Towards more realistic reference microplastics and nanoplastics: preparation of polyethylene micro/nanoparticles with a biosurfactant†
Received
11th September 2018
, Accepted 5th December 2018
First published on 5th December 2018
Abstract
Microplastics are ubiquitous in the aquatic environment and polyethylene (PE) fragments are commonly identified in field samples. Studies are needed to evaluate the toxicity of microplastics, especially for the smallest ones that can be ingested by a wide range of organisms. However, due to a lack of preparation methods, small PE microplastics are not often used in laboratory studies. Here, a simple method was developed for the preparation of PE microparticles using dissolution of PE in toluene followed by emulsification in water. After complete evaporation of water and toluene, a powder of PE microparticles was obtained with radii between 0.2 to 0.80 μm. The protocol could be optimized by adding surfactants like Tween 60 or Tween 80, and the possible use of a solution of microalgae exudates as a biosurfactant was also demonstrated. Planktonic crustaceans were exposed to different types of microparticles to evaluate their bioavailability depending on the surfactant.
Environmental significance
Pollution due to micro and nanoparticles of plastics in the aquatic environment has recently received much attention and its potential impact on aquatic life is an increasing concern. To evaluate the toxicity of micro and nanoplastics, the need for reference particles has recently emerged. Most toxicity studies use commercial nanobeads, mainly polystyrene, with surfactants that can modify their toxicity. In this work, we explored new routes to design micro and nanoparticles of a polymer that would be more realistic for toxicity assessment. Polyethylene was chosen due to its great abundance in natural samples and the particles were prepared without any surfactant or with a surfactant that was chosen to mimic an eco-corona on the particles.
|
Introduction
Pollution caused by plastic debris and fragments has been recognised as a major water quality problem in both fresh and marine water systems.1,2 Degradation of plastic debris in the marine environment leads to the formation of microplastics (<5 mm)3 and potentially nanoplastics4 that were defined as particles smaller than 1 μm.5 Recent investigations show that microplastics are ubiquitous in the marine environment6–8 and that they can interact with the marine biota with possible toxic effects.9–13 The impact of microplastic ingestion on the exposed organism depends on the nature and size of the particles.14 In order to acquire more knowledge on these impacts and to optimise analytical procedures, model particles of different sizes and nature of polymers are necessary.15 However, in the smallest size range (<10 μm), particles of only a few types of polymers are currently available. For this reason, most toxicity tests were reported using PS micro and nanobeads16 whereas polyethylene (PE) and polypropylene (PP) fragments are by far the most common in aquatic environments.17,18 In addition, commercial micro and nanobeads have surfactants at their surface19 which may enhance or at least modify their interaction pathway with aquatic organisms.10,20
So far, only two studies reported protocols to prepare model micro and nanoplastics: Cole et al. (2016)21 used a microtome to produce different types of microfibers and the preparation of PET nanoparticles using laser ablation to mimic nanoplastics was recently proposed.22 Some studies also reported the use of milled polymers to study exposure to aquatic biota,23,24 but obtaining large quantities of small particles by milling can be very difficult and time consuming. The preparation and full characterization of reference polymer materials to mimic micro and nanoplastics may become a necessary step for impact assessment studies.
Concerning polyethylene, there are mainly two different strategies described in the literature to make PE latex particles by polymerisation of ethylene monomers.25,26 The first one is based on the usage of free radical polymerisation of ethylene. The average hydrodynamic radius of the particles prepared by this method was in the range 20–100 nm.26,27 Free radical polymerisation often requires high temperatures and pressures, which is difficult to realize under normal laboratory conditions. Moreover these charged latex particles also contain water soluble initiators and surfactants like cetyltrimethylammonium bromide (CTAB). The second method is catalytic polymerisation of ethylene monomers25,28 for the preparation of a polyethylene aqueous dispersion with diameters between 30 and 300 nm. This method is easier to perform, but Ni(II) complexes were used as catalysts. The presence of initiators, surfactants and metals such as Ni makes all these methods less attractive for further toxicology measurements.
A few studies reported the production of stable dispersions of small polyethylene wax particles.29 Wahle et al. (1998) prepared an oil in water emulsion of a mixture of wax, a hydrophilic non-ionic dispersant, and a hydrophobic co-dispersant. The resulting emulsion was heated to its phase inversion temperature and then cooled down below the inversion temperature. This method produced stable dispersions with particle sizes less than a micrometer.29 The disadvantage of this technique is that the dispersion also contains hydrophilic dispersants and hydrophobic co-dispersants.
Here, we present a new methodology that allows one to prepare small microparticles of PE with a radius between 0.2 μm and 0.8 μm using a toluene-in-water emulsion after total dissolution of PE in the toluene phase. High energy methods like ultra-turrax and acoustic techniques were used to make the PE/toluene-in-water emulsion. To our knowledge, this is the first time that such a method has been used for the preparation of model PE microplastics. Besides providing particles of small size, the advantage of this methodology is that it is possible to produce these particles without any surfactant. It is also much simpler than the techniques mentioned above. Using a surfactant allowed significant yields to be obtained and two types of surfactants were compared (Tween 60 and Tween 80) to increase the produced quantities. Because most surfactants are known to be toxic to aquatic organisms,30 the use of micro-algal exudates as a biosurfactant was also tested. Indeed, microalgae naturally produce extracellular polymeric substances mainly composed of polysaccharides, proteins, nucleic acids and lipids notably to promote their adhesion to surfaces.31 These substances were already described as potential biosurfactants32 and were used here to stabilize the PE particles. The effect of each surfactant on the size, shape and stability of the particles was studied. Finally, preliminary data on the interaction of the so-prepared particles with aquatic organisms were obtained to illustrate their potential bioavailability to aquatic organisms.
