Enhanced toxicity of environmentally transformed ZnO nanoparticles relative to Zn ions in the epibenthic amphipod Hyalella azteca†
Received
12th July 2018
, Accepted 5th December 2018
First published on 5th December 2018
Abstract
After release into the aquatic environment, engineered nanomaterials (ENMs) undergo complex chemical and physical transformations that alter their environmental fate and toxicity to aquatic organisms. Hyalella azteca are sediment-dwelling amphipods predicted to have a high exposure level to ENMs and have previously shown to be highly sensitive to ZnO nanoparticles (NPs). To investigate the impacts of environmentally transformed ZnO NPs and determine the route of uptake for these particles, we exposed H. azteca to ZnSO4, ZnO NPs, and environmental aged ZnO NPs which resulted in three types of particles: 30 nm ZnO–Zn3(PO4)2 core–shell structures (p8-ZnO NPs), micron scale hopeite-like phase Zn3(PO4)2·4H2O (p6-ZnO NPs), and ZnS nano-clusters (s-ZnO NPs). Treatments included freshwater, saltwater (3 ppt), and the presence of sediment, with a final treatment where animals were contained within mesh baskets to prevent burrowing in the sediment. Dissolution was close to 100% for the pristine ZnO NPs and phosphate transformed NPs, while s-ZnO NPs resulted in only 20% dissolution in the water only exposures. In the freshwater exposure, the pristine and phosphate transformed ZnO NPs were more toxic (LC50 values 0.11–0.18 mg L−1) than ZnSO4 (LC50 = 0.26 mg L−1) and the s-ZnO NPs (LC50 = 0.29 mg L−1). Saltwater treatments reduced the toxicity of ZnSO4 and all the ZnO NPs. In the presence of sediment, water column concentrations of Zn were reduced to 10% nominal concentrations and toxicity in the sediment with basket treatment was similarly reduced by a factor of 10. Toxicity was further reduced in the sediment only treatments where the sediments appeared to provide a refuge for H. azteca. In addition, particle specific differences in toxicity were less apparent in the presence of sediment. Bioaccumulation was similar across the different Zn exposures, but decreased with reduced toxicity in the saltwater and sediment treatments. Overall, the results suggest that H. azteca is exposed to ZnO NPs through the water column and NP transformations in the presence of phosphate do not reduce their toxicity. Sulfidized ZnO NPs have reduced toxicity, but their similar level of bioaccumulation in H. azteca suggests that trophic transfer of these particles will occur.
Environmental significance
Sediment dwelling organisms, including the amphipod Hyalella azteca, are at particular risk from engineered nanomaterials. Zinc oxide nanoparticles undergo complex transformations in the environment, including pH dependent changes in speciation and solubility and transformations into phosphates, sulfides and carbonates. This is one of the first studies to compare and demonstrate differences in the toxicity of environmentally transformed ZnO nanoparticles. Hyallela azteca are particularly sensitive to phosphate-transformed ZnO nanoparticles, which form in wastewater treatment plants and aquatic environments with enriched phosphate. However, both phosphate and sulfide-transformed ZnO nanoparticles accumulate in H. azteca, thus providing a route for particles to enter the food web.
|
Introduction
Zinc oxide nanomaterials (ZnO NMs) are a class of nanomaterials utilized to provide UV protection in personal healthcare products,1 and for cancer therapies in medicine,2 and they are attractive materials for photovoltaic cells and electrical sensors.3 Modeling studies suggested that the highest environmental concentrations of ZnO nanoparticles (NPs) occur in wastewater treatment facility (WWTF) effluent with current concentrations estimated to be 0.3–0.4 μg L−1 (ref. 4) while measured values were slightly higher.5 These values are expected to climb, especially within sediments,6 as world-wide production of ZnO NPs increases and they are further incorporated into novel products.
As ZnO NPs make their way into the aquatic environment, water chemistry influences their fate and transport,7,8 and the extent of their dissolution.9,10 For example, high ionic strength and alkaline pH cause ZnO NPs to aggregate and settle out of suspension,9 but dissolved organic matter helps to stabilize NP suspensions.11,12 Dissolution of ZnO NPs has been documented as a major determinant of their toxicity;3,13 however, other studies have found that the NPs themselves cause toxicity.14–17 Although the fate and transport of these particles is complex, sedimentation of ZnO NPs is expected in most surface water environments, which will put benthic organisms in these areas at particular risk.18
Environmental transformations of engineered nanomaterials have been shown to have a profound effect on their fate, bioavailability, and effects.19–23 The impact of these transformations often results in a reduction in bioavailability and toxicity; however, in some cases increases in bioaccumulation24 and toxicity have been observed.19,25 Recent studies have demonstrated that ZnO NPs also undergo a complex set of transformations in the environment, including pH dependent changes in speciation and solubility, changes in surface chemistry from binding inorganic and organic ligands, and transformations into phosphates, sulfides, and carbonates.26 For example, during wastewater treatment, it has been demonstrated that ZnO NPs were completely transformed to ZnS, Zn3(PO4)2 and Zn2+ ions were adsorbed to mineral surfaces such as iron oxohydroxides (FeOOH).22,23,27,28 It is often assumed that such transformation products have similar bioavailability and toxicity as corresponding bulk materials, and therefore do not exhibit nano-specific effects.27 However, there is evidence that some of these transformation products may themselves be nanoscale materials. For example, Ma et al. demonstrated that the product of sulfidation of 30 nm ZnO NPs was 30–40 nm clusters of <5 nm ZnS nanoparticles under a variety of conditions.29 In our studies of ZnO NP aging, ∼30 nm ZnO–Zn3(PO4)2 core–shell structures were formed when ZnO was aged in phosphate at slightly alkaline pH.22,23 The same particles dissolved and precipitated as a micron scale hopeite-like phase (Zn3(PO4)2·4H2O) at pH 6. Further, increased bioavailability and toxicity of Zn from the ZnO–Zn3(PO4)2 core–shell structures relative to the micron scale hopeite-like phases was observed in wheat (Triticum aestivum) seedlings.22 The ZnO–Zn3(PO4)2 core–shell structures had similar solubility as Zn3(PO4)2, indicating that the Zn3(PO4)2 shell protected the ZnO core from dissolution.
Sediments are predicted to be an important site for the accumulation of environmentally transformed ZnO NPs; therefore, investigations into the impacts of NPs on sediment dwelling organisms are essential for understanding their environmental risk. Several studies have begun to examine the effects of ZnO NPs to sediment-dwelling and soil organisms,15,30–34 including our study with the epibenthic amphipod Hyalella azteca.35 However, the number of these studies is limited in comparison with aquatic organisms (highlighted in recent reviews3,36). The range in sensitivity of different benthic organisms spans orders of magnitude, while we still have little understanding of the factors that influence the large differences in toxicity across taxa. In addition, very few studies have investigated the impacts of environmentally relevant exposure scenarios, including exposures to transformed ZnO NPs.
The sediment-dwelling amphipod H. azteca is a model species for sediment toxicity studies and was the subject of a recent genome sequencing project that focused on pollution related gene families including ENM responsive genes.37H. azteca's epibenthic ecology, living and scavenging at the sediment surface, puts them at a high risk of exposure.18 Indeed, it has been found to be one of the most sensitive invertebrate species to ENMs,38 including ZnO NPs.35 In addition, it is one of only a few species tested to date to have a higher sensitivity toward ZnO NPs compared with Zn2+.39 Understanding the mechanism for increased NP sensitivity to H. azteca would enable us to predict which organisms in the aquatic environment are at greatest risk for NP exposure and toxicity.40
The objective of the present study was to investigate the toxicity of environmentally transformed ZnO NPs on H. azteca within four different exposure scenarios designed to uncover the mechanisms responsible for NP uptake and toxicity. Based on previous results,35 we hypothesized that H. azteca are exposed to higher concentrations of ZnO NPs compared with Zn2+ from ZnSO4 due to their close proximity to the sediment surface and their scavenging behavior. Therefore, we exposed H. azteca to different ZnO NPs in the presence and absence of sediment. In a separate exposure we prevented them from scavenging by placing them in a mesh basket suspended above the sediments. Finally, we exposed them to the ZnO NPs at a higher salinity to increase aggregation of the particles and their sedimentation onto the beaker surface. We also measured dissolution of the particles and bioaccumulation in the amphipods. Comparing the toxicity and bioaccumulation of the different ZnO NPs under these exposure scenarios allowed us to begin to tease apart the importance of aggregation, sedimentation, and the scavenging behavior of H. azteca on the toxicity of environmentally transformed ZnO NPs.
Materials and methods
Nanoparticle synthesis, transformation, and initial characterization
Pristine and uncoated ZnO NPs were synthesized by wet chemical method using ZnCl2 and NaOH as precursors. The procedures are described by Becheri et al.41 with minor modification. Briefly, the solutions of ZnCl2 (0.2 M) were stirred at 85–90 °C for 15 minutes, followed by dropwise addition of 5 M NaOH (5 M). The precipitate was washed three times with 18 MΩ deionized (DI) water and separated by centrifugation. The pellet was then lyophilized for storage in a dessicator.
Phosphate-aging procedures are based on those we have described previously,23 and sulfide-aging procedures are those used by Ma et al.29 Phosphate-aged ZnO NPs (p6-ZnO NPs and p8-ZnO NPs) were generated by weighing as-synthesized pristine ZnO NPs (approximately 5 mg Zn) into 50 mL polypropylene centrifuge tube and dispersing in a 1.6 mM solution of Na2HPO4 (pH adjusted to 6 and 8, respectively, with HNO3 or NaOH). Sulfidized ZnO NPs (s-ZnO NPs) were generated by weighing as-synthesized pristine ZnO dispersion (approximately 60 mg Zn) into 50 mL centrifuge tube and dispersing in 0.044 M solution of Na2S in He-sparged water. The resulting ratio of S/Zn was approximately 2, which was shown to cause complete transformation of ZnO NPs.29 The particles were re-dispersed in aqueous media by sonicating in a cup horn sonicator for 30 min (Misonix, Newtown CT, USA). The tubes were filled to maximum capacity to minimize the headspace in the tube, then were capped and sealed with parafilm and placed horizontally on a reciprocating shaker for 5 days. Following incubation, tubes were centrifuged at 3320g for 2 h. Supernatants were decanted and pellet was re-suspended in DI water. This process was repeated three times to ensure that all Na2HPO4 or Na2S was removed. After the final washing and centrifugation, pelleted NPs were lypophilized and stored at room temperature in a dessicator until used in toxicity assays.
