Bacillus subtilis causes dissolution of ceria nanoparticles at the nano–bio interface†
Received
11th September 2018
, Accepted 16th November 2018
First published on 16th November 2018
Abstract
The cytotoxicity of ceria nanoparticles (NPs) against microorganisms was identified as a particle-specific toxicity in previous work since no release of Ce3+ ions was evident. Although the literature has demonstrated a reduction of ceria NPs after their interaction with bacteria, it remains unknown whether the reduction is followed by a release of Ce3+ ions from the particle surface. The present work aims to study whether there is dissolution of ceria NPs at the nano–bio interface and evaluate the possible ionic toxicity against bacteria. Our results suggest that rod-like ceria NPs could be reduced by Bacillus subtilis under planktonic conditions, then the Ce3+ ions adjacent to the surface oxygen vacancies would be chelated by the adsorption sites on the bacterial cell wall and extracted from the particles. The biosorption of the dissolved Ce3+ ions by bacteria is further confirmed by the Ce3+ desorption after the protonation of carboxyl groups on the cell wall. Therefore, we demonstrate for the first time the dissolution of ceria NPs at the bacterial surface as a result of the nano–bio interaction. These findings together reveal a combined mechanism for the toxicity of ceria NPs: particle-related delivery of Ce to the bacterial surface and ion-related toxicity following the dissolution. This insight would benefit our understanding of the mechanism underlying the cytotoxicity of ceria NPs.
Environmental significance
Cerium oxide nanoparticles (ceria NPs) are widely used in fuel additives, green industry, and biomedical applications, however, detailed knowledge on the biological mechanisms underlying their environmental impacts is often lacking. Although the literature has shown the reduction of ceria NPs after their interaction with bacteria, it remains unknown whether the reduction is followed by a release of Ce3+ ions from the particle surface. This work demonstrates that ceria NPs could be reduced after their interaction with bacteria, and then the formed Ce3+ ions on the particle surface could be chelated by the adsorption sites on the bacterial cell wall and extracted from the particles. The dissolution of ceria NPs is further confirmed by a significant desorption of Ce3+ from the bacterial cell wall after the pH-dependent protonation of carboxyl groups. These results would lead to a better understanding of the toxicity of ceria NPs.
|
1. Introduction
With the rapid development of nanoscience and nanotechnology, manufactured nanoparticles (NPs) are being increasingly produced and used, and consequently being gradually released into the environment. Ceria NPs are well known as high performance catalysts, due to the presence of mixed valence states of Ce(III) and Ce(IV), and the presence of oxygen vacancies.1 They are widely used in fuel additives, the green industry, and biomedical applications, and the estimated global production of ceria NPs ranges from 55 to 10
000 tons per year based on different statistical scales.2 Therefore, the Organization for Economic Co-operation and Development (OECD) has identified ceria NPs as one of the 13 priority listed representative manufactured nanomaterials for immediate testing.3,4
The toxicities of NPs against bacteria have attracted great attention since bacteria perform many critical roles in ecosystem function and productivity. It has been documented that exposure to ceria NPs could lead to strain-, size-, coating- and medium-dependent inhibition of bacteria.5–8 In previous work, ceria NPs were considered to be highly insoluble under environmental conditions, and negligible release of Ce3+ could be detected.9,10 Studies based on synchrotron radiation X-ray absorption spectroscopy and electron energy loss spectroscopy have demonstrated the reduction of ceria NPs in bio-tissues,11–14 however, it remains unknown whether Ce reduction would trigger the dissolution of ceria NPs. Actually, the detected Ce(III) was attributed to the reduction-induced Ce3+ lattice defects in ceria NPs,15 rather than to the release of Ce3+ ions from the particles. Therefore, the contribution from the ionic toxicity of Ce3+ ions has not been seriously considered in the toxicological studies of ceria NPs against bacteria.
Our previous work also found a reduction in ceria NPs at the root surface of plants.13,16–19 By using STXM, a method with high spatial and chemical resolutions, we demonstrated a deposition of Ce in the intercellular spaces and vacuoles of cucumber roots, with Ce speciation exclusively in the trivalent state.13,17 These findings suggest a dissolution of ceria NPs following the reduction. Therefore, this study tries to identify a possible dissolution of ceria NPs after their interaction with bacteria and evaluate the consequent toxicity caused by the dissolved Ce3+. Bacillus subtilis (B. subtilis), a Gram-positive bacterium, was exposed to rod-like ceria NPs (rod-ceria) under planktonic conditions in Luria-Bertani (LB) medium, and the speciation of Ce in bacterial samples was analyzed by synchrotron radiation X-ray absorption near edge structure (XANES) spectroscopy. Rod-ceria were chosen because they are much easier to reduce than their octahedral or cubic counterparts.20 Factors that control the dissolution of ceria NPs were carefully studied, and a new separation method was adopted to collect the dissolved Ce3+ ions adsorbed on the bacterial surface. The results would lead to a better understanding of the cytotoxicity of ceria NPs against bacteria.
2. Materials and methods
2.1. Synthesis and characterization of rod-ceria
Rod-ceria were synthesized by a hydrothermal method.21 The process of synthesis and characterization is described in detail in the ESI.†
2.2. Medium and bacterium
LB medium: 10 g L−1 peptone, 5 g L−1 yeast extract, 5 g L−1 NaCl, 1 L DI water (pH = 7.1); 2-concentrated NaCl-free LB medium: 20 g L−1 peptone, 10 g L−1 yeast extract, 1 L DI water. LB medium can be prepared by mixing 1% NaCl solution with an aliquot of 2-concentrated NaCl-free LB medium.