1. Materials and methods
1.1. Materials
Low density polyethylene (LDPE) pellets (diameter of 2 mm and density of 920 kg m−3) were obtained from CTTM, (Le Mans, France) and they were used as received. Two surfactants Tween 60 and Tween 80 were purchased from Sigma-Aldrich. The sea salt used in this study was purchased from a local supermarket. Tween 60 is water insoluble and dynamic light scattering measurements (DLS) on aqueous suspensions of this surfactant show the formation of aggregates with a broad distribution of hydrodynamic radii (Rh) between 10 nm and 1 μm. On the other hand, Tween 80 is a water soluble surfactant that forms micelles in aqueous solutions with Rh = 5 nm.
The biosurfactant was prepared by growing fresh water algae Chlamydomonas reinhardtii in Tris-acetate-phosphate culture medium (TAP) for one month. Afterwards, the algae culture medium was centrifuged at 5 × 104g for two hours to remove the algae and other unwanted sediments. The remaining supernatant was used as the biosurfactant without any further purification. The solid content of the supernatant was 0.5 wt% obtained by freeze-drying of a given amount of the culture medium, whereas the solid content of the TAP medium was 0.47%. The light scattering measurements showed that the biosurfactant contained particles with a broad distribution of Rh around an average value of 100 nm. The solid content of the biosurfactant was varied by adding or evaporating water.
1.2. Preparation of PE particles
0.30 g of PE pellets were dissolved in 3.0 g of toluene by refluxing the mixture at 95 °C overnight (∼15 h) in a 100 ml round bottom flask though no solid PE was visible after 4 h. In some cases, 0.03 g of 500 ppm Nile red solution in toluene was added to make the particles fluorescent. Tween 60 or Tween 80 dissolved in toluene was added before refluxing. After reflux, all the polymer was dissolved and the temperature of the PE suspensions was adjusted to 80 °C. To this mixture, water or the biosurfactant was added at 80 °C and the mixture was immediately homogenized utilizing an ultra-turrax (Ultra-Turrax T25, IKA, USA) for two minutes. Then, the resulting emulsions were subjected to ultrasonication (Vibracell, Fisherbioblock, France) for two and a half minutes at 80 °C. Subsequently, the temperature of the emulsion was quickly cooled down from 80 °C to 20 °C, which led to gelation of PE. The total weight of the emulsion was 30 g and the weight fraction of toluene in the emulsion was varied between 10% and 30%. The concentration of Tween in the emulsions was varied between 0.1 wt% and 2 wt% and the solid content of the biosurfactant was varied between 0.5 and 2%. After cooling rapidly by placing the solution in a vessel filled with cold water, residual un-emulsified PE was removed by filtration with Whatman filter papers, whereas a metallic sieve of mesh size 800 μm was used for filtration of emulsions with the biosurfactant. Finally, toluene and water were removed by evaporation and freeze drying in order to obtain a powder of the PE particles.
1.3. Confocal laser scanning microscopy (CLSM)
The shape and size of the particles were investigated by utilizing a Zeiss LSM 800 (Oberkochem, Germany). A water immersion objective lens was used (HCxPL APO 63× NA = 1.2) with a theoretical resolution of 0.1 μm in the x–y plane. The PE particles were fluorescently labelled by adding Nile red that absorbed onto the particles (see section 1.2). The PE particles were dispersed in a very viscous 0.5 wt% xanthan solution to avoid fast movement of the particles during imaging.
1.4. Thermogravimetric analysis (TGA)
Thermogravimetric analysis was carried out using a TA500Q (TA instruments, USA) system. The weight loss during degradation of the powder was determined as a function of the heating temperature. The temperature was varied from 40 °C to 800 °C at a rate of 20 °C per minute.
1.5. Differential scanning calorimetry
Differential scanning calorimetric (DSC) analysis was performed with a DSC Q 100 (TA instruments, USA) system. The heat flow from the samples was measured as a function of temperature from 40 to 250 °C with a heating rate of 20 °C per minute. The crystalline fraction of the gelled PE was determined by taking the ratio between the enthalpy of fusion of PE at 116 °C and the enthalpy of fusion of 100% crystalline PE (285 J g−1).15
1.6. Bioavailability of PE particles in the presence of Daphnia magna
The planktonic crustacean Daphnia magna is a model organism for ecotoxicological studies and was raised in reconstituted water (ISO 6341-1982) with a light/dark cycle (12
:
12 h) and an oxygen renewal system. The measured pH values ranged between 7.5 and 8. 120 animals (ten per flask in triplicate) in 300 mL ISO medium were exposed to 60 mg L−1 (value of EC50, half maximal effective concentration33) PE particles without a surfactant, with Tween 60 and with the biosurfactant. The exposure lasted 72 h, in the absence of food. After 72 h, the intake of particles by the organisms was visualized with microscopy under polarized light. All experiments were performed in compliance with the university's policy on animal use and ethics.