The primary size and shape of the as-synthesized and transformed ZnO NPs were characterized through transmission electron microscopy (TEM, Jeol 2010F, Tokyo, Japan) at an accelerating voltage of 200 keV. The size distribution of pristine and aged ZnO NPs was determined from more than 100 particles viewed from multiple TEM images across the samples and the diameters of particles were quantified with ImageJ software (http://rsb.info.nih.gov/ij/). The composition and elemental distribution were further mapped using energy dispersive X-ray spectrometry (EDS). The crystal structure of the transformation products was confirmed using powder X-ray diffraction (X'Pert Pro, PANalytical, Malvern, United Kingdom) by comparing to authentic standards.
Zinc concentrations of all stock solutions used to prepare the exposures were determined using inductively coupled plasma mass spectrometry (ICP-MS; Agilent 7500cx, Santa Clara, CA, USA) after digestion of the particles with trace-metal grade HNO3 following EPA methods 3005A42 and 200.8.43 The percent Zn of the pristine and aged samples was also determined by carefully weighing dried particle samples, dissolving in ultra-high purity HNO3, diluting and analyzing zinc content of the sample by ICP-MS. Stoichiometric calculations were used to calculate the percent Zn.
Experimental animals and culturing
Hyalella azteca (US Lab Strain44) were originally obtained from the U.S. Environmental Protection Agency (US EPA), Office of Research and Development Laboratory in Cincinnati, OH, USA, and have been maintained in culture at the University of Massachusetts Boston, USA since 2010. H. azteca were cultured according to the US EPA45 in reconstituted “Duluth-100” water supplemented with 0.05 mg L−1 NaBr and fed three time weekly: desalted Thalassiosira weissflogii (Reed Mariculture, Inc., Campbell, CA) and Tetramin slurry (Tetra Holding, Blacksburg, VA). H. azteca used in all exposure tests were obtained from breeding cultures containing approximately 30 adult H. azteca in 1 L of media. Offspring were collected three times weekly and cultured separately until test initiation. Juvenile H. azteca used in the tests were 7–9 days old.
Toxicity and bioaccumulation tests
The overall study design followed US EPA methods for sediment toxicity testing45 utilizing a 96 h test. However, to determine how exposure conditions affected the toxicity of the NPs, we set up four different exposure scenarios (Fig. 1). These exposure scenarios were designed to determine how different environmental conditions affect the toxicity of ZnO NPs and differentiate between sediment surface exposure versus water exposure. Freshwater exposures provide controlled conditions to measure toxicity and uptake, but because H. azteca are epibenthic organisms, these exposures do not adequately represent environmental conditions. Therefore, sediment exposures were also included to represent realistic environmental conditions where H. azteca either burrow directly in the sediments (sediment only), or inhabit suspended vegetation (sediment with basket). Finally, a 3 ppt exposure was included because H. azteca are able to inhabit slightly saline environments. Under these conditions, we expected the higher ion concentrations to impact the behavior of the NPs, thus potentially altering their toxicity.
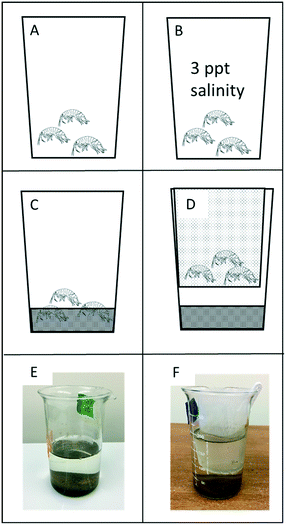 |
| Fig. 1 Exposure conditions for the ZnO NP toxicity tests. Hyallela azteca were exposed to each treatment under four different exposure conditions. In all exposure conditions, the chemical treatments (ZnSO4, ZnO NPs or transformed ZnO NPs) were added to the exposure media prior to adding the H. azteca. (A) Water-only conditions where H. azteca were exposed to each treatment using standard 96 h toxicity test conditions.45H. azteca were provided with a small piece of nylon mesh as substrate. (B) 3 ppt salinity exposure, similar to A, but exposure media was augmented with Instant Ocean (Blacksburg, VA, USA) to obtain a salinity of 3 ppt. (C and E) Sediment conditions where H. azteca were provided with 22 g of clean sediment. (D and F) Sediment-basket conditions where H. azteca were placed within a nylon mesh “basket” suspended above 22 g of clean sediment. | |
Preliminary tests were conducted to determine the maximum salinity that did not impact long-term survival and growth of the H. azteca. Although US EPA45 and Environment Canada46 methods suggest that H. azteca can survive treatments up to 15 ppt salinity, we found that 6 ppt impacted H. azteca growth. Therefore, we chose to use 3 ppt salinity in our tests, which has a sufficient ionic strength to cause aggregation and rapid sedimentation of ZnO NPs.12,47 In addition, we conducted preliminary tests to determine the minimum amount of sediment that would not alter the toxicity of ZnSO4 when compared with 50 ml of sediment. A reduced amount of sediment was desirable to (1) reduce the amount of burrowing of the H. azteca in the tests that may allow them to avoid the exposure, and (2) reduce the amount of sediment needed for analytical tests.
For all tests, test solutions were prepared in Duluth-100 media or Duluth-100 media supplemented with 3 ppt salinity. Stock solutions (1.0 g Zn L−1) of each NP were prepared fresh by adding the appropriate mass of NP to 25 ml of milli-Q water. NPs were thoroughly suspended for 5 min using a Tissue Tearor (Biospec Products, Bartlesville, OK) rotor-type tissue homogenizer at maximum speed. Using this approach, the average hydrodynamic diameter of the ZnO NP suspensions was determined by dynamic light scattering (DLS) to be 650 + 88 nm. The appropriate amount of stock solution was added to Duluth-100 media using a pipet immediately following homogenization and this was allowed to stir for 5 min before preparing test solutions by serial dilution. All test suspensions were prepared by adding the appropriate amount of the more concentrated suspension by pipette and allowing the suspension to stir for 5 min before aliquoting to test beakers or preparing the next test suspension. ZnSO4 stock solutions (Sigma-Aldrich, St. Louis, MO) were prepared by adding the appropriate amount of ZnSO4 to milli-Q water and stirring until fully dissolved. The ZnSO4 stock solutions were placed in an amber bottle and stored at 4 °C for up to 2 months. Test solutions of ZnSO4 were prepared in a similar manner to the ZnO NP suspensions. ZnSO4 was chosen for the Zn control because sulfate was already present in the Duluth-100 exposure media and addition of ZnSO4 did not substantially change the concentration of sulfate already present.
After exposure suspensions (200 ml for water only, 175 ml for sediment exposures) were aliquoted into 300 ml tall form beakers, 10 juvenile H. azteca were added to each beaker. Each exposure condition had four replicate beakers and a minimum of five concentrations and an unexposed control were tested for each chemical. Exposure beakers were placed in a 25 °C environmental chamber for 96 h. After 48 h, beakers were removed and if possible, survivors were observed and counted without removing the animals from the beakers. After 96 h, surviving H. azteca were recorded and water, surviving animals, and sediments were collected. Temperature, pH, DO, conductivity, water hardness, and alkalinity were measured at the beginning end of each exposure. Because ammonium levels may result from bacterial activity in the sediment exposures, ammonium was also measured at the end of the exposures to ensure that levels did not contribute to toxicity. In all exposures, temperature ranged between 22.0–24.5 °C, DO ranged between 6.0–8.3 mg L−1 and pH ranged between 7.7–8.5. Conductivity ranged between 377–413 μS cm−1, water hardness ranged between 100–120 mg L−1, and alkalinity ranged between 85–95 mg L−1 in freshwater treatments. The following values were higher in the salinity treatments: conductivity: 5.67–5.78 mS cm−1, water hardness: 650–810 mg L−1, and alkalinity: 91–100 mg L−1. Ammonium was 0 ppb in the treatments without sediment and ranged between 600–1000 ppb in treatments with sediment, well below levels that induce toxicity in H. azteca.48
Salinity treatments.
Duluth-100 media was adjusted to 3 ppt with Instant Ocean Sea Salt (Spectrum Brands, Blacksburg, VA). Salinity was adjusted by adding approximately 2.9 g L−1 of Instant Ocean until the conductivity equaled 5.6 mS cm−2.
Sample collection and Zn analysis
Water samples.
2 ml samples of exposure water were collected at the initiation and conclusion of each test. Test initiation samples were collected while test suspensions were stirring prior to aliquoting into test beakers. Samples collected at the conclusion of the test represented a pooled sample from all replicates. Water was collected with a pipet after gentle stirring, pooled into a single beaker, and stirred for 5 min prior to collecting the 2 ml samples. To determine the amount of dissolution in the exposures, duplicate samples were collected. One sample “total Zn” was preserved upon collection with the addition of trace metal grade HNO3, while the second sample, “dissolved Zn,” was centrifuged for 1.5 h at 21
330 × g. The top 1.8 ml was removed from the centrifuged sample, placed in a metal-free cyrovial and preserved with nitric acid. Zinc concentrations in both “total Zn” and “dissolved Zn” water samples were determined by ICP-MS after open-vessel, microwave-assisted digestions using HNO3 following US EPA methods 3005A42 and 200.8.43
Sediments.