B. subtilis GIM1.784 (obtained from the Microbial Culture Collection Center of Guangdong, GIMCC) was maintained on LB plates. A single colony of B. subtilis was picked and grown in 100 mL of sterilized LB medium. Then the strain was incubated in a rotary shaker (220 rpm) for 10 h at 37 °C. The cells were harvested by centrifugation for 10 min at 4000g and washed three times with 1% NaCl solution.
2.3. Factors that determine the dissolution of rod-ceria
Rod-ceria (100 μg mL−1) were incubated with HCl (0.01 M), ascorbic acid (Vc, 1 mM), or sodium citrate (Cit, 1 mM) in LB medium (adjusted to pH = 7.1, except for the HCl treatment) for 3 h, respectively. The suspensions were then centrifuged at 13
000 × g for 20 min twice (with a 3 min interval) to collect the supernatants, and the concentrations of Ce3+ in the supernatants were determined with Arsenazo III.22 A mixture of 100 μg mL−1 rod-ceria and 40 μg mL−1 Ce3+ (to make the comparison easier, the concentration of Ce3+ ions was calculated as CeO2; the same hereinafter) was used as a control for centrifugation and spectrophotometric determination.
2.4. Ce speciation by XANES analysis
XANES analysis followed by linear combination fitting (LCF) was used to quantify the Ce3+ fraction in the rod-ceria reduced by Vc. Rod-ceria (100 μg mL−1) were incubated with 1 mM Vc for 3 h in the LB medium with or without B. subtilis (1 × 108 cells per mL), then the cultures were freeze-dried, motor-homogenized, and pressed into circular slices with a diameter of 10 mm and a thickness of 1 mm. Fluorescence mode was applied for the collection of Ce LIII-edge XANES spectra of the samples and reference standards. Athena software was used to perform LCF analysis of XANES spectra,23 and the reliability of Ce speciation has been evaluated previously.18
2.5. Desorption of Ce3+ ions from the bacterial surface
To prove the extraction and biosorption of Ce3+ ions by B. subtilis, we tried to make the Ce3+ ions partially desorbed from the bacterial surface using a method reported by Bonificio et al.24 Briefly, the residual particles, bacteria and the Ce3+ ions adsorbed by the former two were collected via centrifugation, and then the pellet was resuspended in 0.9% NaCl solution (NS) with a pH of 2.5 (adjusted with HCl). The low pH could lead to the desorption of Ce3+ from the bacterial cell wall, then the suspension was centrifuged again, and the concentration of Ce3+ ions in the supernatant was determined with Arsenazo III or ICP-MS.
2.6. Dissolution of rod-ceria caused by the interaction with B. subtilis
The B. subtilis suspension was diluted to ∼103 CFU mL−1 in fresh LB medium and then incubated with 100 μg mL−1 rod-ceria under continuous shaking at 220 rpm at 37 °C. After 48 h, the culture was divided into 2 aliquots, and one was collected for XANES analysis. Another aliquot was used to study the desorption of Ce3+ ions from B. subtilis after the treatment of bacteria in NS pH 2.5. As a comparison, the speciation of 100 μg mL−1 rod-ceria incubated in the bacteria-free LB medium was also studied. The B. subtilis incubated with 100 μg mL−1 rod-ceria were also collected for TEM and SEM observation.
2.7. Cytotoxicity of rod-ceria and Ce3+ ions
Rod-ceria or Ce3+ ions were added into the B. subtilis cultures (1 × 108 cells per mL) in LB medium to reach final concentrations of 1, 10, 20, and 100 μg mL−1. The cultures were incubated for 6 h under continuous shaking at 220 rpm at 37 °C, and then the bacterial viabilities were assessed by the MTT (3-(4,5-dimethylthiazol-2-yl)-2,5-diphenyltetrazolium bromide) assay method.25 Our preliminary test showed that the presence of ceria NPs or Ce3+ would not interfere with the MTT assay (ESI,† Table S1).
In a parallel study, rod-ceria or Ce3+ ions were mixed with 2 × 108 CFU mL−1B. subtilis in 1% NaCl solution and incubated for 5 min. Then, each culture was mixed with an aliquot of 2-concentrated NaCl-free LB medium, making the composition of the culture medium exactly the same as that of the LB medium. The final concentrations of rod-ceria were 1, 10, 20, and 100 μg mL−1; the final concentrations in the Ce3+-treated groups were 0.01, 0.05, 0.1, 0.5, 1, 10, and 100 μg mL−1, respectively. The mixtures were incubated for another 6 h, and then the bacterial viabilities were assessed by MTT assay. The desorption of Ce3+ ions from B. subtilis was also studied after the treatment of bacteria in NS pH 2.5.
2.8. Data analysis
Results were expressed as the mean ± SD (standard deviation). Statistical evaluation was conducted using Statistical Packages for the Social Sciences v15 (SPSS Inc., Chicago, USA). One-way analysis of variance (ANOVA) and Tukey's-test was carried out to illustrate the significant difference between the treatments, and a difference with p < 0.05 was considered statistically significant.
3. Results and discussion
3.1. Characterization of rod-ceria
The synthesized rod-ceria have a fluorite cubic structure, with a diameter of 8.5 ± 1.5 nm and a length of 168 ± 18 nm (Fig. 1). The enclosed planes are {111}, which is confirmed by the average lattice distance (0.31 nm).26 XANES analysis and subsequent spectrum deconvolution by LCF was used to obtain the average Ce valence of both the superficial layer and the bulk of rod-ceria. The result shows that the proportion of Ce3+ in the synthesized rod-ceria is not detectable. The detailed physiochemical properties are shown in the ESI† (Fig. S1).