2. Results and discussion
2.1. Emulsification of PE in toluene solutions in water
The methodology to produce PE microplastics presented here involved as a first step the formation of an emulsion of toluene droplets in water with PE dissolved in toluene. The emulsion was prepared by dispersing PE in toluene solution in water at 80 °C using an ultra-turrax and ultrasonication, followed by rapid cooling to 20 °C. It was reported elsewhere that PE dissolved in toluene at high temperatures forms a space filling network during cooling, which leads to gelation of the dispersed droplets.34 DSC measurements of the gelled toluene solution containing 10 wt% PE showed a distinct melting peak from which it was deduced that 32% of the PE was crystalline (see Fig. S1 of the ESI).† We speculate that the crystalline domains act as the physical cross linking units of the PE network since reheating the gels in toluene resulted in a transparent solution.
In the absence of a surfactant, only a relatively small fraction of the PE in toluene solution could be emulsified and the majority of the toluene creamed rapidly to form a turbid top layer. In order to increase the fraction of emulsified toluene, surfactants were added to the toluene solution at different concentrations. Two commercial surfactants (water soluble Tween 80 and water insoluble Tween 60) and a biosurfactant derived from algae were used (see Materials and methods). The amount of the creamed phase was observed to decrease with increasing surfactant concentration. At a given concentration, the biosurfactant yielded the highest amount of emulsified PE in toluene. These results show that the extracellular polymeric substances (EPS) naturally produced by microalgae are at least as efficient as Tween to stabilize the so-prepared emulsions. EPS are mainly composed of polysaccharides, proteins, nucleic acids and lipids and they were already suggested to be biosurfactants32 for synthetic polymers. This kind of biosurfactant is of particular interest in the field of microplastics research as microplastics are known to be rapidly covered by an organic layer as they enter the aquatic environment.10,15
2.2. Characterization of the emulsions
Freshly prepared emulsions of 10 wt% toluene in the presence of 0.1 wt% to 2 wt% Tween 60 were significantly more turbid than emulsions prepared without a surfactant (see Fig. 1). The toluene phase of the emulsions shown in Fig. 1 contained 10 wt% PE. We note that toluene emulsions without PE had the same visual appearance. The difference in turbidity is due to the fact that without any surfactants only a small fraction of the toluene could be emulsified. It was found that without a surfactant, 97% of the PE/toluene solution remained un-emulsified. The un-emulsified fractions were 53%, 57%, and 13% with 2 wt% Tween 60, 2 wt% Tween 80 and 1 wt% biosurfactant, respectively.
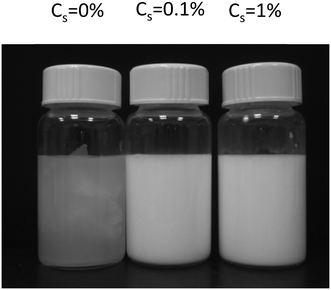 |
| Fig. 1 Freshly prepared emulsions at 3 different Tween 60 concentrations. | |
Fig. 2 shows CLSM images of the emulsions with the 3 different surfactants at different concentrations. The white spots correspond to the fluorescently labelled toluene droplets. The images were analysed with an ImageJ plug-in, “Analyse Particles”, to determine the average radius of the droplets. Fig. 3(top) shows the number concentration (v) of droplets as a function of their radius (R) at different concentrations of Tween 60 (a), Tween 80 (b), and the biosurfactant (c). Each curve in the figure represents an average of at least 4 different images and in most cases one image contains 20 to 30 droplets. From the graph it appears that the number of droplets decreases exponentially with increasing radius. It is important to keep in mind that the minimum radius of the droplets that could be determined by the microscope was limited by its resolution, which was about 100 nm. Hence, here only droplets with a radius ≥ 100 nm were selected for the analysis.
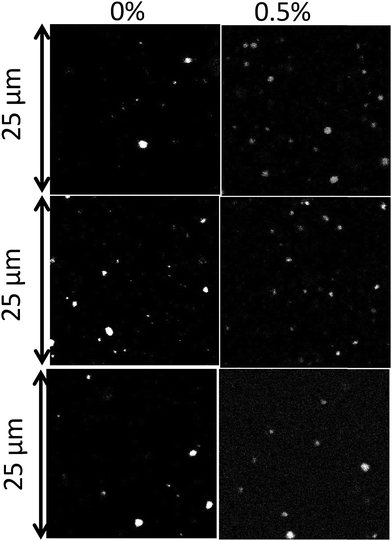 |
| Fig. 2 CLSM images of emulsion droplets with 0.5 wt% surfactant and without a surfactant. From top to bottom: Tween 60, Tween 80 and biosurfactant. | |
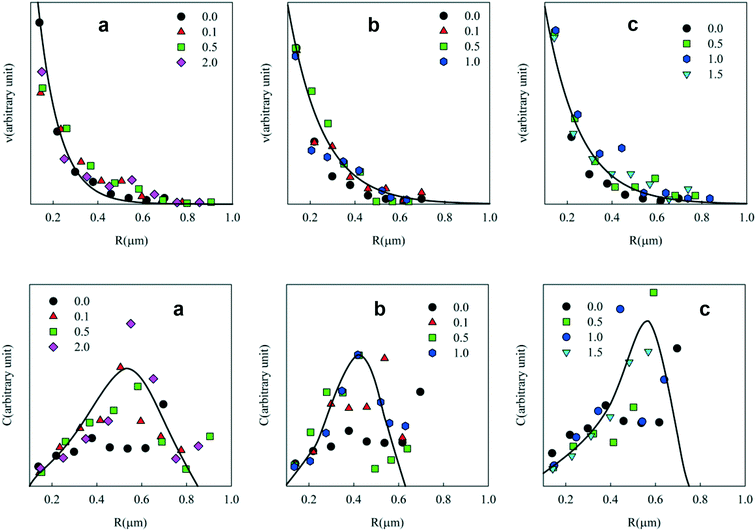 |
| Fig. 3 Number (top) and weight (bottom) concentration distributions of the radius of emulsified PE in toluene droplets dispersed in water at different concentrations (wt%) of (a) Tween 60, (b) Tween 80 and (c) the biosurfactant. The solid lines in the top figures are fits to an exponential decrease, whereas in the bottom figures they represent guides to the eyes. | |
The weight concentration distribution can be calculated as C ∝ v × R3 with the hypothesis that particles are spherical with a constant density independent of R. Fig. 3 (bottom) shows C as a function of the radius at different concentrations of Tween 60, Tween 80 and the biosurfactant. Clearly, the statistics were not good enough to establish the exact shape of the distribution and the solid lines are only meant as a rough indication to guide the eye. However, the results clearly show that in all cases most of the PE was present in droplets with radii between 0.2 and 0.8 μm.