After H. azteca and baskets, if present, were removed from the beakers, sediment was collected using a 25 ml hand pipettor. Sediment was collected into a 15 ml metal-free centrifuge tube and stored at 4 °C until analysis. To determine total Zn concentrations in sediment, about 0.25 g of oven-dried sediment samples were weighed and digested using closed-vessel microwave with concentrated HNO3 following US EPA method 3052.49 The Zn concentrations in the digestates were determined by ICP-MS following EPA method 200.8.43
Bioaccumulation in Hyalella azteca
H. azteca were collected at the conclusion of each test for bioaccumulation measurements. All surviving individuals from each treatment were placed in a plastic weigh boat and washed three times with milli-Q water. Animals were then placed in a metal-free cyrovial and the tubes were dried at 60 °C overnight. Animals were not depurated in clean water prior to collection. Although this means that unabsorbed Zn in the gut of H. azteca was included in the total accumulated Zn, it provided a better prediction of the amount of Zn that could be transferred to higher trophic levels.
Only surviving H. azteca from the treatments were collected for total Zn analysis. For the majority of the samples, a total of 9–10 individuals were successfully collected from each replicate and were collectively weighed and analyzed for total Zn. At the highest concentrations tested in each treatment, mortality affected the number of individuals available for bioaccumulation analysis, and some samples only contained 2–3 individuals. The H. azteca samples were oven-dried at 60 °C. The dried samples were weighed using an ultra-microbalance and transferred into a 1.5 ml fluoropolymer microcentrifuge tube. Ultra-pure HNO3 (Aristar Ultra, VWR, Radnor, PA, USA) were added to each vial. The vial was loosely capped and heated on a hot-block at 100 °C for 2-h until a clear digest was obtained. The Zn concentration in the digestates was analyzed by ICP-MS as described above and normalized to the weight of the combined individuals in each replicate.
Statistical analysis
Toxicity data.
Survival data were fit to non-linear regression models using the DRC package (3.0-1) in R (v. 3.4.0) to construct dose–response curves and calculate LC50 values. Log-logistic and Weibull models provided the best fit for the data based on comparison of the most commonly used models in DRC.50 Goodness of fit was confirmed for each model using “modelFit.” LC50 values, confidence intervals, and standard error were calculated using the “ED” command.
LC50 values were compared across the different treatments using the LC50 ratio method described in Wheeler et al.51 The DRC command “comped” was used to determine if log transformed 95% confidence intervals for the LC50 ratio crossed 0. If the confidence intervals include 0, the null hypothesis is not rejected and the two LC50 values are equal. If the confidence intervals do not include 0, the LC50 are significantly different (p < 0.05).
Bioaccumulation data were evaluated as means ± SD. Statistically significant differences among means were determined using one-way ANOVA followed by the Student–Newman–Keuls procedure being used for post hoc multiple comparisons.
Results
Synthesis and characterization of transformed ZnO NPs
We obtained pure zincite-structured ZnO NP powder as confirmed by powder XRD (Fig. S1†). The Zn content as measured by ICP-MS was 79.2% as compared to the theoretical percentage of 80.3%, indicating relatively high purity. The primary particle size of the as-synthesized ZnO NPs was 22.4 ± 2.8 nm (Fig. 2A). The p6-ZnO NPs were 44.5% Zn; the p8 ZnO NPs were 74.9% Zn; and the sZnO NPs were 59.7% Zn. The theoretical Zn percentages for Zn3(PO4)2 and Zn3(PO4)2 4H20 and ZnS are 50.8, 48.5, and 67.1%, respectively. The Zn, O, P and S content of the particles was confirmed by EDS (Fig. S2†). This demonstrates relatively complete conversion of Zn to a mixture of Zn3(PO4)2 and Zn3(PO4)2 4H2O for the p6-ZnO NPs and a thin shell of amorphous Zn3(PO4)2, for the p8 treatment, as we previously demonstrated using a combination of TEM, Zn K-edge X-ray absorption near edge structure spectroscopy (XANES) and 31P magic angle spinning nuclear magnetic resonance (31P MAS-NMR).23 However, we found that in this case, there was less residual ZnO present in the p6-ZnO NPs product as compared to our previous work as demonstrated by the Zn content, XRD data, and TEM images (Fig. 2). The primary particle size of the ZnO starting material in our previous study was larger (34.2 ± 6.8 nm) than in this study (22.3 ± 2.8 nm). This is one possible explanation for the more complete conversion of Zn from the particles to Zn3(PO4)2 in the p6 treatment. The solubility of particles generally increases with decreasing particle size, and this transformation involves dissolution of ZnO and reprecipitation of Zn3(PO4)2. The TEM data, Zn content, and XRD data demonstrate relatively complete conversion of ZnO to ZnS in the s-ZnO NP treatment (Fig. 2D). Their composition and morphology (clusters of ultra-fine ZnS NPs) are as described in Ma et al.29
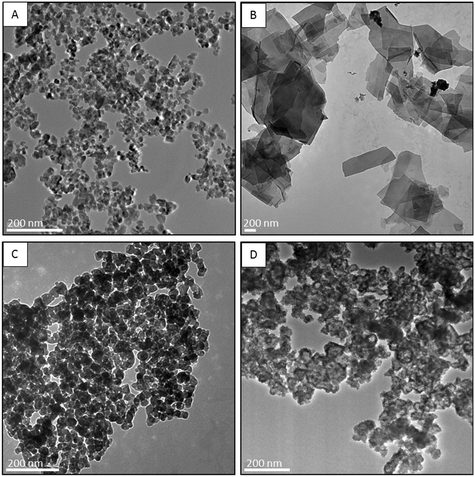 |
| Fig. 2 Transmission electron micrographs of images of (A, ZnO NPs) and aged ZnO NPs (B, p6-ZnO NPs, C, p8-ZnO NPs, D, s-ZnO NPs). Scale bars represent 200 nm. | |
Dissolution and sediment deposition
Zn concentrations were determined in water and sediments following the 96 h exposures. The total and dissolved Zn measured in the water are reported in Table S1.† Measured total Zn was close to nominal values in the freshwater and saltwater exposures (ranged between 75–112% in FW; 72–104% in SW); however, in the presence of sediment, the total Zn in water ranged from 6–23% of nominal values. In the sediment exposures, almost 90% of the Zn was deposited on or sequestered to the sediments (Table S2†). Zinc concentrations in exposures with or without baskets were similar in both the water and sediments, thus suggesting that the burrowing activity of the H. azteca did not impact Zn distribution. In addition, sediment absorption/deposition was similar across all ZnO NP exposures and ZnSO4 exposures.
In the water only exposures, the as-synthesized ZnO NPs, p6-ZnO NPs, and p8-ZnO NPs underwent almost complete dissolution at concentrations below 1.0 mg L−1 over the 96 h exposure (Table S1,†Fig. 3). In the sediment exposures, these NPs also showed a similar or even higher concentration of dissolved Zn compared to ZnSO4; although, there is an overall decrease in the amount of dissolved Zn likely due to precipitation (which is expected at thermodynamic equilibrium at the pH values of the exposure media), or absorption onto suspended sediment particles. In contrast, the s-ZnO NPs had a much lower rate of dissolution (∼20%) in all the exposures (Fig. 3).
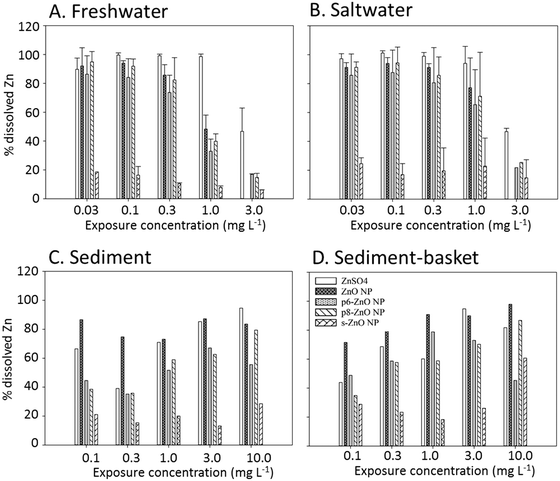 |
| Fig. 3 Dissolution of ZnO NP expressed as the percent of dissolved Zn compared to total Zn measured at the conclusion of the 96 h exposures. (A) Freshwater, water-only treatment, (B) salinity treatment with 3 ppt exposure media, (C) sediment treatment containing clean sediment (D) sediment-basket treatment where H. azteca were suspended in a nylon mesh basket above clean sediment. Concentrations are expressed as mg Zn L−1. Error bars represent standard deviation (n = 4). | |
Toxicity test results
Non-linear regression analysis was used to construct concentration-response relationships for each treatment in the different exposure conditions. All exposure concentrations are expressed as mg Zn L−1. Overall, the shape of the concentration–response curves were similar for all treatments (Fig. 4); however, differences in the toxicity of ZnSO4 and the environmentally-transformed ZnO NPs were shown by the shift of the curves to the right or left in some exposure conditions. For example, in the freshwater exposures (Fig. 4A), the concentration–response curves for the ZnO NPs and both phosphate-transformed particles were shifted toward the left compared with ZnSO4, thus revealing that these NPs are more toxic than Zn2+ alone. The reduced toxicity of the s-ZnO NPs was also apparent by the right shift of the dose–response curves in the sediment exposures (Fig. 4C and D).
