) was estimated as:
| 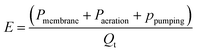 | (12) |
where
Qt is the flow rate for the treated wastewater (m
3 h
−1).
34
3. Results and discussion
3.1 Biodegradability of PAA
Biodegradation of PAA by aerobic organisms was verified in an activated sludge system, and the results show that PAA did not accumulate in the reactor despite continuous addition (Fig. 2). In the first ten days with a frequent dosage of 50 mg d−1 PAA (starting on day 4 till day 10), its concentration decreased from 141 to 121 mg L−1 with a degradation rate of 51–57 mg L−1 d−1. This confirmed the biodegradable nature of PAA. Further proof of PAA biodegradation was obtained by stopping the PAA addition: its concentration started to decrease from 120 mg L−1 on day 11 to 14 mg L−1 on day 30 (Fig. 2), proving that PAA is biodegradable. The residue PAA was due to incomplete degradation, and this is consistent with the previous finding where radiochemical (14C) data confirmed the relatively low degradation potential of 2000 PAA.30 The biodegradation rate of PAA was dependent on its concentration during the period of frequent addition and after stopping the PAA addition, its biodegradation rate would be strongly related to the retention time. Some PAA residue should be expected due to incomplete degradation.
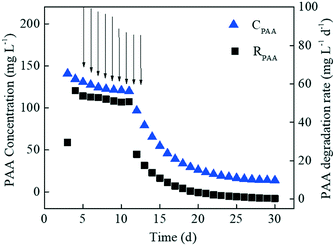 |
| Fig. 2 The PAA biodegradation in an activated sludge system. Note: the arrows indicate 50 mg d−1 dosage; CPAA is the PAA concentration; and RPAA is the PAA degradation rate. | |
3.2 Alleviation of solute buildup
The alleviation of solute buildup was examined by comparing the PAA accumulation (or concentration) between the OMBR and an FO system that was set up in the same type of reactor as the OMBR but had no sludge inocula. The accumulation of organic matter in the feed solution was observed in both the OMBR and the FO system. However, the PAA accumulation behaved differently between the two systems: its concentration kept increasing in the FO system and reached 35.2 mg L−1 at the end of the 30 day operation, while in the OMBR the PAA concentration increased initially until day 10 and then slightly decreased and became relatively stable in the remaining 20 days with a final concentration of 14.1 mg L−1 (Fig. 3A). This difference was attributed to the biodegradation of PAA in the OMBR, which helped maintain a relatively low level of PAA concentration. The reason why the PAA concentration reached a plateau instead of further decreasing in the OMBR was likely due to a dynamic balance between PAA degradation and continuous RSF of the PAA DS. The lower level of PAA accumulation in the OMBR has benefited water flux and resulted in a higher water recovery of 18.0 ± 0.7 L than 16.2 ± 0.5 L in the FO system over 30 days of operation (insert of Fig. 3A, p < 0.05, a one-tailed two-sample t-test with unequal variance at α = 0.05 for all the following statistical tests).