Interestingly, no systematic dependence of the droplet size on the type and concentration of the surfactant or on the PE concentration was observed. Probably, the size of the droplets depended mainly on the applied shear during homogenization. Hideki Sakai et al. (2012) prepared surfactant free emulsions of PE in toluene solutions in water using ultrasonication and they observed that the toluene droplet radii varied between 50 nm and 150 nm depending on the temperature of mixing and the time after the preparation of the emulsion.34 A similar strategy was used by Kamogawa et al.35 for the preparation of emulsions in water of PS in benzene and PS in cyclohexane solutions. The authors reported that the average droplet radius of the emulsions varied between 100 nm and 150 nm. Here, we observed that the average radius of the PE/toluene droplets varied between 200 nm and 800 nm. It is not fully understood how the droplets are stabilized in the absence of surfactants, but it is clear that only a small fraction of toluene droplets is stable. Surfactants are necessary to increase the fraction of stabilized droplets.
2.3. Characterization of PE particles
After removal of toluene and water from the emulsions, a powder of PE particles was obtained. In order to estimate the amount of PE and surfactant in the powder, TGA measurements were done which showed a weight loss in two distinct steps for powders prepared from emulsions containing a surfactant (see Fig. S3 of the ESI).† As expected the fraction of the surfactant in the powder increases with increasing concentration in the toluene phase. The amount of biosurfactant in the powder was relatively small, because most of the material in the biosurfactant solution was not surface active. Notice that the weight loss below 150 °C was negligible, which means that all the toluene had been removed from the particles.
The conversion of PE (ratio between the amount of PE in the powder and the initial amount that was dissolved in toluene) is plotted as a function of the surfactant concentration in Fig. 4. In the absence of a surfactant, the fraction of PE particles in the powder was only a few percent. However, the yield increased steeply with the addition of the surfactant up to 0.35, 0.45 and 0.9 for Tween 60, Tween 80 and the biosurfactant, respectively.
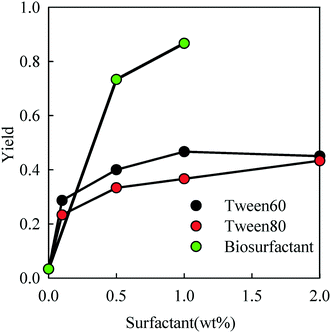 |
| Fig. 4 Weight fraction of PE obtained with different surfactant concentrations. | |
2.4. Size and shape of the PE particles
In Fig. 5, CLSM images of the PE particles are shown as a function of Tween 60 and biosurfactant concentrations. The CLSM images show that particles of different sizes were formed and that the larger particles appeared to be clusters of smaller particles. It is likely that the clusters were formed during the drying process and could not be fully dispersed. Interestingly, the relatively small number of PE particles that were formed without a surfactant could be fully dispersed.
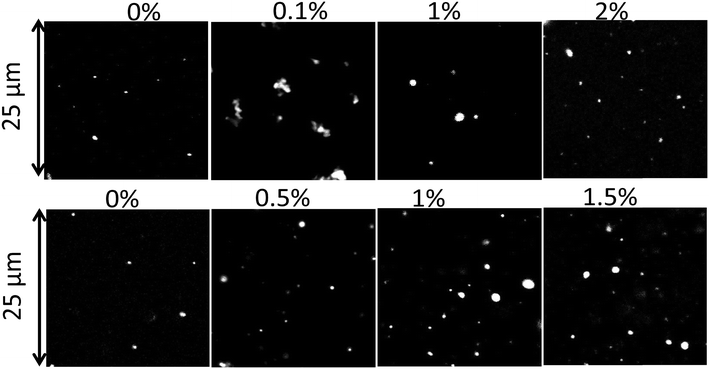 |
| Fig. 5 CLSM images of particles formed with different concentrations of Tween 60 (top) and the biosurfactant (bottom). | |
The number and weight concentration distributions of the particle radii are shown in Fig. 6. As in the case of emulsions, an exponential decrease of the number of particles was observed with increasing radius at different surfactant concentrations (see Fig. 6(top)). The weight distribution shows that most PE was situated in particles with radii between 0.2 and 0.8 μm. A small number of larger clusters were also observed.