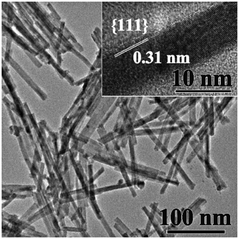 |
| Fig. 1 A TEM micrograph of rod-ceria. | |
3.2. Simulated study on the factors determining the dissolution of rod-ceria
Our work previously demonstrated that ceria NPs could undergo a reduction with the assistance of Vc.13 The reduction of ceria NPs is associated with the formation of oxygen vacancies, which would result in the valence change (Ce4+ → Ce3+) of two cations per vacancy (Fig. 2).27 The formed oxygen vacancies or vacancy clusters expose exclusively Ce3+,28 providing effective coordination sites to carboxyl or phosphate groups. Ce reduction is considered as the first step toward the dissolution of ceria NPs, as Ce3+ is more soluble than Ce4+ under neutral conditions.29 In the absence of Vc, neither the incubation with HCl nor Cit had an individual contribution to the dissolution of rod-ceria in a short time (Fig. 3, gray and purple columns, with dissolution percentages of ∼0.3%).
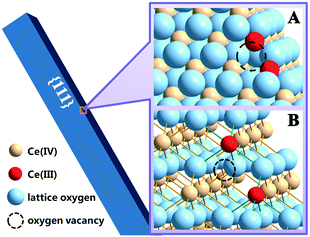 |
| Fig. 2 Schematic representation of the oxygen vacancy on the surface of rod-ceria. Two Ce ions adjacent to the oxygen vacancy site are reduced to Ce3+. A: Space-filling model of the rod-ceria {111} surface and oxygen vacancy; B: ball-and-stick model of the rod-ceria. | |
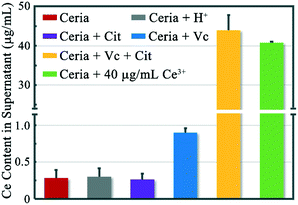 |
| Fig. 3 The dissolution of 100 μg mL−1 rod-ceria after a 3 h incubation with HCl, Vc or Cit in LB medium. The concentrations of Ce in the supernatants of mixtures were determined with Arsenazo III after centrifugation. H+: 0.01 M HCl; Vc: 1 mM ascorbic acid; Cit: 1 mM sodium citrate. A mixture of 100 μg mL−1 rod-ceria and 40 μg mL−1 Ce3+ in LB medium was used as a quality control for centrifugal separation and spectrophotometric measurements. Data are expressed as the mean ± SD (n = 3). | |
Our previous work speculated that carboxyl groups could chelate the surface Ce3+, therefore enhancing the dissolution of ceria NPs after reduction.13 Furthermore, this study reveals that the dissolution-promoting effects of different carboxyl groups vary greatly. There were abundant carboxyl groups in LB medium (mainly amino acids and peptides), but rod-ceria released only a small amount of Ce3+ ions (0.9% of the total Ce) into the supernatant after a 3 h incubation with Vc in LB medium (Fig. 3, blue column). Similarly, oleic acid could hardly extract Ce3+ ions from the particles, as oleic acid was used to stabilize the Ce3+ ions on the surface of ceria NPs.30 Oleic acid and most natural amino acids are monodentate O-donor ligands. The complexes of lanthanides with monodentate ligands are considered unstable and tend to dissociate in aqueous solution.31 These results suggest that the Ce3+ ions could stay stable in the crystal lattice of the reduced ceria NPs when there are no strong complexing ligands for Ce3+ in the surroundings. But if Cit, a multidentate ligand for Ce3+, was mixed with the reduced rod-ceria, 44% of the total cerium was extracted from the particle surface and released into the culture medium (Fig. 3, yellow column). This implies that the chelating interaction between Cit and surface Ce3+ is very strong, so that the bonds of Ce3+ and lattice oxygen (colored green in Fig. 2B) could be irreversibly compromised. These findings together suggest that a simultaneous interaction from the reductant and multidentate chelant is a prerequisite to greatly improve the dissolution rate of ceria NPs.