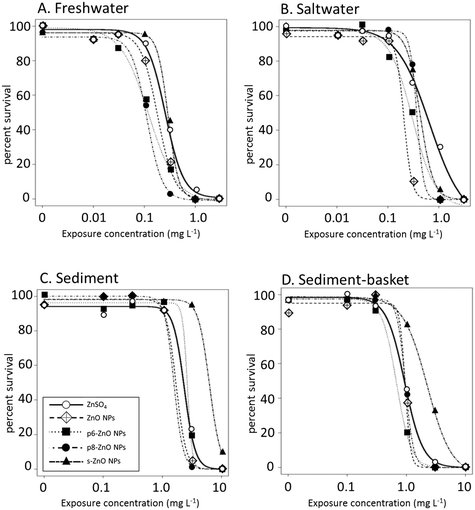 |
| Fig. 4 Concentration response curves illustrating the comparative toxicity of ZnSO4 and each ZnO NP treatment under the four different exposure conditions. (A) Freshwater, water-only treatment, (B) salinity treatment with 3 ppt exposure media, (C) sediment treatment containing clean sediment, (D) sediment-basket treatment where H. azteca were suspended in a nylon mesh basket above clean sediment. Dose response curves were fitted to survival data using non-linear regression models. | |
Concentration–response curves were also used to determine lethal median concentration (LC50) values for each treatment in all four exposure conditions. In the freshwater conditions, the phosphate particles exhibited the highest toxicity (Table 2) with LC50 values less than half the LC50 value for ZnSO4. As seen previously,35 the as-synthesized ZnO NPs were also significantly more toxic than ZnSO4 under these conditions. However, the differences between the treatments decreased in the other exposure conditions. In saltwater, only the ZnO NP and p6-ZnO NP were less toxic than ZnSO4 and in both sediment exposures none of the particles were more toxic than ZnSO4. In contrast, s-ZnO NPs showed decreased toxicity in the presence of sediment with an LC50 two to four times higher than ZnSO4 and the other particles.
Table 2 Comparative toxicity of ZnSO4 and ZnO NPs under different exposure conditions. The lethal median concentration (LC50) values in mg Zn per L are shown with 95% confidence intervals for ZnSO4 and each nanoparticle under four different exposure conditions. Sediment exposures were conducted in freshwater allowing H. azteca to freely burrow in sediment (sediment) or placed in a mesh basket above the sediment preventing them from burrowing (sediment-basket). For each condition, different superscript letters represent statistically different LC50 values based on the LC50 ratio method described by Wheeler et al. (2006).51 Numbers in bold are used to highlight differences from the ZnSO4 treatment
|
Freshwater |
Saltwater (3 ppt) |
Sediment |
Sediment-basket |
ZnSO4 |
0.26 (0.22–0.30)a |
0.66 (0.45–0.86)a |
2.35 (1.56–3.14)a |
0.94 (0.80–1.01)a |
ZnO NP |
0.18 (0.14–0.22)
b
|
0.23 (0.16–0.30)
b
|
1.55 (1.26–1.84)a |
0.96 (0.74–1.19)a |
p6-ZnO NP |
0.13 (0.10–0.15)
c
|
0.30 (0.24–0.35)
b
|
2.58 (0.60–4.57)a |
0.75 (0.53–0.96)a |
p8-ZnO NP |
0.11 (0.10–0.13)
c
|
0.36 (0.00–1.15)a,b |
1.57 (1.23–1.90)a |
0.96 (0.50–1.41)a |
s-ZnO NP |
0.29 (0.26–0.32)a |
0.48 (0.43–0.52)a |
7.12 (5.48–8.76)
b
|
2.27 (1.98–2.57)
b
|
The presence of sediment reduced the toxicity of all treatments including the ZnSO4 and the NP exposures. Because the reduction of toxicity was seen in both sediment treatments, the sediments likely acted as a sink for the toxicants, possibly sequestering Zn2+ from the exposure media or heteroaggregating with the particles. All of the treatments showed increased toxicity when H. azteca were placed in a basket above the sediment compared with the sediment only exposures. This implies that the H. azteca were not exposed to the NPs as they scavenge on the sediment surface. Instead, as the H. azteca burrowed in the sediments, the sediments acted as a refuge, limiting the exposure of the H. azteca to Zn2+ or the NPs.
Bioaccumulation
Following the 96 h exposures, total Zn was measured in the surviving H. azteca to compare the amount of bioaccumulation across ZnO NP exposures and among the different treatments. Zn concentrations were also measured in H. azteca prior to test initiation to determine background levels of this essential metal. Average Zn concentrations in control H. azteca following the 96 h exposures ranged from 60–62 mg Zn per kg dry mass, which were not different from initial values (59 + 10 mg Zn per kg dry mass). This indicates that H. azteca did not accumulate additional Zn from the untreated media or sediments during the exposure in the control treatment. However, in all the ZnSO4 and ZnO NP exposures, Zn accumulated in H. azteca to concentrations that were significantly higher than initial values. These concentrations also increased relative to the increasing concentrations of Zn in the exposures (Fig. 5). In general, there was little difference in the amount of Zn accumulated in H. azteca between the different ZnSO4 and ZnO NP exposures at each concentration. The one exception was the much higher bioaccumulation seen for the s-ZnO NPs in the saltwater treatment at all tested concentrations (Fig. 5B).
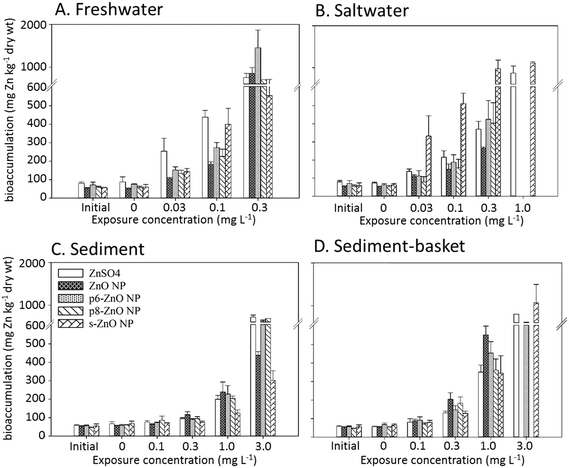 |
| Fig. 5 Bioaccumulation of Zn measured in H. azteca following 96 h exposures to ZnSO4 or environmental transformed ZnO NPs. (A) Freshwater, water-only treatment, (B) salinity treatment with 3 ppt exposure media, (C) sediment treatment containing clean sediment (D) sediment-basket treatment where H. azteca were suspended in a nylon mesh basket above clean sediment. Error bars represent standard deviation (n = 4). Missing bars in some treatments (i.e. saltwater 1.0 mg L−1, sediment-basket, 3.0 mg L−1) are because there was no survival in the exposure at that concentration. | |
Bioaccumulation occurred to a greater extent in the water only exposures compared with the sediment exposures (Fig. 5). Interestingly, in the sediment exposures, H. azteca which were held in a basket and unable to borrow in the sediment accumulated more Zn than those able to scavenge and burrow in the sediment despite the higher Zn concentration in the sediment compared to the water.
Comparison of bioaccumulation and toxicity
Whole-body Zn concentrations partially explained the differences in toxicity seen in each of the treatments. Across all the treatments, we generally observed high mortality (>40%) when whole-body Zn concentrations reached 200–500 mg Zn per kg dry mass. Bioaccumulation occurred to a greater extent in the freshwater treatment where toxicity was greatest. In addition, the sediment-basket treatment caused a higher level of Zn bioaccumulation than in the sediment treatment, which also matched the trend in toxicity. Although the bioaccumulation data support the differences in toxicity across the freshwater, saltwater, and sediment treatments, it is not able to explain particle specific differences in toxicity. In the freshwater exposure where we see the greatest difference in toxicity to the different particles (Table 2), bioaccumulation does not show a similar trend (Fig. 6). For example, the phosphate-aged particles (p6-ZnO NPs and p8-ZnO NPs) are more toxic than ZnSO4 but these particles are not accumulated to a greater extent. In all the treatments, the toxicity of the s-ZnO NPs was 2–5 times lower than the other NPs (Table 2), but in many of the treatments, the Zn bioaccumulation is similar to the other particles and in the saltwater treatment, the s-ZnO NP exposure results in the highest bioaccumulation (Fig. 6).
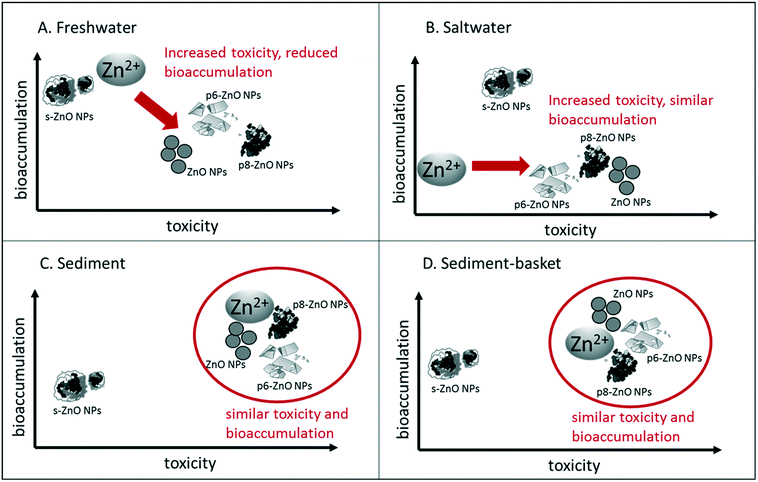 |
| Fig. 6 Relative toxicity and bioaccumulation of Zn ions and ZnO NPs in different exposure scenarios. (A) Freshwater, water-only treatment, (B) salinity treatment with 3 ppt exposure media, (C) sediment treatment containing clean sediment (D) sediment-basket treatment where H. azteca were suspended in a nylon mesh basket above clean sediment. In each figure, bioaccumulation increases toward the top of the figure and toxicity increases toward the right. Toxicity and bioaccumulation were summarized from Table 2 and Fig. 5 and are shown relative to the different treatments (Zn ions or the different ZnO NPs). Treatments that group together had similar bioaccumulation and toxicity in the given exposure, while treatments that are separated had a distinct bioaccumulation and/or toxicity pattern. Direction of arrows illustrates the difference in toxicity and bioaccumulation of the majority of the particles in comparison with the Zn ion. Circles represent similarities in toxicity and bioaccumulation between NPs and the Zn ions. In each figure, s-ZnO NPs have a distinct pattern in comparison with the other particles. | |
Discussion
Recent studies have shown that ENMs undergo complex environmental transformations after entering aquatic systems,8,18 emphasizing the importance of studying environmentally-realistic exposures to transformed NPs. In the present study, we reconfirmed that H. azteca is more sensitive to ZnO NPs compared to Zn2+ in water only exposures, and that under some conditions, environmentally-transformed particles are more toxic. However, in the presence of sediment, there are no differences and only one type of transformed ZnO NP, the s-ZnO NP, is less toxic than Zn2+. ZnO NPs were less toxic in saltwater and sediment exposures, suggesting that water-only exposures overestimate the risk to H. azteca. The differences across the exposure conditions were well explained by the decrease in Zn bioaccumulation; however, bioaccumulation did not explain the differences in the toxicity across the ZnO NPs.