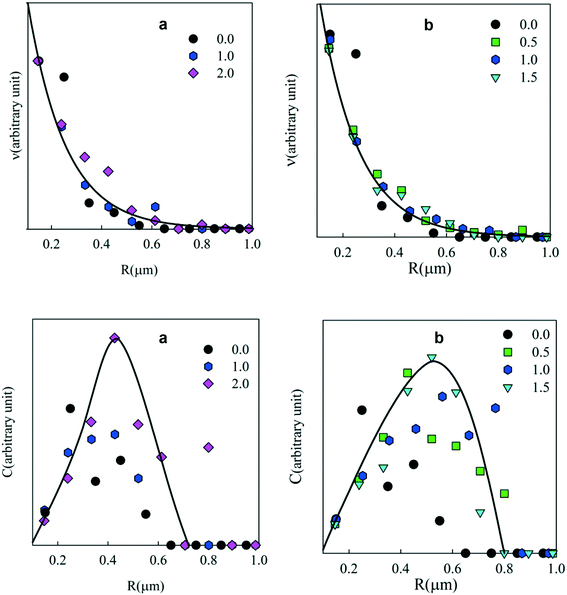 |
| Fig. 6 Number (top) and weight (bottom) concentration distributions of the particle radii at different Tween 60 (a) and biosurfactant (b) concentrations. | |
One might expect that the particles obtained after drying of the emulsion droplets are smaller than the initial droplet size. The size of the particles that were not clustered was close to that of the emulsion droplets before drying. This would imply that the PE network does not shrink much after drying and redispersion. The effect of drying on the PE gel was observed for macroscopic bits of the gelled PE in toluene solution. Fig. 7 shows that the gels decreased by about 25% in size after evaporation of toluene, which means that the PE density increased by about a factor of 2.5 from 100 kg m−3 in the droplets to about 250 kg m−3 in the dried particles. When the gels were immersed in water they did not swell. These results explain why the individual PE particles dispersed in water were similar in size to the emulsion droplets. It follows that the particles are porous containing only about 25 wt% PE, assuming that the effect of drying was the same.
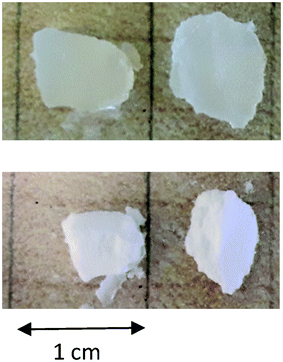 |
| Fig. 7 Images of the PE gels before (top) and after (bottom) the removal of toluene by drying. | |
An attempt was made to prepare denser PE particles by increasing the concentration of PE in toluene up to 30 wt%. This increase did not significantly modify the yield and size of the obtained microparticles (see ESI† Fig. S4 and S5) suggesting that the particle density can be modified by varying the initial PE concentration in the toluene solution.
2.5. PE particle stability
Suspensions of the particles were found to be remarkably stable in Millipore water showing no further aggregation or creaming for a period of at least 3 months. The creaming velocity (v) depends on R and the density difference (Δρ) between the particles and water: Δρ depends strongly on whether the pores of the PE network are filled with air or water. If we assume that 25% of the volume of the particles consists of PE with a density of 920 kg m−3 then the density of particles is 230 kg m−3 if the pores are filled with air and 980 kg m−3 if the pores are filled with water. For particles with R = 350 nm, v = 0.20 μm s−1 if the pores are filled with air and v = 0.005 μm s−1 if the pores are filled with water. Considering that no creaming was visible within 3 months it follows that the pores were filled with water.
The effect of sea salt on the stability of the particles was tested by dispersing the particles first in ultrapure water and subsequently adding sea salt to reach the average concentration of seawater (35 g L−1). The CLSM images of the PE particles with or without surfactants in Millipore (top) water and sea salt water (bottom) are shown in Fig. 8. PE particles without a surfactant formed small clusters in seawater and hence they were not analysed further, but particles containing Tween 60 or the biosurfactant were stable against aggregation (see Fig. 9). This observation shows that surfactant-free particles are stabilized by electrostatic repulsion in salt free water.