3.3. Chelation of Ce3+ ions by B. subtilis
The bacterial cell wall has abundant binding sites for heavy metals. It has been suggested that carboxylate and phosphate sites are mainly responsible for the adsorption of lanthanides onto bacterial cells.32,33 A literature survey suggests that the biosorption of lanthanides by bacteria occurs through multidentate coordination at the carboxylate sites.34,35 The phosphate sites have an even greater affinity to lanthanides,36 and an EXAFS study revealed that the lanthanides are primarily bound to the phosphate sites and subsequently to the carboxylate sites on the bacterial cell surface.37
In this work, we tried to investigate whether the adsorption sites on the cell wall of B. subtilis could play a similar enhancing role of Cit in the dissolution of reduced rod-ceria. Rod-ceria were incubated with Vc in LB medium with or without B. subtilis for 3 h, and then the cultures were collected via freeze drying for speciation analysis by XANES. The Ce(III) fraction detected by XANES analysis is composed of two types of Ce3+ ions: the Ce3+ ions released from the particle surface and the lattice Ce3+ ions still bonded in the reduced ceria NPs. When incubated with Vc, the LCF data reveal a reduction of 3.4% of total Ce to the trivalent state in the LB medium without B. subtilis (Fig. 4). Since Fig. 3 shows that about 0.9% of total Ce was released into the culture medium, 2.5% of Ce remained together with rod-ceria. It seems like Vc could only reduce part of the Ce localized at the superficial layer of rod-ceria, as there was not enough chelant to extract Ce3+ from the particle surface and allow the further reduction of Ce in the deeper layers. When B. subtilis was introduced into the culture of rod-ceria and Vc, the fraction of Ce(III) was greatly increased to 10.4%, nearly 3-fold that in the absence of B. subtilis. It was shown that ceria NPs could be reduced after they come into contact with the surface of bacteria,12,38 but negligible Ce(III) was detected after a 3 h incubation of rod-ceria with B. subtilis in this study, suggesting the reduction of rod-ceria by B. subtilis was inefficient or time-consuming (Fig. 4). Since the bacteria-caused Ce reduction was very limited, the increased fraction of Ce(III) should probably be attributed to the enhanced release of Ce3+ from rod-ceria. We speculate that the adsorption sites for Ce3+ on the bacteria cell wall may act like Cit, extracting Ce3+ from the surface of reduced rod-ceria, thereby exposing more Ce in the deeper layers for further reduction. Thus, more Ce in rod-ceria were reduced to Ce3+ by Vc and then extracted from the NPs by B. subtilis.
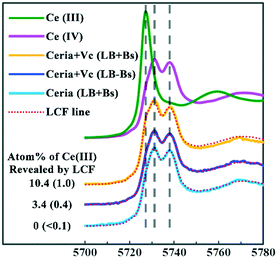 |
| Fig. 4 Ce LIII-edge XANES spectra. XANES spectra of reference standards: bulk CeO2 and CePO4; XANES spectra of samples: 100 μg mL−1 rod-ceria incubated with 1 mM Vc in LB medium with or without B. subtilis (marked as +Bs or −Bs, respectively) for 3 h, and 100 μg mL−1 rod-ceria incubated with B. subtilis in LB medium for 3 h; the LCF result is expressed as the atomic percent of Ce(III) in ceria, followed by the fitting uncertainty in parentheses. | |
The XANES results provide supportive, but no definitive, evidence for the dissolution of the reduced ceria NPs on the bacterial surface. To further verify the dissolution of rod-ceria, we tried to directly identify the release of Ce3+ ions from NPs. Since both the dissolved Ce3+ ions and ceria NPs could be adsorbed on the bacterial surface (ESI,† Fig. S2), it is impossible to distinguish the dissolved Ce3+ ions and the lattice Ce3+ ions from each other by XANES analysis or ICP-MS analysis alone. Recently, Bonificio et al. reported a method to trigger the desorption of lanthanides from the bacterial surface as a function of pH.24 By adjusting the pH to 2.5, the carboxyl groups on the bacterial cell wall are protonated, therefore their affinity to lanthanides will be weakened and the adsorbed lanthanides will be partially released into the culture medium. In this study, 100 μg mL−1 rod-ceria and 1 mM Vc were incubated with B. subtilis in LB medium for 3 h, then the supernatant of the culture was removed. According to our XANES analysis, about 10.4 μg mL−1 Ce was reduced to Ce3+ during the incubation, and 0.8 μg mL−1 Ce3+ was removed within the supernatant (Fig. 5). After the protonation of the carboxyl groups on the bacterial surface, the pellet (the Vc-treated NPs and B. subtilis) released 3.97 μg mL−1 Ce in the 0.9% NaCl solution containing HCl (NS, pH 2.5), while in the absence of B. subtilis, the Vc-treated rod-ceria released only 0.74 μg mL−1 Ce (Fig. 5). At low pH, the phosphate groups on the cell wall could still contribute to the biosorption of Ce3+, therefore there were still some Ce3+ ions adsorbed on the surface of B. subtilis. These results provide a solid support for the chelating role of B. subtilis to Ce3+ ions after the reduction of rod-ceria, thereby demonstrating the dissolution of reduced ceria NPs on the bacterial surface.
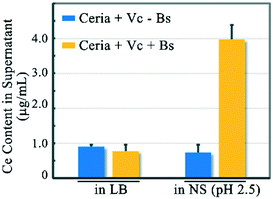 |
| Fig. 5 The concentration of Ce3+ ions in the supernatant of culture after a 3 h incubation of rod-ceria with Vc in LB medium with or without B. subtilis. The pellet was resuspended in 0.9% NCl solution (NS, pH 2.5) for 10 min, and then the concentration of Ce3+ ions in the supernatant was determined after centrifugation. Data are expressed as the mean ± SD (n = 3). | |
3.4. The dissolution of rod-ceria on the surface of B. subtilis
We have proved that the adsorption sites on the B. subtilis cell wall would chelate and extract surface Ce3+ ions from ceria NPs after the reduction. In the above studies, rod-ceria were reduced with the assistance of Vc. Thill et al. found that bacteria could also cause the reduction of ceria NPs at the nano–bio interface where they directly come into contact with each other.12 That means bacteria alone could induce not only the reduction of ceria NPs but also the dissolution of the once-believed-to-be-insoluble NPs.