Environmental transformations of ZnO NPs altered toxicity
Despite large differences in solubility constants for the phosphate-transformed ZnO NPs, we observed similar levels of dissolution compared with pristine particles. In addition, particle-specific differences in toxicity (e.g., LC50 values) could not be attributed to the dissolution or bioaccumulation data, which was measured at the end of the 96 h exposures. Transformation of ZnO NPs in the presence of phosphate reduces their solubility;52 however, the structure and composition of the transformation products is strongly dependent upon the pH at which the reaction takes place.23 We used phosphate-transformed ZnO NPs following the methods of Ranthnayake et al. at pH 6 and pH 8.23 At pH 6, the ZnO NPs were transformed into larger sheets consisting primarily of hopeite-like Zn3(PO4)2 and other Zn3(PO4)2 phases. In the pH 8 treatment, particles had a ZnO–Zn3(PO4)2 core–shell structure, where the shell consists of amorphous Zn3(PO4)2.23 Because Zn3(PO4)2 has a very low solubility, we predicted low dissolution of p6-ZnO NPs and p8-ZnO NPs. However, we observed similar dissolution in the exposures to the as-syntheized ZnO NPs particles (Fig. 3), and toxicity of the p6-ZnO NPs and p8-ZnO NPs were similar or greater than the toxicity of the pristine ZnO NPs (Table 2, Fig. 6). The similarity in dissolution may be reflective of the low exposure concentrations in the study. Specifically, given a Ksp value of 9 × 10−33 for Zn3(PO4)2 in DI water, the dissolved Zn concentration at saturation would be 0.030 mg L−1. This suggests that differences in dissolution kinetics, which were not reflected in the present study, may be important. For example, if dissolution is more rapid, the area under the concentration versus time curve is greater than if it is slower, resulting in a greater time-integrated exposure to dissolved ions.
In contrast, sulfidized particles (s-ZnO NPs) had lower dissolution and lower toxicity compared with the ZnO NPs and pZnO NPs. Lower dissolution is expected. Given a Ksp value of 3 × 10−23 for ZnS in DI water, the dissolved Zn concentration at saturation would be 1 × 10−9 mg L−1. The s-ZnO NPs used in this study were produced following the methods of Ma et al. by aging ZnO NPs in the presence of sulfide.29 These particles had a structure consisting of clusters of ultra-fine ZnS. They were smaller than ZnO NPs (2.5–5 nm), had reduced dissolution, and increased aggregation compared to pristine particles.29 These expectations were met in our s-ZnO NPs exposures. Zn dissolution was <20% in almost all the s-ZnO NPs exposures and they exhibited reduced toxicity compared with the other NPs.
While transformation processes are important for environmental ENM exposures, there are still relatively few studies that have investigated the toxicity of environmentally-transformed NMs. One area of focus has been on how wastewater treatment plants (WWTPs) influence chemical and physical transformations of NPs,53 and the resulting toxicity of effluents and biosolids containing transformed NPs. Chronic toxicity and metal uptake was investigated in the legume Medicago truncatula when grown on biosolids amended with ENMs. They found that biosolids amended with ZnO NPs resulted in higher Zn accumulation in plants and increased chronic toxicity, although the speciation of Zn in the biosolids was similar for NP-amended and bulk/dissolved metal-amended biosolids.24 Several additional studies have investigated the toxicity of sulifidized Ag NPs, which are the major Ag end product from chemical transformations in WWTPs.53 Sulfidation of AgNPs has been shown to reduce its toxicity to a range of aquatic and terrestrial species including fish,25,54 aquatic plants,25 and the nematode Caenorhabditis elegans.25,55 Reductions in toxicity are correlated with reduced dissolution of Ag+ and lower bioacculumation.25,55 However, other studies in terrestrial plants and arthropods have emphasized that sulifidized Ag NPs are still bioavailable and have been shown to accumulate in these species.56,57 These results are similar to our findings for s-ZnO NPs. Sulifidation of the ZnO NPs decreased dissolution and toxicity, but still resulted in a similar level of bioaccumulation to the other ZnO NPs in most treatments. Therefore, there a potential for s-ZnO NPs to be transferred to higher trophic levels, and it is not clear what form of Zn the predators that consume H. azteca would be exposed to.
Given the relatively low solubility of ZnS particles, it is likely that most effects were caused by particle-specific mechanisms. Similarly, the greater toxicity of the particles compared with equivalent concentrations of dissolved Zn, also suggest particle-specific mechanisms. There are possible effects of particles caused by damage to external body surfaces that are not related to dissolution and uptake of ions. For example, toxicity to the gut epithelium, as seen with C. elegans exposed to CeO NPs, may alter digestive processes.58 Similarly, Starnes et al. demonstrated that Ag2S nanoparticles damage the C. elegans cuticle and cause toxicity in the absence of uptake of Ag into the internal tissues. Particles which are internalized and remain intact can also cause effects such as unfolded protein responses.59 Finally, phototoxicity is a possibility for ZnO NPs.17
Salinity and sediment reduces the toxicity of ZnO NPs
The ionic strength of natural waters is known to impact the stability of NPs causing greater aggregation and sedimentation of particles.12 ZnO NPs were shown to undergo aggregation and rapid sedimentation at NaCl concentrations above 15 meq L−1 (or 0.9 ppt).47 In the present study, particle concentrations were far too low to measure aggregation by dynamic light scattering. Although it is predicted that increased particle size and sedimentation will decrease ZnO NP bioavailability, because H. azteca are epibenthic species, we hypothesized that sedimentation would increase their level of exposure and possibly the toxicity of the particles. However, the toxicity of Zn2+ decreases in seawater or brackish water given greater competition of cations such as Ca2+ for sites of uptake as well as greater concentrations of ligands which bind Zn2+.60 As expected, the toxicity of ZnSO4 was reduced 2-fold in the saltwater exposures. Most of the ZnO NPs particles also exhibited reduced toxicity. Because salinity did not increase the toxicity of the ZnO NPs, it appears that increased sedimentation does not pose a risk for H. azteca and that the larger aggregate particle size may reduce absorption and bioavailability. This was reflected in the reduced bioaccumulation in the saltwater treatment compared to the freshwater. However, dissolution of the ZnO NPs was similar in the saltwater exposures and the freshwater exposures, suggesting that the main impact in the salinity treatment was the presence of additional cations that compete for uptake.
The presence of sediment reduced concentrations of Zn in the water column across all the exposures, which resulted in decreased toxicity in both the sediment-basket and sediment only exposures. Experiments by Li et al. demonstrated that ZnO NPs bind to sediment particles and show low mobility through sand packed columns.61 Phosphate increased the mobility of Zn suggesting that phosphate transformed ZnO NPs may have a lower affinity for sediment. Despite this prediction, the total Zn measured in the water column was similar across the exposures and represented approximately 10% of the nominal Zn added. The remaining 90% of Zn was accounted for in the sediment measurements. The Zn partitioning was similar, but slightly lower, to a previous study that found 2% Zn in the water column and 97% in the sediments. The increased partitioning to the sediments was likely due to the high salinity of their exposure media.31 In the sediment-basket treatment, the toxicity across all treatments was reduced about 10-fold in comparison to the freshwater only treatment, which correlates with the 10-fold reduction in total Zn found in the water column. Toxicity was further reduced in the sediment only treatment where H. azteca were able to scavenge on the sediment surface and burrow. Instead of increasing their exposure and toxicity, the presence of sediment appeared to act as refuge enabling the amphipods to escape from the exposure as shown in the reduced bioaccumulation in this treatment (Fig. 5). Overall, these results strongly suggest that H. azteca are exposed to ZnO NPs through the water column and not by scavenging on or within the sediment.
