PCN-224/rGO nanocomposite based photoelectrochemical sensor with intrinsic recognition ability for efficient p-arsanilic acid detection†
Received
20th August 2018
, Accepted 14th November 2018
First published on 15th November 2018
Abstract
Considering p-arsanilic acid (p-ASA) as an organoarsenic pollutant, monitoring concentration of p-ASA is significant to maintain environmental security. Photoelectrochemical (PEC) detection is promisingly applied in environmental monitoring for its lower cost and simpler operation, compared to traditional techniques. However, general electrode materials of PEC sensors need to be modified with biological probes due to lack of specific binding sites to analytes, which may lead to complicated sensor fabrication and bio-recognizers inactivation during storage. Herein, a porphyrinic metal–organic framework/reduced graphene oxide (PCN-224/rGO) nanocomposite with simultaneously excellent photoelectric activity and strong binding characteristics was prepared and used to fabricate a PEC sensor for p-ASA detection avoiding the modification of bio-recognizers. Benefiting from the high conductivity of rGO, the separation efficiency of charge carriers was improved in PCN-224/rGO and thereby photocurrent gained an amplification of about 12 times. The PCN-224/rGO nanocomposite had strong affinity to p-ASA via Zr–O–As coordination and π–π stacking. Significantly, the binding of p-ASA could adjust the band position of PCN-224/rGO and facilitate oxygen reduction by releasing more photogenerated electrons, achieving photocurrent enhancement. The developed PEC sensor had a wide detection range, from 10 ng L−1 to 10 mg L−1, with a detection limit of 5.47 ng L−1. Furthermore, the sensor was successfully applied to monitor p-ASA in simulated natural water and swine manure lixivium. This study paved the way for the design of more efficient PEC sensors for environmental monitoring.
Environmental significance
Detection of p-arsanilic acid (p-ASA) is significant for environmental risk assessment as it can be converted into highly toxic inorganic arsenic species. Photoelectrochemical (PEC) sensors show promising application in environmental monitoring for its brief operation and high sensitivity. Herein, we take advantage of the photoelectric activity and strong binding of the PCN-224/rGO nanocomposite to develop a novel PEC sensor for p-ASA detection without the introduction of bio-recognizers. To our knowledge, this is the first study to apply the PEC sensor with intrinsic recognition ability for p-ASA monitoring. The novel PEC sensor exhibited excellent sensitivity and environmental tolerance for p-ASA detection. It is believed that this work will open a window to designing more efficient PEC sensors for pollutant monitoring.
|
1 Introduction
p-Arsanilic acid (p-ASA), an emerging organoarsenic pollutant, can be converted into highly poisonous inorganic arsenic species (such as arsenate and arsenite) by biological or chemical reactions, causing serious arsenic pollution in groundwater and surface water.1,2 Exposure to inorganic arsenic can lead to bladder, skin cancer and cardiovascular disease.3,4 Thus, knowing the exact distribution and concentration of organoarsenic compounds becomes increasingly essential for environmental risk assessment. Several traditional detection techniques, including high performance liquid chromatography (HPLC) coupled to UV detectors and inductively coupled plasma mass spectrometry (ICP-MS) have been already employed for p-ASA detection and achieved high sensitivity.5,6 However, these methods inevitably require expensive and bulky equipment as well as complex sample pretreatment. Thus, developing simple and efficient techniques for p-ASA detection is desired to satisfy environmental practical detection demands.
Photoelectrochemical (PEC) detection has emerged as a promising analytical method for its simple composition, brief sample pretreatment, high sensitivity and low background.7 Therein, inorganic semiconductors are the most commonly used photoelectric materials for fabricating PEC sensors due to their excellent photoelectric conversion efficiency.8 Generally, biological probes, such as proteins, DNAzymes and antibodies, have to be modified on these semiconductors to endow them with specific binding to analytes.9–11 Nevertheless, the photogenerated holes in semiconductors would damage or even destroy biomolecules, and the bio-recognizers may suffer inactivation or degeneration in a complicated environment.12,13 Therefore, improving the specific binding ability of photoelectric materials instead of bio-recognizer modification is pivotal in developing an advanced PEC sensor for p-ASA practical detection.