In the following study, we tried to characterize the dissolution of rod-ceria after the nano–bio interaction. B. subtilis was incubated with 100 μg mL−1 rod-ceria for 6 h in LB medium, then the residual particles and bacteria were collected by centrifugation, and the desorption of Ce3+ ions from bacteria in NS (pH 2.5) was determined with ICP-MS. LCF combined with XANES analysis failed to detect the total Ce3+ fraction in the whole culture, because the reduction of rod-ceria by B. subtilis was inefficient and the LCF method is not sensitive and accurate enough to quantify a slight valence change.39 The ICP-MS result suggests that 0.49 μg mL−1 Ce3+ was desorbed from the bacterial surface after the protonation of carboxyl groups (Fig. 6). When rod-ceria were incubated with B. subtilis for 48 h, LCF analysis showed that 10.3% of the total Ce was reduced to Ce3+ (Fig. 6 and S3 in ESI†) and a desorption of 3.32 μg mL−1 Ce3+ from the bacterial surface (in NS, pH 2.5) was determined by ICP-MS. Therefore, these results demonstrate that B. subtilis could cause the dissolution of rod-ceria at the nano–bio interface.
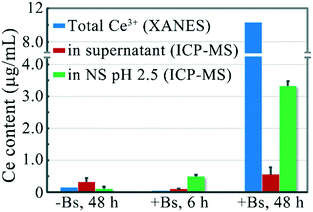 |
| Fig. 6 The content of Ce3+ ions determined by XANES and the desorption of Ce3+ from B. subtilis determined by ICP-MS. Rod-ceria were incubated in the LB medium with or without B. subtilis for 6 or 48 h, and the total Ce3+ fraction in the culture was determined with XANES analysis. The concentration of Ce3+ ions in the supernatant of culture was determined with ICP-MS after centrifugation. The pellet was resuspended in 0.9% NaCl solution (NS, pH 2.5) for 10 min, and the desorption of Ce3+ ions from the bacterial surface was determined with ICP-MS. The ICP-MS results are expressed as the mean ± SD (n = 3). | |
3.5. Contribution of Ce3+ ions to the toxicity of rod-ceria
The cytotoxicity of ceria NPs against bacteria is generally found in some low-nutrient solutions rather than in LB medium, as the bio-molecules in LB medium might disturb the nano–bio interaction.8,12 In this study, 100 μg mL−1 rod-ceria could suppress the growth of B. subtilis by 23.5% even in LB medium, while Ce3+ exposure in LB medium showed no cytotoxicity (ESI,† Fig. S4). At a superficial glance, these results seem to rule out the contribution of Ce3+ ions to the toxicity of rod-ceria against B. subtilis. However, further investigation just reveals that the ionic toxicity caused by ceria dissolution could not be properly simulated by the incubation of bacteria with Ce3+ ions in LB medium. When Ce3+ ions were added into the culture of B. subtilis in LB medium, less than 5% of the Ce3+ ions could be adsorbed by the bacteria after a 6 h incubation (data not shown). This is because Ce3+ would coordinate with the abundant O-donor ligands in LB medium immediately after they were added into the culture.31 Although the formed complexes may not be stable enough, the biosorption of Ce3+ ions by B. subtilis would be greatly suppressed due to the ubiquity of amino acids and peptides in the surroundings as well as the steric hindrance caused by the complexation. By contrast, when Ce3+ ions were introduced into the culture by the dissolution of rod-ceria on the bacterial surface, most of the ions were chelated by B. subtilis, releasing only 0.098 μg mL−1 Ce3+ into the medium after a 6 h incubation (Fig. 6). Therefore, the ionic toxicity of Ce3+ following the dissolution of rod-ceria at the bacterial surface will be greatly underestimated by incorrect simulation results.
To make sure there were enough Ce3+ ions adsorbed on the bacterial surface, B. subtilis was incubated with Ce3+ or rod-ceria at a series of concentrations in 1% NaCl solution for 5 min. Then each culture was mixed with an equal volume of 2-concentrated NaCl-free LB medium, to make the composition of the culture medium exactly the same as that of the LB medium. After a 6 h incubation, the viability of B. subtilis was determined by MTT assay. Through the modification of the test protocol, the inhibition of bacterial viability caused by 100 μg mL−1 rod-ceria was not significantly changed (25.5 ± 5.7% versus 23.5 ± 9.7%), while the ion-related toxicity in LB medium was greatly exacerbated (Fig. 7). When adsorbed by the cell wall of B. subtilis, 0.1–1 μg mL−1 Ce3+ ions could reduce the bacterial viability as much as 100 μg mL−1 rod-ceria did. It should be noted that the modified protocol can't exactly simulate the ionic toxicity attributed to the dissolution of rod-ceria either, as almost all of the Ce3+ ions were adsorbed by the bacteria at the very beginning of the exposure, while during the incubation of rod-ceria and B. subtilis, Ce3+ ions were gradually dissolved and adsorbed to the bacterial surface. Nevertheless, this work proves that the ionic toxicity of Ce3+ ions could at least partially account for the toxicity of rod-ceria against bacteria.
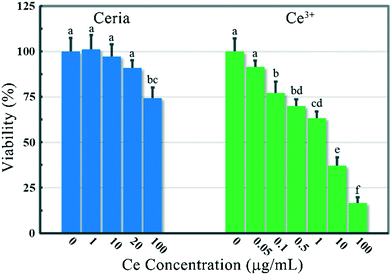 |
| Fig. 7 The viability of B. subtilis after a 5 min incubation with rod-ceria or Ce3+ ions in 1% NaCl followed by a 6 h incubation in LB medium. The values are expressed as the mean ± SD (n = 6). Columns labelled with different letters are significantly different at p < 0.05 using Tukey's t-test. | |
The potential contribution from the nanoproperties of rod-ceria to their toxicity has also been evaluated in the present study. Previous work supposed that the Ce4+ ions of ceria NPs could undergo a reduction on the surface of bacteria, at the expense of the oxidation of the bacterial membrane.12,38 Another hypothesis was that the surface Ce3+ ions in ceria NPs could act as hot spots for the catalysis of ROS generation, therefore imposing oxidative stress on cells.40 But the oxidative-damage hypothesis is not supported by our findings, as no lipid peroxidation was found in B. subtilis after a 6 h exposure (ESI,† Fig. S5).