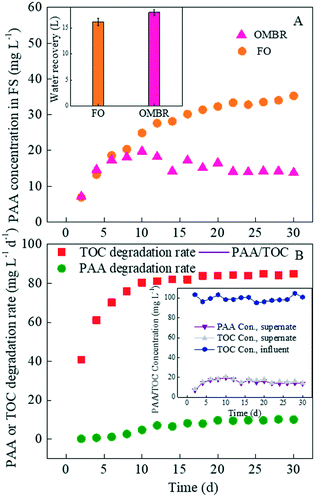 |
| Fig. 3 Mitigation of reverse-fluxed PAA at an SRT of 10 d and with 30 wt% PAA DS: (A) the comparison of PAA concentration in the feed solution and water recovery between the OMBR (biodegradation) and the FO system (no biodegradation); and (B) TOC degradation and PAA degradation in the OMBR. | |
Fig. 3B shows that the PAA degradation rate increased to 9.5 mg L−1 d−1 in the first 20 days, and then tended to stabilize at 10.1 ± 1.2 mg L−1 d−1; meanwhile, the PAA biodegradation efficiency reached 57.5–58.1% after 30 days of operation. These results have confirmed the biodegradation of PAA and that the reverse-fluxed PAA could be removed via biodegradation in the OMBR. Significant degradation of TOC was observed in the OMBR, and the TOC concentration decreased from 100.5 ± 3.0 mg L−1 to 15.3 ± 2.8 mg L−1 with a maximum degradation rate of 81.1 ± 2.1 mg L−1 d−1. The residue TOC was mostly PAA, because PAA accounted for 84.7–98.6% of TOC. This suggested that the readily biodegradable carbon source (i.e., acetate) could be easily used by the microorganisms, and more complex substrates such as PAA would need more time to be degraded.
3.3 Comparison with a non-biodegradable draw solute
The benefits of using biodegradable PAA as a DS in the OMBR were further demonstrated by comparing it to a non-biodegradable DS, NaCl. To do this, three PAA DS concentrations, 10, 20, and 30 wt%, were investigated at two different SRTs, and the performance of the OMBR was compared to that with 10 wt% NaCl DS (Fig. 4 and 5). At an SRT of 10 d, the water recovery of the OMBR with 10 wt% PAA DS was 4.3 ± 0.3 L, which increased to 17.9 ± 0.5 L when the PAA DS concentration was increased to 30 wt% (Fig. 4A, p < 0.05). This increase was due to a higher theoretical osmotic pressure with a higher DS concentration; however, this increase became slower with the increase in DS concentration, likely due to the increased internal concentration polarization (ICP), which may arise from the higher viscosity of polyelectrolytes at a higher concentration.27 For comparison, the water recovery with 10 wt% NaCl (1.9 M) was 3.4 ± 0.1 L, lower than that with 10 wt% PAA (p < 0.05). According to previous studies, the osmotic pressure of 10 wt% PAA is 20 atm, which could increase to 38 atm (20 wt%) or 54 atm (30 wt%), while that of 10 wt% NaCl was about 80 atm.27,35 Thus, one can see that although the osmotic pressure of NaCl is much higher than that of PAA (even with a higher weight concentration), the high salt leakage of the NaCl DS might have resulted in lower water recovery over the 30-day operation.
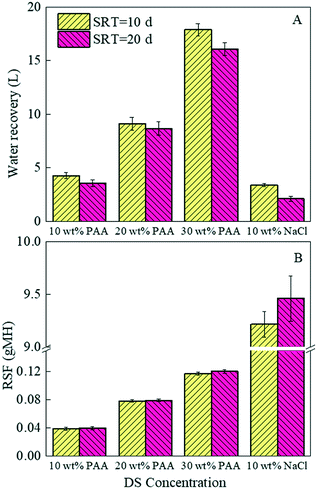 |
| Fig. 4 Comparison between the PAA DS and a non-biodegradable DS (NaCl) in the OMBR at two SRTs (10 d and 20 d): (A) water recovery over 30 days of operation; and (B) reverse salt flux (RSF). Error bars represent the standard deviation, n = 3. | |
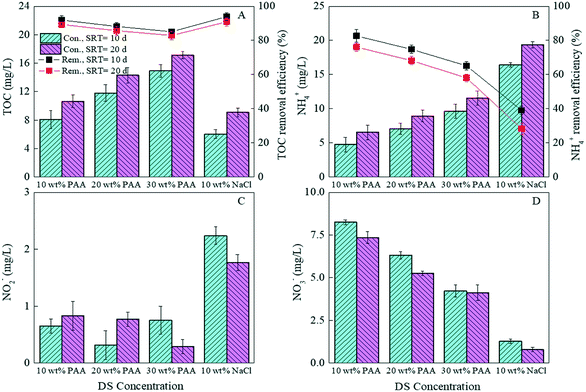 |
| Fig. 5 The treatment performance of the OMBR with two types of DSs at two SRTs (10 d and 20 d): (A) TOC concentration and removal; (B) NH4+ concentration and removal; (C) NO2− concentration; and (D) NO3− concentration. Error bars represent the standard deviation, n = 3. | |