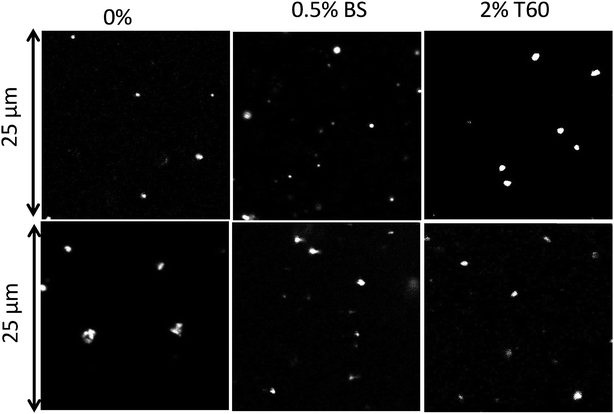 |
| Fig. 8 CLSM images of PE particles obtained without a surfactant, with 0.5 wt% biosurfactant and 2 wt% Tween 60 in Millipore (top) and in sea salt water (bottom). | |
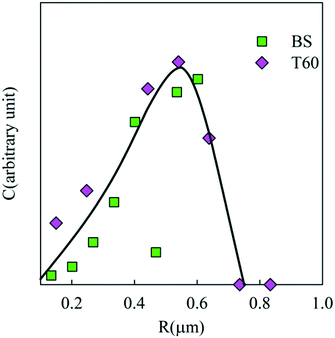 |
| Fig. 9 Weight concentration distribution of the particle radii in seawater for particles with 0.5 wt% biosurfactant and 2 wt% Tween 60. | |
2.6. PE particle interaction with Daphnia magna
After exposure to the particles for 72 hours, the uptake of PE particles and their accumulation in the digestive track of Daphnia magna were recorded using a polarized optical microscope. Fig. 10 shows microscopy images of the digestive track of Daphnia magna that were not exposed (a), or were exposed to particles without a surfactant (b), with Tween 60 (c) or with the biosurfactant (d). No particle uptake was found after exposure to particles with Tween 60. The highest uptake was observed after exposure to particles with the biosurfactant, whereas only a few surfactant free particles of PE were detected in the digestive track. These preliminary results show that the produced PE particles are in the size range of ingestion of most aquatic organisms and that they can be used as model microplastics to evaluate the toxicity of PE. Moreover, this study suggests that the nature of the surfactant has a strong influence on the particle ingestion. The role of an eco-corona at the surface of nanoparticles on their ingestion by biota was recently investigated36,37 and needs to be better controlled in toxicity assessment of micro and nanoplastics.15 The absence of ingestion of particles with Tween 60 could be explained by the capacity of Daphnia magna to detect Tween 60 with a gustative chemoreceptor38 leading to rejection.
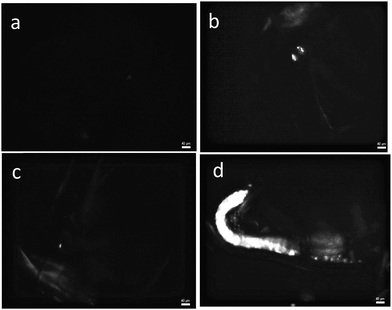 |
| Fig. 10 Microscopy images of Daphnia magna observed in polarized light (×10 magnification) after three days of exposure to PE particles without a surfactant (b), with Tween 60 (c) and with the biosurfactant (d) at a concentration of 60 mg L−1. Image a shows the control situation without particles. The scale bar in the image represents 40 μm. | |
Conclusion
Polyethylene particles with radii between 200 nm and 800 nm can be produced with or without a surfactant using emulsions of toluene in water with PE dissolved in toluene. The yield of PE microparticles was very small in the absence of a surfactant, but could be increased substantially by addition of a surfactant and depended on the type of surfactant. However, the particle size did not depend much on the amount or type of surfactant that was tested (Tween 60, Tween 80 and a biosurfactant). Particles prepared with 10 wt% PE in toluene were porous with a PE content of about 25 wt%, but the PE weight fraction can be varied by varying the PE concentration in toluene during preparation. The same methodology can probably be used to prepare microparticles of other polymers as long as they can be dissolved in a volatile solvent. In addition, instead of using pristine polymers one could use degraded plastics sampled from the environment as the starting material.
The PE particles were stable in aqueous solution for a period of at least 3 months and did not aggregate in water containing sea salt at the average marine concentration. Using a biosurfactant, the presence of an eco-corona at the surface of the particles could be mimicked. These PE particles can be used as model small microplastics and nanoplastics and they might be of interest for toxicological studies on marine biota. Their uptake by Daphnia magna was demonstrated and the preliminary results presented here highlight the importance of the eco-corona on the particle uptake.
Conflicts of interest
There are no conflicts to declare.
Acknowledgements
This work was funded by Ifremer (convention 17/1212947B, project MERLIN MICROPLASTIQUES) and by the ANR CESA (ANR-15-CE34-0006-02, NANOPLASTICS project). We also would like to thank Fréderic Renou for help with the TGA measurements and Mathilde Constans for help with the microplastic exposure experiment.
References
- M. A. Browne, T. Galloway and R. Thompson, Microplastic-an emerging contaminant of potential concern?, Integr. Environ. Assess. Manage., 2007, 3(4), 559–561 CrossRef.
- D. K. A. Barnes, F. Galgani, R. C. Thompson and M. Barlaz, Accumulation and fragmentation of plastic debris in global environments, Philos. Trans. R. Soc., B, 2009, 364(1526), 1985–1998 CrossRef CAS PubMed.
-
C. Arthur, J. Baker and H. BamfordProceedings of the International Research Workshop on the Occurrence, Effects, and Fate of Microplastic Marine Debris. Group. 2009, (January), p. 530 Search PubMed.
- A. Ter Halle, L. Jeanneau, M. Martignac, E. Jardé, B. Pedrono and L. Brach,
et al. Nanoplastic in the North Atlantic Subtropical Gyre, Environ. Sci. Technol., 2017, 51(23), 13689–13697, DOI:10.1021/acs.est.7b03667.