Previous studies investigating the toxicity of ZnO NPs to sediment-dwelling organisms focused on bioaccumulation in clams, polychaetes, and crustaceans. Two studies in marine systems used isotopically-labeled Zn to investigate partitioning and bioaccumulation in marine organisms. Both studies revealed rapid sedimentation of ZnO NPs with increased concentrations found in the top layers of the sediment.30,31 Uptake of isotopically labeled Zn was seen in the clam Scrobicularia plana and ragworm Nereis diversicolor exposed to ZnO NPs and was correlated with chronic toxicity (increased GST activity, reduced feeding); however, this level of Zn was only about 10% of the Zn that accumulated in these species from their natural environment.30 Considering the low concentration used in their study (similar to the 0.3 mg L−1 exposure in our study), the levels of bioaccumulation were similar. Larner et al. used isotopically labeled 68ZnO NPs to trace their distribution in a saltwater-sediment exposure. Amphipods accumulated more 68Zn in the ZnO NP exposures than in bulk ZnO exposures, but bioaccumulation was similar to the ZnCl2 exposure.31 Two additional studies investigated the toxicity of sediment exposures to crustaceans. Hanna et al. showed that dissolved Zn2+ in pore water released from ZnO NP-amended sediment was sufficient to cause the observed toxicity to the marine amphipod Leptocheirus plumulosus,32 suggesting that similar to our study, Zn uptake is through the water column or pore water. Jośko et al. exposed Heterocypris incongruens to ZnO NPs in two sediments with different amounts of organic carbon content. The sediment with lower organic content (i.e., sandier) had higher bioavailable Zn and caused increased mortality. The authors suggested that organic matter causes immbolization of the metals in the sediments and makes them less bioavailable.62
Toxicity data suggest complex route of uptake
For metals, H. azteca primarily respond to the concentrations of metals found in the overlaying waters, and not metals bound to sediments.63 Therefore, for Zn2+, we expected that bioaccumulation and toxicity would be strongly correlated with water column concentrations of Zn. However, for metal oxide NPs, the route of exposure is less clear. H. azteca are found primarily on the sediment surface preferring epiphytic algae as a primary food source.63 If sedimentation of NPs occurs, we would expect H. azteca to ingest these particles as they scavenge on the sediment surface. Cross et al.18 also explains that in ponds and slow moving rivers, NP deposition will lead to high concentrations in the “benthic boundary layer,” or the top layer of sediment that is resuspended and mixed through bioturbation and may result in a very high level of exposure for epibethic organisms like H. azteca. Dietary exposure has also been shown to be a significant source of NP exposure for sediment dwelling organisms. For example, the freshwater snail L. stagnalis was able to assimilate over 85% of ZnO delivered through a diatom food source.64 Many benthic and epibenthic organisms feed on the biofilm that develops on surficial sediment,65 which retains ZnO NPs and aids in the accumulation and transfer of ZnO NPs to consumers.66 When taken together, these studies suggest that the higher toxicity of the ZnO NPs compared to Zn2+ could be due to sedimentation and higher exposure at the sediment surface. However, results from our present study contradict this prediction.
Several lines of evidence strongly suggest that H. azteca are accumulating Zn through the water column. First, in the saltwater treatment, we expected an increase in the sedimentation of ZnO NPs due to the higher ionic strength. In this treatment, we observed a decrease in toxicity, suggesting that the sedimentation and removal of NPs from the water column was related to decreased toxicity. Secondly, the 5–10-fold reduction in toxicity in the sediment with basket treatment compared to freshwater treatment corresponds well to the reduction of Zn in the water column to about 10% nominal in the sediment treatments. Finally, the sediment with basket treatments resulted in higher toxicity to ZnSO4 and all ZnO NP exposures when compared to the sediment only treatment. These organisms were not exposed to the settling NPs, but only to those suspended or dissolved in the water column.
Many studies have suggested that ZnO NP toxicity is governed primarily through dissolution and subsequent exposure to Zn2+.39 For example, ZnO NP toxicity was primarily related to Zn2+ dissolution in an early study with the green algae Pseudokirchneriella subcapitata.13 Several studies in aquatic crustaceans supported this mechanism of toxicity,67,68 but it was not found to be universal and often depended on the specific particles investigated and the environmental parameters of the exposure media.3
Since dissolution was high in all the ZnO NP exposures except for the s-ZnO NPs which exhibited reduced toxicity, our results suggested that dissolution was playing a large role in NP toxicity. However, this could not explain the greater toxicity seen in the ZnO NP exposures compared to ZnSO4 in the water only exposures. In addition, dissolution was a poor predictor of bioacummulation, which is most apparent when comparing the ZnSO4 and s-ZnO NPs. In most treatments, the bioaccumulation of the s-ZnO NPs was equivalent or greater than in the ZnSO4 exposures despite the low level of s-ZnO NP dissolution. In summary, although the water column is the primary route of exposure for the ZnO NPs, dissolution does not account for the differences in toxicity. Other factors must be involved that govern the differential toxicity. Because all the measurements in the present study were taken after 96 hours, the differences in dissolution and bioaccumulation may occur at early time points, and these differences could drive the differential toxicity of the ZnO NPs. It is also possible that this is coupled with particle-specific modes of toxicity. Other studies have shown particle-specific effects, even when high levels of dissolution have been observed. For example, Lopes et al. found chronic effects to feeding and growth in NP exposed Daphnia magna not observed in the Zn2+ treatments despite high levels of dissolution.69 Given the high sensitivity of H. azteca, understanding the factors that contribute to ZnO NP toxicity in this species will enable us to predict which aquatic organisms are most susceptible to ZnO NPs and likely other ENMs as well.
Conclusions
Once NPs enter the aquatic environment, they undergo physical and chemical transformations that alter their toxicity. ZnO NPs can react with phosphate and sulfide within wastewater treatment facilities or in aquatic environments that are enriched for phosphate. This study investigated whether transformation processes alter the toxicity of ZnO NPs to one of the most sensitive aquatic species, the epibenthic crustacean H. azteca, under different environmental exposure scenarios. Phosphate-transformed particles were at least as toxic as pristine particles and Zn ions, and under some conditions they caused enhanced toxicity, while sulfide-transformed particles were generally less toxic. All ZnO NPs were taken up by H. azteca and bioaccumulated in this important prey species, suggesting that H. azteca may act as vector for trophic transfer to fish.
Toxicity results from the different exposure scenarios provided new insight into the route of exposure and potential uptake mechanisms for the transformed ZnO NPs. Sediment exposures reduced the toxicity and bioaccumulation of all ZnO NPs and Zn2+, consistent with the overlying water being the primary route of exposure for NPs. However, different levels of dissolution and bioaccumulation could not fully explain the differences in toxicity across the particles. For example, the sulfidized ZnO NPs showed the least toxicity across all exposure scenarios, but had similar or higher levels of bioaccumulation. Phosphate-transformed particles showed high levels of dissolution, but were twice as toxic as Zn ions in the water-only exposures. These results suggest that other mechanisms are responsible for governing toxicity, possibility related to differences in uptake kinetics and internal absorption. For example, we could propose that ZnO NPs provide a mechanism of enhanced uptake and accumulation within the gut of H. azteca, similar to a “Trojan-horse”-like mechanism.70 Differences in the internal solubility of the particles and absorption into tissues may then govern particle specific toxicity.
Uptake kinetics studies may shed light on these proposed mechanisms explaining the differential toxicity of the NPs. Recent studies have utilized stable isotope methods to track the bioaccumulation and elimination of ZnO NPs over time allowing for calculations of uptake and elimination rate constants.15,31,64 Similar studies in H. azteca would allow us to understand differences in the rate of uptake of the different particles as well as how much of the particles are actually absorbed by the organisms. The sediment community is expected to experience some of the highest levels of NP exposure; therefore, understanding the relative toxicity of environmentally realistic NP exposures to this community is critical for NP risk assessment. Our study suggests that the processes governing NP uptake and absorption maybe key for predicting risks to the sediment community, and studies focusing on the relevant risk of environmentally-transformed particles will provide increased realism to our risk assessments.
Conflicts of interest
The author declare no conflicts of interest.
Acknowledgements
This work was supported by a National Science Foundation grant (CBET: 1437409) to H. C. P. and J. M. U. K. M. M. was supported by an NSF Integrative Graduate Education and Research Traineeship (IGERT) DGE-1249946 and K. M. M. and S. A. were supported by Sanofi Genzyme Doctoral and Undergraduate Research Fellowships awarded to the College of Science and Mathematics at the University of Massachusetts Boston. Special thanks to Albert Armstrong for his editorial assistance on this manuscript.
References
- Z. A. Lewicka, A. F. Benedetto, D. N. Benoit, W. W. Yu, J. D. Fortner and V. L. Colvin, The structure, composition, and dimensions of TiO2 and ZnO nanomaterials in commercial sunscreens, J. Nanopart. Res., 2011, 13, 3607–3617 CrossRef CAS.
- C. Hanley, J. Layne, A. Punnoose, K. M. Reddy, I. Coombs, A. Coombs, K. Feris and D. Wingett, Preferential killing of cancer cells and activated human T cells using ZnO nanoparticles, Nanotechnology, 2008, 19, 295103 CrossRef PubMed.
- H. Ma, P. L. Williams and S. A. Diamond, Ecotoxicity of manufactured ZnO nanoparticles--a review, Environ. Pollut., 2013, 172, 76–85 CrossRef CAS PubMed.
- F. Gottschalk, T. Sonderer, R. W. Scholz and B. Nowack, Modeled environmental concentrations of engineered nanomaterials (TiO2, ZnO, Ag, CNT, Fullerenes) for different regions, Environ. Sci. Technol., 2009, 43, 9216–9222 CrossRef CAS PubMed.
- F. Gottschalk, T. Sun and B. Nowack, Environmental concentrations of engineered nanomaterials: review of modeling and analytical studies, Environ. Pollut., 2013, 181, 287–300 CrossRef CAS PubMed.
- K. L. Garner, S. Suh and A. A. Keller, Assessing the Risk of Engineered Nanomaterials in the Environment: Development and Application of the nanoFate Model, Environ. Sci. Technol., 2017, 51, 5541–5551 CrossRef CAS PubMed.
- M. N. Moore, Do nanoparticles present ecotoxicological risks for the health of the aquatic environment?, Environ. Int., 2006, 32, 967–976 CrossRef CAS PubMed.
- G. V. Lowry, K. B. Gregory, S. C. Apte and J. R. Lead, Transformations of nanomaterials in the environment, Environ. Sci. Technol., 2012, 46, 6893–6899 CrossRef CAS PubMed.
- D. Zhou and A. A. Keller, Role of morphology in the aggregation kinetics of ZnO nanoparticles, Water Res., 2010, 44, 2948–2956 CrossRef CAS PubMed.
- R. B. Reed, D. A. Ladner, C. P. Higgins, P. Westerhoff and J. F. Ranville, Solubility of nano-zinc oxide in environmentally and biologically important matrices, Environ. Toxicol. Chem., 2012, 31, 93–99 CrossRef CAS PubMed.
- Y. Zhang, Y. Chen, P. Westerhoff and J. Crittenden, Impact of natural organic matter and divalent cations on the stability of aqueous nanoparticles, Water Res., 2009, 43, 4249–4257 CrossRef CAS PubMed.