The SRT also had a strong effect on water recovery, and the water recovery at an SRT of 20 d was lower than that at an SRT of 10 d for all the tested DSs (p < 0.05). This was mainly attributed to the reduced driving force resulting from the solute accumulation at a higher SRT. The trend of RSF was similar to that of water flux, and increased with a higher DS concentration. The highest RSF of 0.12 ± 0.01 gMH was obtained with 30% PAA (Fig. 4B). However, the RSF with 10 wt% NaCl was 9.46 ± 0.21 gMH, which was 231–237 times higher than that of 10 wt% PAA, or even 76–80 times higher than that of 30 wt% PAA. A longer SRT of 20 d slightly increased the RSF of NaCl to 9.46 ± 0.21 gMH resulting from a higher solute concentration in accord with eqn (8). The PAA DS had a similar RSF at two SRTs (p > 0.05), possibly because of the biodegradable effect of PAA resulting in less solute accumulation compared with the NaCl DS.
The organic matter and nutrients in an OMBR are removed by both biodegradation and membrane separation. Biodegradation in the feed is often the rate limiting step for the removal of organic matter and nutrients, while membrane separation can achieve high pollutant removal.36 The removal of organics in the feed solution (mixed liquor) was investigated by monitoring the TOC concentration. The OMBR with 10 wt% PAA DS was able to reduce the organic concentration from 103.6 to 8.1 mg L−1, and the residue TOC was mostly the residue PAA. A higher DS concentration at 30 wt% resulted in higher RSF of PAA and thus a higher residue TOC concentration of 14.9 ± 0.9 mg L−1 at an SRT of 10 d (Fig. 5A). For comparison, the residue TOC concentration with the NaCl DS was lower at 6.1 ± 0.6 mg L−1, because the NaCl DS did not contain any organic matter. A higher TOC concentration in the OMBR at an SRT of 20 d (p < 0.05) might be because of a higher salinity that could cause the loss of plasmolysis and metabolic activity, which in turn resulted in the release of intracellular constituents and soluble microbial products.37
The nitrogen compounds in wastewater are commonly removed by nitrification under aerobic conditions, followed by a biomass conversion process. The growth of nitrifiers in an OMBR can be inhibited by the elevation of salinity, resulting in ammonia accumulation in the supernatant of the mixed liquor.8 In the present study, the ammonium concentration in the OMBR was 4.8 ± 1.1, 7.1 ± 0.9, and 9.6 ± 1.0 mg L−1, corresponding to a removal efficiency of 82.6 ± 3.7, 74.8 ± 2.3, and 65.1 ± 3.4% at three PAA DS concentrations and an SRT of 10 d. For comparison, the OMBR with the NaCl DS had a higher ammonium concentration of 16.5 ± 0.3 mg L−1 (SRT 10 d) with a removal efficiency of 39.0 ± 2.3% (Fig. 5B). The higher ammonium removal with the PAA DS than that with the NaCl DS was likely because of the fact that the PAA solute build-up was mitigated by biodegradation, thereby benefiting the nitrifying organisms. There was little nitrite (0.3–0.8 mg L−1) in the OMBR with the PAA DS, but the system with the NaCl DS had a higher nitrite concentration of 1.8–2.2 mg L−1 in the feed (Fig. 5C, p < 0.05), indicating the inhibition of NOB. This is because of the fact that NOB is more sensitive than AOB under high salinity conditions,38 and as a result, the OMBR with the NaCl DS had incomplete nitrification. Nitrate accumulation was observed and its concentration with the PAA DS decreased from 8.2 ± 0.1 to 4.2 ± 0.4 mg L−1 with increasing DS concentration from 10 to 30 wt% (Fig. 5D). The NaCl DS had a low nitrate concentration, due to inefficient nitrification, resulting in significant ammonium accumulation (82.1–87.7% of TN).