Currently, metal–organic frameworks (MOFs), constructed from metal clusters and organic linkers,14 have earned enthusiastic research interest for their potential application in photocatalysis,15 pollutant adsorption16,17 and sensing.18 The availability of functional moieties from ligands or metal clusters design makes it possible to provide chemical binding sites.19 For instance, our previous work found that the Zr-based MOF (UIO-67) had strong affinity to p-ASA through Zr–O–As coordination and π–π stacking.20 Furthermore, some organic ligands of MOFs, such as porphyrin, could act as an antenna to absorb light and activate the metal clusters upon illumination, which makes some MOFs possess semiconductor-like characters and have the possibility of being used as photoelectric materials.21 For example, PCN-224, a kind of porphyrinic MOF, consisting of Zr6O4(OH)4 clusters and an organic porphyrin ligand (TCPP), has excellent photovoltaic activity and environmental stability.22 The Zr6 octahedron of PCN-224 only has six edges connected to carboxylates from TCPP. Thus, the connection number is lower than that of UIO-67 (12) and PCN-222 (8), indicating that PCN-224 could expose more Zr–OH groups for p-ASA binding.23,24 Hence, our motivation in this work is to take advantages of the photoelectric activity and strong binding of PCN-224 to develop a novel PEC sensor for p-ASA detection without the participation of biological recognizers.
In this work, PCN-224 was grown in situ on graphene oxide (GO) sheets using a solvothermal method to obtain the PCN-224/rGO (reduced graphene oxide) nanocomposite. RGO could improve photogenerated electron–hole separation and achieve synergetic magnification of the photocurrent signal with PCN-224. The PCN-224/rGO nanocomposite was modified on an indium tin oxide (ITO) electrode to fabricate a turn-on PEC sensor for p-ASA detection. The major aims are devoted to (1) systematically characterizing PEC behavior of the PCN-224/rGO nanocomposite, (2) investigating analytical performances of the fabricated PEC sensor for p-ASA in water and real samples, (3) disclosing chemical interactions between PCN-224/rGO and p-ASA as well as the mechanism of PEC signal increment. This study provides a new thought to develop a PEC sensor based on a MOF-based nanocomposite with photoelectric activity and binding characteristics for p-ASA detection. We expect that it could attract more interest in the design and development of numerous other advanced sensors for environmental pollutant detection.
2 Experimental section
2.1 Chemicals and materials
Zirconium(IV) chloride (ZrCl4) and meso-tetra(4-carboxyphenyl)porphyrin (TCPP) were purchased from J&K Scientific Ltd. (Shanghai, China). Benzoic acid, humic acid, N,N-dimethylformamide (DMF), p-arsanilic acid, and 4-(2-hydroxyethyl)piperazine-1-ethanesulfonic acid (HEPES) were ordered from Aladdin Reagent Ltd. (Shanghai, China). The other reagents were of analytical grade and were utilized without further purification. Ultrapure water (≥18 MΩ, Milli-Q) was used during the experiment. Indium tin oxide (ITO) conductive glass was obtained from Nanbo Corp. (Shenzhen, Guangdong Province, China) and cut into 2.5 cm × 1 cm size.