- J. Gigault, A. T. Halle, M. Baudrimont, P.-Y. Pascal, F. Gauffre and T.-L. Phi,
et al. Current opinion: What is a nanoplastic?, Environ. Pollut., 2018, 235, 1030–1034 CrossRef CAS PubMed , Available from: https://www.sciencedirect.com/science/article/pii/S0269749117337247?via%3Dihub.
- A. Cózar, F. Echevarría, J. I. González-Gordillo, X. Irigoien, B. Úbeda and S. Hernández-León,
et al. Plastic debris in the open ocean, Proc. Natl. Acad. Sci. U. S. A., 2014, 111(28), 10239–10244 CrossRef PubMed.
- M. Eriksen, L. C. M. Lebreton, H. S. Carson, M. Thiel, C. J. Moore and J. C. Borerro,
et al. Plastic Pollution in the World's Oceans: More than 5 Trillion Plastic Pieces Weighing over 250,000 Tons Afloat at Sea, PLoS One, 2014, 9(12), e111913, DOI:10.1371/journal.pone.0111913.
- A. L. Lusher, V. Tirelli, I. O'Connor and R. Officer, Microplastics in Arctic polar waters: the first reported values of particles in surface and sub-surface samples, Sci. Rep., 2015, 5, 14947 CrossRef CAS PubMed , Available from: http://europepmc.org/articles/PMC4597356.
- N. N. Phuong, A. Zalouk-Vergnoux, A. Kamari, C. Mouneyrac, F. Amiard and L. Poirier,
et al. Quantification and characterization of microplastics in blue mussels (Mytilus edulis): protocol setup and preliminary data on the contamination of the French Atlantic coast, Environ. Sci. Pollut. Res., 2018, 25(7), 6135–6144 CrossRef CAS PubMed.
- T. S. Galloway, M. Cole and C. Lewis, Interactions of microplastic debris throughout the marine ecosystem, Nat. Ecol. Evol., 2017, 1, 116, DOI:10.1038/s41559-017-0116.
- A. L. Lusher, G. Hernandez-Milian, J. O'Brien, S. Berrow, I. O'Connor and R. Officer, Microplastic and macroplastic ingestion by a deep diving, oceanic cetacean: The True's beaked whale Mesoplodon mirus, Environ. Pollut., 2015, 199, 185–191 CrossRef CAS PubMed.
- A. L. Lusher, M. McHugh and R. C. Thompson, Occurrence of microplastics in the gastrointestinal tract of pelagic and demersal fish from the English Channel, Mar. Pollut. Bull., 2013, 67(1–2), 94–99 CrossRef CAS PubMed , Available from: https://www.sciencedirect.com/science/article/pii/S0025326X12005668?via%3Dihub.
- J. A. Ivar Do Sul and M. F. Costa, The present and future of microplastic pollution in the marine environment, Environ. Pollut., 2014, 185, 352–364, DOI:10.1016/j.envpol.2013.10.036.
- S. L. Wright, R. C. Thompson and T. S. Galloway, The physical impacts of microplastics on marine organisms: A review, Environ. Pollut., 2013, 178, 483–492 CrossRef CAS PubMed.
- I. Paul-Pont, K. Tallec, C. Gonzalez-Fernandez, C. Lambert, D. Vincent and D. Mazurais,
et al. Constraints and Priorities for Conducting Experimental Exposures of Marine Organisms to Microplastics, Front. Mar. Sci., 2018, 5, 252 CrossRef , Available from: https://www.frontiersin.org/articles/10.3389/fmars.2018.00252/full.
- N. N. Phuong, A. Zalouk-Vergnoux, L. Poirier, A. Kamari, A. Châtel and C. Mouneyrac,
et al. Is there any consistency between the microplastics found in the field and those used in laboratory experiments?, Environ. Pollut., 2016, 211, 111–123 CrossRef CAS PubMed.
- K. Enders, R. Lenz, C. A. Stedmon and T. G. Nielsen, Abundance, size and polymer composition of marine microplastics ≥ 10 μm in the Atlantic Ocean and their modelled vertical distribution, Mar. Pollut. Bull., 2015, 100(1), 70–81 CrossRef CAS PubMed , Available from: https://www.sciencedirect.com/science/article/pii/S0025326X15300370?via%3Dihub.
-
C. M. Rochman, A. Andrady, S. Dudas, J. Fabres, F. Galgani and D. Hardesty, et al.Sources, Fate and Effects of Microplastics in the Marine Environment: Part 2 of a Global Assessment, GESAMP Reports & Studies Series 93, 2016, p. 220 Search PubMed.
- M. P. Casado, A. Macken and H. J. Byrne, Ecotoxicological assessment of silica and polystyrene nanoparticles assessed by a multitrophic test battery, Environ. Int., 2013, 51, 97–105 CrossRef CAS PubMed , Available from: https://www.sciencedirect.com/science/article/pii/S0160412012002383?via%3Dihub.
- E. Bergami, S. Pugnalini, M. L. Vannuccini, L. Manfra, C. Faleri and F. Savorelli,
et al. Long-term toxicity of surface-charged polystyrene nanoplastics to marine planktonic species Dunaliella tertiolecta and Artemia franciscana, Aquat. Toxicol., 2017, 189, 159–169 CrossRef CAS PubMed , Available from: https://www.sciencedirect.com/science/article/pii/S0166445X17301625?via%3Dihub.