3.4 Draw solute recovery and reuse with long-term operation
The recovery and reuse of the PAA DS were investigated with a long-term operation (150 days) using 30 wt% PAA DS and for five cycles (30 days per cycle), and major results are summarized in Fig. 6. In the first cycle, the fresh PAA DS was used and in the subsequent four cycles, the recovered PAA DS was employed. During the 30 day operation, the water flux generated by the fresh PAA DS exhibited a decreasing trend and then stabilized at approximately 4.4 LMH (Fig. 6A). The recovered PAA DS had a 14.8% decrease in water flux at the end of the 4th reuse cycle. The maximum water flux was 11.5 LMH in the 1st cycle (fresh PAA DS), 10.9 LMH in the 2nd cycle (reused PAA DS), 10.8 LMH in the 3rd cycle (reused PAA DS), 10.1 LMH in the 4th cycle (reused PAA DS), and 9.8 LMH in the 5th cycle (reused PAA DS). This was mainly related to a lower conductivity in the recovered PAA DS (i.e., 6.9% decline) due to the loss of PAA during recovery and the progressive accumulation of wastes (i.e., salinity, solute, and cellular debris) in the feed solution during long-term operation, resulting in a decline of the effective osmotic pressure. The TOC concentration firstly increased, likely related to higher reverse solute flux at the beginning of a batch cycle, and then, it decreased and became stable at 17.8 mg L−1 at the end of the first cycle of operation (Fig. 6B), because of the dynamic balance between the PAA degradation and the continuous RSF. The profile of TOC variation was similar among five cycles, but the TOC residue concentration gradually increased to 21.9 mg L−1, possibly due to the accumulation of PAA. The TN concentration had a decreasing trend and stabilized at 15.2 mg L−1 with the fresh PAA DS, and the reused PAA DS had 13.8% higher accumulation at the end of the fourth cycle of reuse (Fig. 6C). The concentration effect due to the water extract could also contribute to the gradual increase in the accumulation of both TOC and nitrogen. Nevertheless, these results have demonstrated the feasibility of recovering and reusing PAA during the long-term operation of the OMBR, and a strategy to deal with the gradual accumulation of TOC and nitrogen which warrants further investigation.
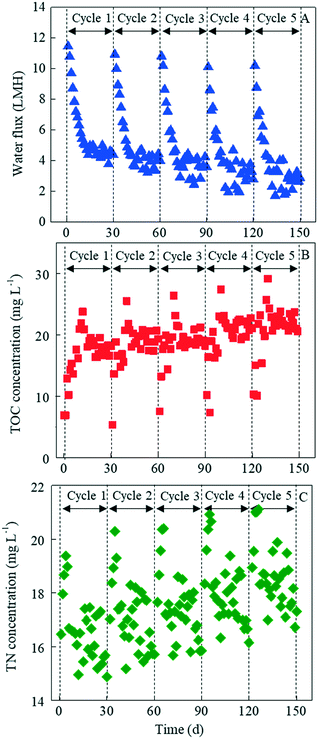 |
| Fig. 6 The long-term operation of the OMBR with recovered PAA at an SRT of 10 d and with 30 wt% PAA DS: (A) average water flux, (B) TOC concentration, and (C) TN concentration. | |
4. Conclusions
In this study, a biodegradable PAA was studied as a reusable draw solute in an OMBR system to mitigate salinity build-up in the feed solution. The reverse organic flux generated by the PAA DS could be controlled by microbial degradation that removed a significant portion of PAA. This mitigation resulted in enhanced water flux and recovery in the OMBR, compared to an FO system. However, due to the continuous RSF, a dynamic balance was observed between PAA removal/biodegradation and PAA residue concentration in the feed solution. The advantages of using the biodegradable PAA DS were more significant when compared to a non-biodegradable DS–NaCl. The OMBR with the PAA DS achieved higher water flux/recovery and better treatment performance in terms of TOC and nitrogen removal. The long-term operation of the OMBR for 150 days has demonstrated the feasibility of recovering and reusing PAA, although some decrease in performance due to PAA loss and accumulation of TOC/nitrogen was observed. These results imply the great promise of using PAA as a draw solute for OMBR systems, and encourage further investigation towards OMBR development.