2.2 Synthesis of PCN-224 and PCN-224/rGO
GO was synthesized by oxidation of natural graphite according to the modified Hummers' method.25 The PCN-224 was synthesized based on a published report.26 Typically, ZrCl4 (300 mg) and benzoic acid (2.8 g) were dissolved in 50 mL DMF with the aid of ultrasonic treatment. Then, 100 mg TCPP was added into the above mixing solution under consecutive ultrasound. Next, the mixture was transferred into a 100 mL Teflon-lined autoclave and heated in an oven at 120 °C for 24 h. The resulting purple powder was collected by centrifugation and washed with DMF and methanol several times, followed by drying at 100 °C under vacuum. To prepare PCN-224/rGO, GO, as the precursor of rGO, was first dispersed in DMF under ultrasonic treatment to obtain a yellow-brown suspension (1.0 mg mL−1). Then, a certain volume of GO suspension, ZrCl4 (300 mg) and benzoic acid (2.8 g) were dissolved in DMF. The volume of the resulting mixed solution was adjusted to 50 mL. The following treatment was the same as that for PCN-224 synthesis (the synthesis procedure is displayed in Fig. 1A). Specifically, various GO ratios of 0.02, 0.04, 0.08, 0.16, and 0.32 were obtained by adding different volumes of GO suspension, then GO was transformed into reduced graphene oxide during the process of thermal reduction. The products were denoted as PCN/rGO-n (n = 0.02, 0.04, 0.08, 0.16, and 0.32). In this work, the GO ratio was calculated based on the theoretical yield of PCN-224, as depicted in the following
GO ratio = GO mass/PCN-224 mass(theoretical yield). |
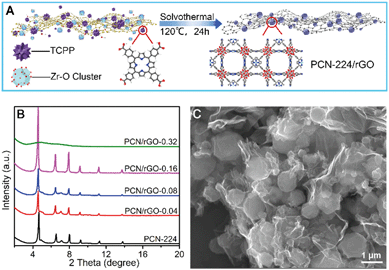 |
| Fig. 1 (A) The design illustration of preparing PCN-224/rGO nanocomposite; (B) XRD patterns of PCN-224 and PCN/rGO-n nanocomposites; (C) SEM image of PCN/rGO-0.16. | |
2.3 Preparation of PCN/rGO-modified ITO electrodes
Before modification, ITO glass electrodes were cleaned using ultrasound in ethanol and water for 30 min, sequentially. After drying under a N2 flow, 5 μL of PCN/rGO suspension (5 mg mL−1) was dropped on the electrode surface and dried at 60 °C for 30 min. The coating area was a circle with a diameter of 0.6 cm, and the residual area of ITO electrodes was insulated by coating with epoxy glue. For comparison, the PCN-224-modified ITO electrode was prepared using the same method.
2.4 Detection of p-ASA in water and real samples
For p-ASA detection in water, PCN/rGO-modified ITO electrodes were incubated in p-ASA samples with different concentrations for 2 h at room temperature to guarantee sufficient reaction between PCN/rGO and p-ASA. After washing with water several times, the resulting electrodes were transferred into the PEC detection cell for photocurrent signal measurement. Detection of p-ASA in real samples with PCN/rGO-modified electrodes was described in section 1 of the ESI.†
2.5 Characterization
The morphologies of the as-prepared materials were characterized by field-emission scanning electron microscopy (FE-SEM; Carl Zeiss MERLIN Compact, Germany). X-ray diffraction (XRD) data of the samples were acquired from an X-ray diffractometer (D8-Advance, Bruker, German) with high-intensity monochromatic nickel-filtered Cu Kα radiation. The tube voltage and current were 40 kV and 40 mA, respectively. XPS spectra were recorded using an Axis Ultra DLD instrument (Kratos Analytical, U.K.) with an Al Kα X-ray source. The spectra were collected at a pass energy of 160 eV for survey scans and 40 eV for high-resolution scans. Fourier transform infrared spectroscopy (FT-IR) spectra of the samples were collected on an RX1 PerkinElmer FT-IR spectrometer employing KBr as the diluter. Photoluminescence (PL) spectra were collected in a quartz cuvette on an Edinburgh Instruments FLS980 spectrophotometer coupled with a time-correlated single-photo-counting system at room temperature.
PEC and electrochemical measurements were conducted with a CHI 660E electrochemical workstation (Shanghai Chenhua Instrument Co., China) using a traditional three-electrode system: the prepared PCN/rGO-modified ITO glass as the working electrode, an Ag/AgCl electrode as the reference electrode and Pt net as the counter electrode. Photocurrent was measured at a bias voltage of −0.1 V with a 500 W Xe lamp as the light source. Electrochemical impedance spectroscopy (EIS) measurements were conducted in the frequency range from 1 Hz to 100 KHz at an open-circuit voltage of 0 V. Mott–Schottky plots were collected in the range from −0.8 V to 0.8 V at a constant frequency of 10 KHz. All PEC and electrochemical measurements were conducted in HEPES buffer (10 mM, pH 7.4) containing 0.3 M KCl solution.