- M. Cole, A novel method for preparing microplastic fibers, Sci. Rep., 2016, 6, 34519, DOI:10.1038/srep34519.
- D. Magrì, P. Sánchez-Moreno, G. Caputo, F. Gatto, M. Veronesi and G. Bardi,
et al. Laser Ablation as a Versatile Tool To Mimic Polyethylene Terephthalate Nanoplastic Pollutants: Characterization and Toxicology Assessment, ACS Nano, 2018, 12(8), 7690–7700 CrossRef PubMed.
- S. Rainieri, N. Conlledo, B. K. Larsen, K. Granby and A. Barranco, Combined effects of microplastics and chemical contaminants on the organ toxicity of zebrafish (Danio rerio), Environ. Res., 2018, 162, 135–143 CrossRef CAS PubMed.
- J. S. Choi, Y. J. Jung, N. H. Hong, S. H. Hong and J. W. Park, Toxicological effects of irregularly shaped and spherical microplastics in a marine teleost, the sheepshead minnow (Cyprinodon variegatus), Mar. Pollut. Bull., 2018, 129(1), 231–240, DOI:10.1016/j.marpolbul.2018.02.039.
- F. M. Bauers, R. Thomann and S. Mecking, Submicron polyethylene particles from catalytic emulsion polymerization, J. Am. Chem. Soc., 2003, 125(29), 8838–8840 CrossRef CAS PubMed.
- G. Billuart, M. Lansalot, V. Monteil, E. Bourgeat-Lami, M. Lansalot and V. Monteil, Free Radical Emulsion Polymerization of Ethylene, Macromolecules, 2014, 47(19), 6591–6600 CrossRef CAS.
- E. Grau, P. Y. Dugos, J. P. Broyer, C. Boisson, R. Spitz and V. Monteil, Aqueous dispersions of nonspherical polyethylene nanoparticles from free-radical polymerization under mild conditions, Angew. Chem., Int. Ed., 2010, 49(38), 6810–6812 CrossRef CAS PubMed.
- C. H. M. Weber, A. Chiche and G. Krausch, Single Lamella Nanoparticles of Polyethylene, Analysis, 2007, 7(2007), 6–11 Search PubMed.
-
I. G. E. Gore, N. Branch, T. Roman, E. Orange, K. Wayne and R. A. GoreUS Pat., no 19, 1998, (19), pp. 1–6 Search PubMed.
- S. Rebello, A. K. Asok, S. Mundayoor and M. S. Jisha, Surfactants: toxicity, remediation and green surfactants, Environ. Chem. Lett., 2014, 12(2), 275–287, DOI:10.1007/s10311-014-0466-2.
- R. Xiao and Y. Zheng, Overview of microalgal extracellular polymeric substances (EPS) and their applications, Biotechnol. Adv., 2016, 34(7), 1225–1244 CrossRef CAS PubMed , Available from: https://www.sciencedirect.com/science/article/pii/S0734975016301057?via%3Dihub.
- J. Paniagua-Michel Jde, J. Olmos-Soto and E. R. Morales-Guerrero, Algal and microbial exopolysaccharides: New insights as biosurfactants and bioemulsifiers, Adv. Food Nutr. Res., 2014, 73, 221–257 Search PubMed.
- S. Rehse, W. Kloas and C. Zarfl, Short-term exposure with high concentrations of pristine microplastic particles leads to immobilisation of Daphnia magna, Chemosphere, 2016, 153, 91–99 CrossRef CAS PubMed.
- H. Sakai, K. Kamogawa, T. Sakai, T. Umeda, A. Matsumura and K. Sakai,
et al. Stable Surfactant-Free Toluene-Polyethylene-in- Water Emulsion Prepared by Ultrasonication at High Temperature, J. Oleo Sci., 2012, 63(2), 57–63 CrossRef.
- K. Kamogawa, N. Kuwayama, T. Katagiri, H. Akatsuka, T. Sakai and H. Sakai,
et al. Dispersion and Stabilization in Water of Droplets of Hydrophobic Organic Liquids with the Addition of Hydrophobic Polymers, Langmuir, 2003, 19(10), 4063–4069 CrossRef CAS.
- F. Nasser and I. Lynch, Secreted protein eco-corona mediates uptake and impacts of polystyrene nanoparticles on Daphnia magna, J. Proteomics, 2016, 137, 45–51 CrossRef CAS PubMed , Available from: http://www.sciencedirect.com/science/article/pii/S1874391915301214.
- I. L. N. Bråte, M. Blázquez, S. J. Brooks and K. V. Thomas, Weathering impacts the uptake of polyethylene microparticles from toothpaste in Mediterranean mussels (M. galloprovincialis), Sci. Total Environ., 2018, 626, 1310–1318, DOI:10.1016/j.scitotenv.2018.01.141.
- D. C. Peñalva-Arana, M. Lynch and H. M. Robertson, The chemoreceptor genes of the waterflea Daphnia pulex: Many Grs but no Ors, BMC Evol. Biol., 2009, 9(1), 8–11 CrossRef PubMed.
Footnote |
† Electronic supplementary information (ESI) available. See DOI: 10.1039/c8en01005f |
|
This journal is © The Royal Society of Chemistry 2019 |
Click here to see how this site uses Cookies. View our privacy policy here.