Conflicts of interest
There are no conflicts to declare.
Acknowledgements
This work was partially supported by the National Natural Science Foundation of China (51828801, 41571476), and an award from the Institute for Critical Technology and Applied Science (ICTAS) of Virginia Tech. Dr. Yuli Yang was supported by the Startup Fund for Talented Scholars of Nanjing Normal University, and the National Science and Technology Major Project of China (2017ZX07202004). The authors would like to thank Mr. Mingtao Chen (Virginia Tech) for helping with the PAA test.
References
- X. Wang, V. W. Chang and C. Y. Tang, Osmotic membrane bioreactor (OMBR) technology for wastewater treatment and reclamation: Advances, challenges, and prospects for the future, J. Membr. Sci., 2016, 504, 113–132 CrossRef CAS.
- R. W. Holloway, A. Achilli and T. Y. Cath, The osmotic membrane bioreactor: a critical review, Environ. Sci.: Water Res. Technol., 2015, 1, 581–605 RSC.
- M. Qin and Z. He, Resource recovery by osmotic bioelectrochemical systems towards sustainable wastewater treatment, Environ. Sci.: Water Res. Technol., 2017, 3, 583–592 RSC.
- A. Achilli, T. Y. Cath, E. A. Marchand and A. E. Childress, The forward osmosis membrane bioreactor: a low fouling alternative to MBR processes, Desalination, 2009, 239, 10–21 CrossRef CAS.
- R. W. Holloway, J. Regnery, L. D. Nghiem and T. Y. Cath, Removal of trace organic chemicals and performance of a novel hybrid ultrafiltration-osmotic membrane bioreactor, Environ. Sci. Technol., 2014, 48, 10859–10868 CrossRef CAS PubMed.
- E. Cornelissen, D. Harmsen, K. De Korte, C. Ruiken, J.-J. Qin, H. Oo and L. Wessels, Membrane fouling and process performance of forward osmosis membranes on activated sludge, J. Membr. Sci., 2008, 319, 158–168 CrossRef CAS.
- W. J. Yap, J. Zhang, W. C. Lay, B. Cao, A. G. Fane and Y. Liu, State of the art of osmotic membrane bioreactors for water reclamation, Bioresour. Technol., 2012, 122, 217–222 CrossRef CAS PubMed.
- G. Qiu and Y.-P. Ting, Direct phosphorus recovery from municipal wastewater via osmotic membrane bioreactor (OMBR) for wastewater treatment, Bioresour. Technol., 2014, 170, 221–229 CrossRef CAS PubMed.
- B. Aftab, S. J. Khan, T. Maqbool and N. P. Hankins, Heavy metals removal by osmotic membrane bioreactor (OMBR) and their effect on sludge properties, Desalination, 2017, 403, 117–127 CrossRef CAS.
- Y. Yang, X. Yang and Z. He, Bioelectrochemically-assisted mitigation of salinity buildup and recovery of reverse-fluxed draw solute in an osmotic membrane bioreactor, Water Res., 2018, 141, 259–267 CrossRef CAS PubMed.