- A. A. Keller, H. Wang, D. Zhou, H. S. Lenihan, G. Cherr, B. J. Cardinale, R. Miller and Z. Ji, Stability and aggregation of metal oxide nanoparticles in natural aqueous matrices, Environ. Sci. Technol., 2010, 44, 1962–1967 CrossRef CAS PubMed.
- N. M. Franklin, N. J. Rogers, S. C. Apte, G. E. Batley, G. E. Gadd and P. S. Casey, Comparative toxicity of nanoparticulate ZnO, bulk ZnO, and ZnCl2 to a freshwater microalga (Pseudokirchneriella subcapitata): the importance of particle solubility, Environ. Sci. Technol., 2007, 41, 8484–8490 CrossRef CAS PubMed.
- H. C. Poynton, J. M. Lazorchak, C. A. Impellitteri, M. E. Smith, K. Rogers, M. Patra, K. A. Hammer, H. J. Allen and C. D. Vulpe, Differential gene expression in Daphnia magna suggests distinct modes of action and bioavailability for ZnO nanoparticles and Zn ions, Environ. Sci. Technol., 2011, 45, 762–768 CrossRef CAS PubMed.
- J. Fabrega, R. Tantra, A. Amer, B. Stolpe, J. Tomkins, T. Fry, J. R. Lead, C. R. Tyler and T. S. Galloway, Sequestration of zinc from zinc oxide nanoparticles and life cycle effects in the sediment dweller amphipod Corophium volutator, Environ. Sci. Technol., 2012, 46, 1128–1135 CrossRef CAS PubMed.
- W.-M. Li and W.-X. Wang, Distinct biokinetic behavior of ZnO nanoparticles in Daphnia magna quantified by synthesizing 65Zn tracer, Water Res., 2013, 47, 895–902 CrossRef CAS PubMed.
- H. Ma, N. Kabengi, P. Bertsch, J. Unrine, T. Glenn and P. Williams, Comparative phototoxicity of nanoparticulate and bulk ZnO to a free-living nematode Caenorhabditis elegans: the importance of illumination mode and primary particle size, Environ. Pollut., 2011, 159, 1473–1480 CrossRef CAS PubMed.
- R. K. Cross, C. Tyler and T. S. Galloway, Transformations that affect fate, form and bioavailability of inorganic nanoparticles in aquatic sediments, Environ. Chem., 2015, 12, 627–642 CrossRef CAS.
- A. J. Bone, B. P. Colman, A. P. Gondikas, K. M. Newton, K. H. Harrold, R. M. Cory, J. M. Unrine, S. J. Klaine, C. W. Matson and R. T. Di Giulio, Biotic and Abiotic Interactions in Aquatic Microcosms Determine Fate and Toxicity of Ag Nanoparticles: Part 2-Toxicity and Ag Speciation, Environ. Sci. Technol., 2012, 46, 6925–6933 CrossRef CAS PubMed.
- J. M. Unrine, B. P. Colman, A. J. Bone, A. P. Gondikas and C. W. Matson, Biotic and Abiotic Interactions in Aquatic Microcosms Determine Fate and Toxicity of Ag Nanoparticles. Part 1. Aggregation and Dissolution, Environ. Sci. Technol., 2012, 46, 6915–6924 CrossRef CAS PubMed.
- B. Collin, E. Oostveen, O. V. Tsyusko and J. M. Unrine, Influence of Natural Organic Matter and Surface Charge on the Toxicity and Bioaccumulation of Functionalized Ceria Nanoparticles in Caenorhabditis elegans, Environ. Sci. Technol., 2013, 48, 1280–1289 CrossRef.
-
S. Rathnayake, Transformations, Bioavailability and Toxicity of Manufactured ZnO Nanomaterials in Wastewater, MS Thesis, University of Kentucky, 2013 Search PubMed.
- S. Rathnayake, J. M. Unrine, J. Judy, A.-F. Miller, W. Rao and P. M. Bertsch, Multitechnique investigation of the pH dependence of phosphate induced transformations of ZnO nanoparticles, Environ. Sci. Technol., 2014, 48, 4757–4764 CrossRef CAS.
- J. D. Judy, D. H. McNear, C. Chen, R. W. Lewis, O. V. Tsyusko, P. M. Bertsch, W. Rao, J. Stegemeier, G. V. Lowry, S. P. McGrath, M. Durenkamp and J. M. Unrine, Nanomaterials in Biosolids Inhibit Nodulation, Shift Microbial Community Composition, and Result in Increased Metal Uptake Relative to Bulk/Dissolved Metals, Environ. Sci. Technol., 2015, 49, 8751–8758 CrossRef CAS PubMed.
- C. Levard, E. M. Hotze, B. P. Colman, A. L. Dale, L. Truong, X. Y. Yang, A. J. Bone, G. E. Brown, R. L. Tanguay, R. T. Di Giulio, E. S. Bernhardt, J. N. Meyer, M. R. Wiesner and G. V. Lowry, Sulfidation of Silver Nanoparticles: Natural Antidote to Their Toxicity, Environ. Sci. Technol., 2013, 47, 13440–13448 CrossRef CAS PubMed.
-
H. C. Poynton and W. E. Robinson, in Green Chemistry: An Inclusive Approach, ed. B. Torok and T. Dransfield, Elsevier, Cambridge, MA, 2018, ch. 3.7, pp. 291–315, DOI:10.1016/B978-0-12-809270-5.00012-1.
- E. Lombi, E. Donner, E. Tavakkoli, T. W. Turney, R. Naidu, B. W. Miller and K. G. Scheckel, Fate of Zinc Oxide Nanoparticles during Anaerobic Digestion of Wastewater and Post-Treatment Processing of Sewage Sludge, Environ. Sci. Technol., 2012, 46, 9089–9096 CrossRef CAS PubMed.
- R. Ma, C. Levard, J. D. Judy, J. M. Unrine, M. Durenkamp, B. Martin, B. Jefferson and G. V. Lowry, Fate of Zinc Oxide and Silver Nanoparticles in a Pilot Wastewater Treatment Plant and in Processed Biosolids, Environ. Sci. Technol., 2013, 48, 104–112 CrossRef PubMed.
- R. Ma, C. Levard, F. M. Michel, G. E. Brown, Jr. and G. V. Lowry, Sulfidation Mechanism for Zinc Oxide Nanoparticles and the Effect of Sulfidation on Their Solubility, Environ. Sci. Technol., 2013, 2527–2534, DOI:10.1021/es3035347.
- P. E. Buffet, C. Amiard-Triquet, A. Dybowska, C. Risso-de Faverney, M. Guibbolini, E. Valsami-Jones and C. Mouneyrac, Fate of isotopically labeled zinc oxide nanoparticles in sediment and effects on two endobenthic species, the clam Scrobicularia plana and the ragworm Hediste diversicolor, Ecotoxicol. Environ. Saf., 2012, 84, 191–198 CrossRef CAS PubMed.
- F. Larner, Y. Dogra, A. Dybowska, J. Fabrega, B. Stolpe, L. J. Bridgestock, R. Goodhead, D. J. Weiss, J. Moger, J. R. Lead, E. Valsami-Jones, C. R. Tyler, T. S. Galloway and M. Rehkamper, Tracing bioavailability of ZnO nanoparticles using stable isotope labeling, Environ. Sci. Technol., 2012, 46, 12137–12145 CrossRef CAS PubMed.
- S. K. Hanna, R. J. Miller, D. Zhou, A. A. Keller and H. S. Lenihan, Accumulation and toxicity of metal oxide nanoparticles in a soft-sediment estuarine amphipod, Aquat. Toxicol., 2013, 142-143, 441–446 CrossRef CAS PubMed.
- S. W. Wong, P. T. Leung, A. B. Djurisic and K. M. Leung, Toxicities of nano zinc oxide to five marine organisms: influences of aggregate size and ion solubility, Anal. Bioanal. Chem., 2010, 396, 609–618 CrossRef CAS PubMed.
- S. Manzo, A. Rocco, R. Carotenuto, L. Picione Fde, M. L. Miglietta, G. Rametta and G. Di Francia, Investigation of ZnO nanoparticles' ecotoxicological effects towards different soil organisms, Environ. Sci. Pollut. Res., 2011, 18, 756–763 CrossRef CAS PubMed.
- H. C. Poynton, J. M. Lazorchak, C. A. Impellitteri, B. Blalock, M. E. Smith, K. Struewing, J. Unrine and D. Roose, Toxicity and transcriptomic analysis in Hyalella azteca suggests increased exposure and susceptibility of epibenthic organisms to zinc oxide nanoparticles, Environ. Sci. Technol., 2013, 47, 9453–9460 CrossRef CAS.
- J. Hou, Y. Wu, X. Li, B. Wei, S. Li and X. Wang, Toxic effects of different types of zinc oxide nanoparticles on algae, plants, invertebrates, vertebrates and microorganisms, Chemosphere, 2018, 193, 852–860 CrossRef CAS PubMed.
- H. C. Poynton, S. Hasenbein, J. B. Benoit, M. S. Sepulveda, M. F. Poelchau, D. S. Hughes, S. C. Murali, S. Chen, K. M. Glastad, M. A. Goodisman, J. H. Werren, J. H. Vineis, J. L. Bowen, M. Friedrich, J. Jones, H. M. Robertson, R. Feyereisen, A. Mechler-Hickson, N. Mathers, C. E. Lee, J. K. Colbourne, A. D. Biales, J. S. Johnston, G. A. Wellborn, A. J. Rosendale, A. G. Cridge, M. C. Munoz-Torres, P. A. Bain, A. R. Manny, K. M. Major, F. N. Lambert, C. D. Vulpe, P. Tuck, B. J. Blalock, Y.-Y. Lin, M. E. Smith, H. Ochoa-Acuña, M.-J. M. Chen, C. P. Childers, J. Qu, S. Dugan, S. L. Lee, H. Chao, H. Dinh, Y. Han, H. Doddapaneni, K. C. Worley, D. M. Muzny, R. A. Gibbs and S. Richards, The Toxicogenome of Hyalella azteca: a model for sediment ecotoxicology and evolutionary toxicology, Environ. Sci. Technol., 2018, 52, 6009–6022 CrossRef CAS PubMed.