A “Trojan horse” type effect has been proposed to explain the cytotoxicity of some easily ionized NPs: NPs could bypass the cellular defense system against toxic ions (in animal cells, mainly the cell membrane) and release high levels of toxic ions in cells after their internalization.41 Unlike the cell experiment, in this work the culture medium acts as a major defense against Ce3+ ions. The solvents of the culture medium could detoxify the metal ions dissolved in the medium by decreasing their bioavailability.42 When compared with the direct addition of Ce3+ ions into LB medium, the introduction of rod-ceria results in a more efficient contact between Ce3+ ions and the bacterial cell wall and avoids the inactivation of Ce3+ ions in the culture medium. Thus, rod-ceria exert toxicity to bacteria via an unconventional “Trojan horse” mechanism, which could be regarded as a combination of the particle-specific delivery of Ce to the bacterial surface and the ionic toxicity following the dissolution.
4. Conclusion
In summary, we have demonstrated that B. subtilis could cause a reduction of rod-ceria at the nano–bio interface, and the generated surface Ce3+ ions would be chelated by the adsorption sites of the bacterial cell wall and extracted from the particles. The dissolution of ceria NPs at the nano–bio interface as well as the consequent biosorption of Ce3+ ions on the bacterial cell wall is further confirmed by an obvious Ce3+ desorption from bacteria following the protonation of carboxyl groups on the cell wall. As a result, rod-ceria efficiently deliver Ce3+ ions to the bacterial cell wall via a “Trojan Horse” mechanism, causing ionic toxicity against B. subtilis. These results would lead to a better understanding of the toxicity of ceria NPs.
Conflicts of interest
There are no conflicts to declare.
Acknowledgements
This work was financially supported by the Ministry of Science and Technology of China (Grant No. 2016YFA0201600), the National Natural Science Foundation of China (11675190, 11575208 and 11375009), the Youth Innovation Promotion Association, CAS (2012006), and the Key Laboratory of Biomedical Effects of Nanomaterials and Nanosafety, CAS (No: NSKF201705).
Notes and references
- Y. Li, X. He, J. J. Yin, Y. Ma, P. Zhang, J. Li, Y. Ding, J. Zhang, Y. Zhao, Z. Chai and Z. Zhang, Acquired superoxide-scavenging ability of ceria nanoparticles, Angew. Chem., Int. Ed., 2015, 54(6), 1832–1835 CrossRef CAS PubMed.
- D. Speed, P. Westerhoff, R. Sierra-Alvarez, R. Draper, P. Pantano, S. Aravamudhan, K. L. Chen, K. Hristovski, P. Herckes and X. Bi, Physical, chemical, and in vitro toxicological characterization of nanoparticles in chemical mechanical planarization suspensions used in the semiconductor industry: Towards environmental health and safety assessments, Environ. Sci.: Nano, 2015, 2(3), 227–244 RSC.
- P. Demokritou, S. Gass, G. Pyrgiotakis, J. M. Cohen, W. Goldsmith, W. McKinney, D. Frazer, J. Ma, D. Schwegler-Berry and J. Brain, An in vivo and in vitro toxicological characterisation of realistic nanoscale ceo2 inhalation exposures, Nanotoxicology, 2013, 7(8), 1338–1350 CrossRef CAS PubMed.
- S. Majumdar, J. Trujillo-Reyes, J. A. Hernandez-Viezcas, J. C. White, J. R. Peralta-Videa and J. L. Gardea-Torresdey, Cerium biomagnification in a terrestrial food chain: Influence of particle size and growth stage, Environ. Sci. Technol., 2016, 50(13), 6782–6792 CrossRef CAS PubMed.
- D. A. Pelletier, A. K. Suresh, G. A. Holton, C. K. McKeown, W. Wang, B. Gu, N. P. Mortensen, D. P. Allison, D. C. Joy and M. R. Allison, Effects of engineered cerium oxide nanoparticles on bacterial growth and viability, Appl. Environ. Microbiol., 2010, 76(24), 7981–7989 CrossRef CAS PubMed.
- Q. Wang, J. M. Perez and T. J. Webster, Inhibited growth of pseudomonas aeruginosa by dextran-and polyacrylic acid-coated ceria nanoparticles, Int. J. Nanomed., 2013, 8, 3395–3399 Search PubMed.
- Y. Kuang, X. He, Z. Zhang, Y. Li, H. Zhang, Y. Ma, Z. Wu and Z. Chai, Comparison study on the antibacterial activity of nano-or bulk-cerium oxide, J. Nanosci. Nanotechnol., 2011, 11(5), 4103–4108 CrossRef CAS PubMed.
- X. He, Y. Kuang, Y. Li, H. Zhang, Y. Ma, W. Bai, Z. Zhang, Z. Wu, Y. Zhao and Z. Chai, Changing exposure media can reverse the cytotoxicity of ceria nanoparticles for escherichia coli, Nanotoxicology, 2012, 6(3), 233–240 CrossRef CAS PubMed.