- F. Li, Q. Xia, Y. Gao, Q. Cheng, L. Ding, B. Yang, Q. Tian, C. Ma, W. Sand and Y. Liu, Anaerobic biodegradation and decolorization of a refractory acid dye by a forward osmosis membrane bioreactor, Environ. Sci.: Water Res. Technol., 2018, 4, 272–280 RSC.
- R. W. Holloway, A. S. Wait, A. F. da Silva, J. Herron, M. D. Schutter, K. Lampi and T. Y. Cath, Long-term pilot scale investigation of novel hybrid ultrafiltration-osmotic membrane bioreactors, Desalination, 2015, 363, 64–74 CrossRef CAS.
- G. Blandin, C. Gautier, M. S. Toran, H. Monclús, I. Rodriguez-Roda and J. Comas, Retrofitting membrane bioreactor (MBR) into osmotic membrane bioreactor (OMBR): A pilot scale study, Chem. Eng. J., 2018, 339, 268–277 CrossRef CAS.
- M. S. Nawaz, G. Gadelha, S. J. Khan and N. Hankins, Microbial toxicity effects of reverse transported draw solute in the forward osmosis membrane bioreactor (FO-MBR), J. Membr. Sci., 2013, 429, 323–329 CrossRef CAS.
- W. C. Lay, Q. Zhang, J. Zhang, D. McDougald, C. Tang, R. Wang, Y. Liu and A. G. Fane, Study of integration of forward osmosis and biological process: membrane performance under elevated salt environment, Desalination, 2011, 283, 123–130 CrossRef CAS.
- Y. Lu and Z. He, Mitigation of salinity buildup and recovery of wasted salts in a hybrid osmotic membrane bioreactor–electrodialysis system, Environ. Sci. Technol., 2015, 49, 10529–10535 CrossRef CAS PubMed.
- X. Wang, Y. Chen, B. Yuan, X. Li and Y. Ren, Impacts of sludge retention time on sludge characteristics and membrane fouling in a submerged osmotic membrane bioreactor, Bioresour.
Technol., 2014, 161, 340–347 CrossRef CAS PubMed.
- T. Hu, X. Wang, C. Wang, X. Li and Y. Ren, Impacts of inorganic draw solutes on the performance of thin-film composite forward osmosis membrane in a microfiltration assisted anaerobic osmotic membrane bioreactor, RSC Adv., 2017, 7, 16057–16063 RSC.
- W. Luo, F. I. Hai, W. E. Price, W. Guo, H. H. Ngo, K. Yamamoto and L. D. Nghiem, Phosphorus and water recovery by a novel osmotic membrane bioreactor–reverse osmosis system, Bioresour. Technol., 2016, 200, 297–304 CrossRef CAS PubMed.
- N. C. Nguyen, S.-S. Chen, H. T. Nguyen, H. H. Ngo, W. Guo, C. W. Hao and P.-H. Lin, Applicability of a novel osmotic membrane bioreactor using a specific draw solution in wastewater treatment, Sci. Total Environ., 2015, 518, 586–594 CrossRef PubMed.
- W. Luo, F. I. Hai, W. E. Price, M. Elimelech and L. D. Nghiem, Evaluating ionic organic draw solutes in osmotic membrane bioreactors for water reuse, J. Membr. Sci., 2016, 514, 636–645 CrossRef CAS.
- X. Li, Y. Lu and Z. He, Removal of reverse-fluxed ammonium by anammox in a forward osmosis system using ammonium bicarbonate as a draw solute, J. Membr. Sci., 2015, 495, 424–430 CrossRef CAS.
- M. S. Siddique, S. J. Khan, M. A. Shahzad, M. S. Nawaz and N. P. Hankins, Insight into the effect of organic and inorganic draw solutes on the flux stability and sludge characteristics in the osmotic membrane bioreactor, Bioresour. Technol., 2018, 249, 758–766 CrossRef CAS PubMed.