- S. Li, L. K. Wallis, D. S. A, H. Ma and D. J. Hoff, Species sensitivity and dependence on exposure conditions impacting the phototoxicity of TiO2 nanoparticles to benthic organisms, Environ. Toxicol. Chem., 2014, 33, 1563–1569 CrossRef CAS PubMed.
- D. A. Notter, D. M. Mitrano and B. Nowack, Are nanosized or dissolved metals more toxic in the environment? A meta-analysis, Environ. Toxicol. Chem., 2014, 33, 2733–2739 CrossRef CAS PubMed.
- T. M. Scown, R. van Aerle and C. R. Tyler, Review: Do engineered nanoparticles pose a significant threat to the aquatic environment?, Crit. Rev. Toxicol., 2010, 40, 653–670 CrossRef CAS PubMed.
- A. Becheri, M. Dürr, P. L. Nostro and P. Baglioni, Synthesis and characterization of zinc oxide nanoparticles: application to textiles as UV-absorbers, J. Nanopart. Res., 2008, 10, 679–689 CrossRef CAS.
-
USEPA, Acid digestion of waters for total recoverable or dissolved metals for analysis by FLAA or ICP spectroscopy, United States Environmental Protection Agency, Cincinnati, OH, USA, 1992 Search PubMed.
-
USEPA, Method 200.8: Determination of trace elements in waters and wastes by inductively coupled plasma-mass spectrometry, United States Environmental Protection Agency, Cincinnati, OH, USA, 1994 Search PubMed.
- K. Major, D. J. Soucek, R. Giordano, M. J. Wetzel and F. Soto-Adames, The common ecotoxicology laboratory strain of Hyalella azteca is genetically distinct from most wild strains sampled in Eastern North America, Environ. Toxicol. Chem., 2013, 32, 2637–2647 CAS.
-
USEPA, Methods for measuring the toxicity and bioaccumulation of sediment-associated contaminants with freshwater invertebrates, US Environmental Protection Agency, 2000 Search PubMed.
-
E. Canada, Biological test method: Test for survival and growth in sediment and water using the freshwater amphipod Hyalella azteca., EPS 1/RM/33, Science and Technology Branch, Ottawa, Ontario, 2nd edn, 2013 Search PubMed.
- Y.-H. Peng, Y.-C. Tsai, C.-E. Hsiung, Y.-H. Lin and Y.-H. Shih, Influence of water chemistry on the environmental behaviors of commercial ZnO nanoparticles in various water and wastewater samples, J. Hazard. Mater., 2017, 322, 348–356 CrossRef CAS.
- U. Borgmann, Chronic toxicity of ammonia to the amphipod Hyalella azteca; importance of ammonium ion and water hardness, Environ. Pollut., 1994, 86, 329–335 CrossRef CAS.
-
USEPA, Method 3052: Microwave assisted acid digestion of siliceous and organically based matrices, United States Environmental Protection Agency, Washington, DC, 1996 Search PubMed.
- C. Ritz, F. Baty, J. C. Streibig and D. Gerhard, Dose-response analysis using R, PLoS One, 2015, 10, e0146021 CrossRef PubMed.
- M. W. Wheeler, R. M. Park and A. J. Bailer, Comparing median lethal concentration values using confidence interval overlap or ratio tests, Environ. Toxicol. Chem., 2006, 25, 1441–1444 CrossRef CAS PubMed.
- J. Lv, S. Zhang, L. Luo, W. Han, J. Zhang, K. Yang and P. Christie, Dissolution and microstructural transformation of ZnO nanoparticles under the influence of phosphate, Environ. Sci. Technol., 2012, 46, 7215–7221 CrossRef CAS PubMed.
- R. Ma, C. M. Levard, J. D. Judy, J. M. Unrine, M. Durenkamp, B. Martin, B. Jefferson and G. V. Lowry, Fate of zinc oxide and silver nanoparticles in a pilot wastewater treatment plant and in processed biosolids, Environ. Sci. Technol., 2013, 48, 104–112 CrossRef.
- G. P. Devi, K. B. A. Ahmed, M. S. Varsha, B. Shrijha, K. S. Lal, V. Anbazhagan and R. Thiagarajan, Sulfidation of silver nanoparticle reduces its toxicity in zebrafish, Aquat. Toxicol., 2015, 158, 149–156 CrossRef CAS PubMed.
- D. L. Starnes, J. M. Unrine, C. P. Starnes, B. E. Collin, E. K. Oostveen, R. Ma, G. V. Lowry, P. M. Bertsch and O. V. Tsyusko, Impact of sulfidation on the bioavailability and toxicity of silver nanoparticles to Caenorhabditis elegans, Environ. Pollut., 2015, 196, 239–246 CrossRef CAS PubMed.
- P. Wang, N. W. Menzies, E. Lombi, R. Sekine, F. P. C. Blamey, M. C. Hernandez-Soriano, M. Cheng, P. Kappen, W. J. G. M. Peijnenburg, C. Tang and P. M. Kopittke, Silver sulfide nanoparticles (Ag2S-NPs) are taken up by plants and are phytotoxic, Nanotoxicology, 2015, 9, 1041–1049 CrossRef PubMed.
- K. Sebastian, K. Ralf, S. Karsten, W. Claus, H. Henner and S. Christian, Silver nanoparticles in sewage sludge: Bioavailability of sulfidized silver to the terrestrial isopod Porcellio scaber, Environ. Toxicol. Chem., 2018, 37, 1606–1613 CrossRef PubMed.
- D. A. Arndt, E. K. Oostveen, J. Triplett, D. Allan Butterfield, O. V. Tsyusko, B. Collin, D. L. Starnes, J. Cai, J. B. Klein, R. Nass and J. M. Unrine, The role of charge in the toxicity of polymer-coated cerium oxide nanomaterials to Caenorhabditis elegans, Comp. Biochem. Physiol., Part C: Toxicol. Pharmacol., 2017, 201, 1–10 CAS.
- O. V. Tsyusko, J. M. Unrine, D. Spurgeon, E. Blalock, D. Starnes, M. Tseng, G. Joice and P. M. Bertsch, Toxicogenomic responses of the model organism Caenorhabditis elegans to gold nanoparticles, Environ. Sci. Technol., 2012, 46, 4115–4124 CrossRef CAS PubMed.
- G. K. Bielmyer, J. B. Bullington, C. A. Decarlo, S. J. Chalk and K. Smith, The effects of salinity on acute toxicity of zinc to two euryhaline species of fish, Fundulus heteroclitus and Kryptolebias marmoratus, Integr. Comp. Biol., 2012, 52, 753–760 CrossRef CAS PubMed.
- L. Li and M. Schuster, Influence of phosphate and solution pH on the mobility of ZnO nanoparticles in saturated sand, Sci. Total Environ., 2014, 472, 971–978 CrossRef CAS PubMed.
- I. Jośko, P. Oleszczuk and E. Skwarek, The bioavailability and toxicity of ZnO and Ni nanoparticles and their bulk counterparts in different sediments, J. Soils Sediments, 2016, 16, 1798–1808 CrossRef.
- F. Wang, R. R. Goulet and P. M. Chapman, Testing sediment biological effects with the freshwater amphipod Hyalella azteca: the gap between laboratory and nature, Chemosphere, 2004, 57, 1713–1724 CrossRef CAS PubMed.
- M. N. Croteau, A. D. Dybowska, S. N. Luoma and E. Valsami-Jones, A novel approach reveals that zinc oxide nanoparticles are bioavailable and toxic after dietary exposures, Nanotoxicology, 2011, 5, 79–90 CrossRef CAS PubMed.
- K. L. Goodyear and S. McNeill, Bioaccumulation of heavy metals by aquatic macro-invertebrates of different feeding guilds: a review, Sci. Total Environ., 1999, 229, 1–19 CrossRef CAS.
- X. Jiang, X. Wang, M. Tong and H. Kim, Initial transport and retention behaviors of ZnO nanoparticles in quartz sand porous media coated with Escherichia coli biofilm, Environ. Pollut., 2013, 174, 38–49 CrossRef CAS PubMed.
- M. Heinlaan, A. Ivask, I. Blinova, H.-C. Dubourguier and A. Kahru, Toxicity of nanosized and bulk ZnO, CuO and TiO2 to bacteria Vibrio fischeri and crustaceans Daphnia magna and Thamnocephalus platyurus, Chemosphere, 2008, 71, 1308–1316 CrossRef CAS PubMed.
- I. Blinova, A. Ivask, M. Heinlaan, M. Mortimer and A. Kahru, Ecotoxicity of nanoparticles of CuO and ZnO in natural water, Environ. Pollut., 2010, 158, 41–47 CrossRef CAS.
- S. Lopes, F. Ribeiro, J. Wojnarowicz, W. Łojkowski, K. Jurkschat, A. Crossley, A. M. V. M. Soares and S. Loureiro, Zinc oxide nanoparticles toxicity to Daphnia magna: size-dependent effects and dissolution, Environ. Toxicol. Chem., 2014, 33, 190–198 CrossRef CAS PubMed.
- E. J. Park, J. Yi, Y. Kim, K. Choi and K. Park, Silver nanoparticles induce cytotoxicity by a Trojan-horse type mechanism, Toxicol. In Vitro, 2010, 24, 872–878 CrossRef CAS PubMed.
Footnotes |
† Electronic supplementary information (ESI) available. See DOI: 10.1039/c8en00755a |
‡ Current affiliation: State Key Laboratory of Crop Stress Biology in Arid Areas, College of Life Sciences, Northwestern A&F University, Yangling, Shaanxi 712100, P.R. China. |
|
This journal is © The Royal Society of Chemistry 2019 |