- X. He, H. Zhang, Y. Ma, W. Bai, Z. Zhang, K. Lu, Y. Ding, Y. Zhao and Z. Chai, Lung deposition and extrapulmonary translocation of nano-ceria after intratracheal instillation, Nanotechnology, 2010, 21(28), 285103 CrossRef PubMed.
- S. Bandyopadhyay, J. R. Peralta-Videa, G. Plascencia-Villa, M. José-Yacamán and J. L. Gardea-Torresdey, Comparative toxicity assessment of ceo2 and zno nanoparticles towards sinorhizobium meliloti, a symbiotic alfalfa associated bacterium: Use of advanced microscopic and spectroscopic techniques, J. Hazard. Mater., 2012, 241, 379–386 CrossRef.
- U. M. Graham, M. T. Tseng, J. B. Jasinski, R. A. Yokel, J. M. Unrine, B. H. Davis, A. K. Dozier, S. S. Hardas, R. Sultana and E. A. Grulke, In vivo processing of ceria nanoparticles inside liver: Impact on free-radical scavenging activity and oxidative stress, ChemPlusChem, 2014, 79(8), 1083–1088 CrossRef CAS PubMed.
- A. Thill, O. Zeyons, O. Spalla, F. Chauvat, J. Rose, M. Auffan and A. Flank, Cytotoxicity of ceo2 nanoparticles for escherichia coli. Physico-chemical insight of the cytotoxicity mechanism, Environ. Sci. Technol., 2006, 40(19), 6151–6156 CrossRef CAS.
- P. Zhang, Y. Ma, Z. Zhang, X. He, J. Zhang, Z. Guo, R. Tai, Y. Zhao and Z. Chai, Biotransformation of ceria nanoparticles in cucumber plants, ACS Nano, 2012, 6(11), 9943–9950 CrossRef CAS PubMed.
- J. A. Hernandez-Viezcas, H. Castillo-Michel, J. C. Andrews, M. Cotte, C. Rico, J. R. Peralta-Videa, Y. Ge, J. H. Priester, P. A. Holden and J. L. Gardea-Torresdey, In situ synchrotron x-ray fluorescence mapping and speciation of ceo2 and zno nanoparticles in soil cultivated soybean (glycine max), ACS Nano, 2013, 7(2), 1415–1423 CrossRef CAS PubMed.
- S. Turner, S. Lazar, B. Freitag, R. Egoavil, J. Verbeeck, S. Put, Y. Strauven and G. Van Tendeloo, High resolution mapping of surface reduction in ceria nanoparticles, Nanoscale, 2011, 3(8), 3385–3390 RSC.
- P. Zhang, Y. Ma, Z. Zhang, X. He, Y. Li, J. Zhang, L. Zheng and Y. Zhao, Species-specific toxicity of ceria nanoparticles to lactuca plants, Nanotoxicology, 2015, 9(1), 1–8 CrossRef CAS PubMed.
- Y. Ma, P. Zhang, Z. Zhang, X. He, J. Zhang, Y. Ding, J. Zhang, L. Zheng, Z. Guo and L. Zhang, Where does the transformation of precipitated ceria nanoparticles in hydroponic plants take place?, Environ. Sci. Technol., 2015, 49(17), 10667–10674 CrossRef CAS PubMed.
- Y. Ma, P. Zhang, Z. Zhang, X. He, Y. Li, J. Zhang, L. Zheng, S. Chu, K. Yang and Y. Zhao, Origin of the different phytotoxicity and biotransformation of cerium and lanthanum oxide nanoparticles in cucumber, Nanotoxicology, 2015, 9(2), 262–270 CrossRef CAS PubMed.
- D. Cui, P. Zhang, Y. Ma, X. He, Y. Li, J. Zhang, Y. Zhao and Z. Zhang, Effect of cerium oxide nanoparticles on asparagus lettuce cultured in an agar medium, Environ. Sci.: Nano, 2014, 1(5), 459–465 RSC.
- P. Zhang, C. Xie, Y. Ma, X. He, Z. Zhang, Y. Ding, L. Zheng and J. Zhang, Shape-dependent transformation and translocation of ceria nanoparticles in cucumber plants, Environ. Sci. Technol. Lett., 2017, 4(9), 380–385 CrossRef CAS.
- H. Mai, L. Sun, Y. Zhang, R. Si, W. Feng, H. Zhang, H. Liu and C. Yan, Shape-selective synthesis and oxygen storage behavior of ceria nanopolyhedra, nanorods, and nanocubes, J. Phys. Chem. B, 2005, 109(51), 24380–24385 CrossRef CAS PubMed.
- W. Briner, R. F. Rycek, A. Moellenberndt and K. Dannull, Neurodevelopmental effects of lanthanum in mice, Neurotoxicol. Teratol., 2000, 22(4), 573–581 CrossRef CAS PubMed.
- B. Collin, E. Oostveen, O. Tsyusko and J. M. Unrine, Influence of natural organic matter and surface charge on the toxicity and bioaccumulation of functionalized ceria nanoparticles in caenorhabditis elegans, Environ. Sci. Technol., 2014, 48(2), 1280–1289 CrossRef CAS PubMed.
- W. D. Bonificio and D. R. Clarke, Rare-earth separation using bacteria, Environ. Sci. Technol. Lett., 2016, 3(4), 180–184 CrossRef CAS.