- K. S. Bowden, A. Achilli and A. E. Childress, Organic ionic salt draw solutions for osmotic membrane bioreactors, Bioresour. Technol., 2012, 122, 207–216 CrossRef CAS PubMed.
- P. Zhao, B. Gao, S. Xu, J. Kong, D. Ma, H. K. Shon, Q. Yue and P. Liu, Polyelectrolyte-promoted forward osmosis process for dye wastewater treatment – Exploring the feasibility of using polyacrylamide as draw solute, Chem. Eng. J., 2015, 264, 32–38 CrossRef CAS.
- B.-M. Jun, T. P. N. Nguyen, S.-H. Ahn, I.-C. Kim and Y.-N. Kwon, The application of polyethyleneimine draw solution in a combined forward osmosis/nanofiltration system, J. Appl. Polym. Sci., 2015, 132, 42198 CrossRef.
- Q. Ge, J. Su, G. L. Amy and T.-S. Chung, Exploration of polyelectrolytes as draw solutes in forward osmosis processes, Water Res., 2012, 46, 1318–1326 CrossRef CAS PubMed.
- Y. Yang, M. Chen, S. Zou, X. Yang, T. E. Long and Z. He, Efficient recovery of polyelectrolyte draw solutes in forward osmosis towards sustainable water treatment, Desalination, 2017, 422, 134–141 CrossRef CAS.
- Y. Yang, M. Qin, X. Yang and Z. He, Sustainable operation of osmotic microbial fuel cells through effective reproduction of polyelectrolyte draw solutes facilitated by cathodic pH increase, J. Cleaner Prod., 2017, 168, 1143–1149 CrossRef CAS.
- R. Larson, E. Bookland, R. Williams, K. Yocom, D. Saucy, M. Freeman and G. Swift, Biodegradation of acrylic acid polymers and oligomers by mixed microbial communities in activated sludge, J. Environ. Polym. Degrad., 1997, 5, 41–48 CAS.
- I. L. Alsvik and M.-B. Hägg, Pressure retarded osmosis and forward osmosis membranes: materials and methods, Polymer, 2013, 5, 303–327 Search PubMed.
- L. T. Angenent and S. Sung, Development of anaerobic migrating blanket reactor (AMBR), a novel anaerobic treatment system, Water Res., 2001, 35, 1739–1747 CrossRef CAS PubMed.
- A. Saxena and A. E. García, Multisite ion model in concentrated solutions of divalent cations (MgCl2 and CaCl2): osmotic pressure calculations, J. Phys. Chem. B, 2014, 119, 219–227 CrossRef PubMed.
- B. Verrecht, S. Judd, G. Guglielmi, C. Brepols and J. Mulder, An aeration energy model for an immersed membrane bioreactor, Water Res., 2008, 42, 4761–4770 CrossRef CAS PubMed.
- M. Hamdan, A. O. Sharif, G. Derwish, S. Al-Aibi and A. Altaee, Draw solutions for Forward Osmosis process: Osmotic pressure of binary and ternary aqueous solutions of magnesium chloride, sodium chloride, sucrose and maltose, J. Food Eng., 2015, 155, 10–15 CrossRef CAS.
- M. Xie, L. D. Nghiem, W. E. Price and M. Elimelech, Comparison of the removal of hydrophobic trace organic contaminants by forward osmosis and reverse osmosis, Water Res., 2012, 46, 2683–2692 CrossRef CAS PubMed.
- K. Yogalakshmi and K. Joseph, Effect of transient sodium chloride shock loads on the performance of submerged membrane bioreactor, Bioresour. Technol., 2010, 101, 7054–7061 CrossRef CAS PubMed.
- S. Liu, F. Yang, Z. Gong and Z. Su, Assessment of the positive effect of salinity on the nitrogen removal performance and microbial composition during the start-up of CANON process, Appl. Microbiol. Biotechnol., 2008, 80, 339 CrossRef CAS PubMed.
|
This journal is © The Royal Society of Chemistry 2019 |