- M. Stevens and S. Olsen, Comparative analysis of using mtt and xtt in colorimetric assays for quantitating bovine neutrophil bactericidal activity, J. Immunol. Methods, 1993, 157(1–2), 225–231 CrossRef CAS.
- M. Wang, F. Wang, J. Ma, M. Li, Z. Zhang, Y. Wang, X. Zhang and J. Xu, Investigations on the crystal plane effect of ceria on gold catalysis in the oxidative dehydrogenation of alcohols and amines in the liquid phase, Chem. Commun., 2014, 50(3), 292–294 RSC.
- F. Esch, S. Fabris, L. Zhou, T. Montini, C. Africh, P. Fornasiero, G. Comelli and R. Rosei, Electron localization determines defect formation on ceria substrates, Science, 2005, 309(5735), 752–755 CrossRef CAS PubMed.
- X. Liu, K. Zhou, L. Wang, B. Wang and Y. Li, Oxygen vacancy clusters promoting reducibility and activity of ceria nanorods, J. Am. Chem. Soc., 2009, 131(9), 3140–3141 CrossRef CAS PubMed.
- F. Schwabe, R. Schulin, P. Rupper, A. Rotzetter, W. Stark and B. Nowack, Dissolution and transformation of cerium oxide nanoparticles in plant growth media, J. Nanopart. Res., 2014, 16(10), 2668 CrossRef.
- J. Zhang, T. Naka, S. Ohara, K. Kaneko, T. Trevethan, A. Shluger and T. Adschiri, Surface ligand assisted valence change in ceria nanocrystals, Phys. Rev. B: Condens. Matter Mater. Phys., 2011, 84(4), 045411 CrossRef.
-
C. H. Evans, Biochemistry of the lanthanides, Plenum press, New york, 1990, vol. 8 Search PubMed.
- Y. Takahashi, X. Châtellier, K. H. Hattori, K. Kato and D. Fortin, Adsorption of rare earth elements onto bacterial cell walls and its implication for ree sorption onto natural microbial mats, Chem. Geol., 2005, 219(1–4), 53–67 CrossRef CAS.
- N. Das and D. Das, Recovery of rare earth metals through biosorption: An overview, J. Rare Earths, 2013, 31(10), 933–943 CrossRef CAS.
- T. Ozaki, Y. Suzuki, T. Nankawa, T. Yoshida, T. Ohnuki, T. Kimura and A. J. Francis, Interactions of rare earth elements with bacteria and organic ligands, J. Alloys Compd., 2006, 408, 1334–1338 CrossRef.
- R. E. Martinez, O. Pourret and Y. Takahashi, Modeling of rare earth element sorption to the gram positive bacillus subtilis bacteria surface, J. Colloid Interface Sci., 2014, 413, 106–111 CrossRef CAS PubMed.
- B. T. Ngwenya, J. F. W. Mosselmans, M. Magennis, K. D. Atkinson, J. Tourney, V. Olive and R. M. Ellam, Macroscopic and spectroscopic analysis of lanthanide adsorption to bacterial cells, Geochim. Cosmochim. Acta, 2009, 73(11), 3134–3147 CrossRef CAS.
- Y. Takahashi, M. Yamamoto, Y. Yamamoto and K. Tanaka, Exafs study on the cause of enrichment of heavy rees on bacterial cell surfaces, Geochim. Cosmochim. Acta, 2010, 74(19), 5443–5462 CrossRef CAS.
- O. Zeyons, A. Thill, F. Chauvat, N. Menguy, C. Cassier-Chauvat, C. Oréar, J. Daraspe, M. Auffan, J. Rose and O. Spalla, Direct and indirect ceo2 nanoparticles toxicity for escherichia coli and synechocystis, Nanotoxicology, 2009, 3(4), 284–295 CrossRef CAS.
- J. Prietzel, A. Botzaki, N. Tyufekchieva, M. Brettholle, J. Thieme and W. Klysubun, Sulfur speciation in soil by s k-edge xanes spectroscopy: Comparison of spectral deconvolution and linear combination fitting, Environ. Sci. Technol., 2011, 45(7), 2878–2886 CrossRef CAS PubMed.
- G. Pulido-Reyes, I. Rodea-Palomares, S. Das, T. S. Sakthivel, F. Leganes, R. Rosal, S. Seal and F. Fernández-Piñas, Untangling the biological effects of cerium oxide nanoparticles: The role of surface valence states, Sci. Rep., 2015, 5, 15613 CrossRef CAS PubMed.
- I. L. Hsiao, Y.-K. Hsieh, C.-F. Wang, I. C. Chen and Y.-J. Huang, Trojan-horse mechanism in the cellular uptake of silver nanoparticles verified by direct intra- and extracellular silver speciation analysis, Environ. Sci. Technol., 2015, 49(6), 3813–3821 CrossRef CAS PubMed.
- R. Li, Z. Ji, C. H. Chang, D. R. Dunphy, X. Cai, H. Meng, H. Zhang, B. Sun, X. Wang, J. Dong, S. Lin, M. Wang, Y.-P. Liao, C. J. Brinker, A. Nel and T. Xia, Surface interactions with compartmentalized cellular phosphates explain rare earth oxide nanoparticle hazard and provide opportunities for safer design, ACS Nano, 2014, 8(2), 1771–1783 CrossRef CAS PubMed.
Footnote |
† Electronic supplementary information (ESI) available. See DOI: 10.1039/c8en01002a |
|
This journal is © The Royal Society of Chemistry 2019 |
Click here to see how this site uses Cookies. View our privacy policy here.