3 Results and discussion
3.1 Characterization of PCN/rGO-n nanocomposites
The design of PCN/rGO-n nanocomposites is shown in Fig. 1A. First, chemical precursors including Zr–O cluster and TCPP were anchored on GO sheets with plenty of oxygen-containing groups. Then the mixture was heated under solvothermal conditions to obtain PCN/rGO-n nanocomposites. The PCN/rGO-n samples were characterized by XRD and SEM. The XRD patterns (Fig. 1B) show that the diffraction peaks of PCN-224 are in accordance with previously reported literature, demonstrating the successful synthesis of PCN-224.26 When the ratio of GO was no more than 0.16, the diffraction patterns of the PCN/rGO-n were similar to that of PCN-224, indicating that the existence of GO did not affect the crystal structure of PCN-224. However, the diffraction peaks of PCN-224 disappeared when the ratio of GO increased to 0.32, suggesting that an excess amount of GO will disrupt crystal growth of PCN-224.
The SEM images of PCN-224 and PCN/rGO-n nanocomposites are displayed in Fig. 1C and S1.† PCN-224 has a spindly needle shape with a width of 400–600 nm and a length of a few micrometers (Fig. S1A†). The introduction of GO changed the surface morphology and crystal size of PCN-224. When the ratio of GO was no more than 0.08, needles and grains coexisted in PCN/rGO-n nanocomposites, and the amount of grains increased with increasing ratio of GO (Fig. S1B and S1C†). When the ratio of GO reached to 0.16, the needles disappeared and the PCN-224 became absolutely granular with a diameter of hundreds of nanometers (Fig. 1C). Moreover, when the ratio of GO increased to 0.32, combining the SEM image (Fig. S1D†) with the above XRD patterns, it can be found that the amorphous particles were covered by rGO nanosheets. The underlying reason may be that the GO nanosheets could act as the nucleation template, and the growth of PCN/rGO-n corresponds to a kinetically regulated template-directed model. At a low amount of GO, the growth rate of PCN-224 crystals is mainly controlled by precursors such as TCPP and the Zr–O cluster, and the addition of GO has little impact on the crystal morphology. While that situation changes substantially at high concentration of GO, and the high density of GO sheets will compete with TCPP for Zr–O cluster coordination, leading to the shift of kinetic equilibrium.27,28 Hence the growth orientation of PCN-224 suffers severe disturbance and an excess of GO even damages the crystal structure of PCN-224. Overall, the PCN-224 particles were successfully grown on ultrathin rGO sheets, forming an intimate interfacial complexion structure. Thus, it is deduced that there may be chemical interactions between PCN-224 and GO.
FT-IR and XPS analyses were conducted to elucidate the chemical interactions between PCN-224 and GO. As depicted in Fig. S4A,† the vibrational peaks of GO matched with its fingerprint groups, such as the C
O stretch of carboxylic species peaking at 1734 cm−1 (curve a).27 The FT-IR spectrum of PCN/rGO (curve c) is similar to that of PCN-224 (curve b), wherein the peaks in the range of 1500–1600 cm−1 are owing to the stretching vibration of C
C in the aromatic ring, and the C
O stretching vibration of –COOH in TCPP shifted to 1715 cm−1.29 The red shift of the C
O stretch from 1734 cm−1 to 1715 cm−1 might be ascribed to π–π stacking and the hydrogen bond between the GO sheets and TCPP ligand.30 Furthermore, as shown in Fig. S4B,† the N 1s XPS spectrum, for PCN-224, was fitted to 2 peaks, including 397.8 eV and 399.9 eV, which were assigned to C–N–C and C
N–C respectively.31 After compounding the GO, a new peak ascribed to H-bonded N at 400.9 eV appeared in the spectra of PCN/rGO, verifying the hydrogen bond between GO sheets and TCPP.32 It is expected that the intimate interfacial contact originating from the chemical interactions between GO and PCN-224 is beneficial to achieving excellent PEC behavior.
The PEC performances of GO, PCN-224 and PCN/rGO are shown in Fig. 2A. It can be seen that the photocurrent of GO was extremely faint and pristine PCN-224 exhibited a weak photocurrent of 0.14 μA upon illumination. After introducing GO into PCN-224, the photocurrent increased remarkably to 1.7 μA, which was about 12 times higher than that of PCN-224. It could be explained that highly conductive rGO facilitated the transfer of electrons from photoexcited PCN-224, and thus the PCN/rGO nanocomposite achieved effective separation of photogenerated electron–hole, obtaining obvious enhancement of PEC behavior.33 Furthermore, according to the EIS results shown in Fig. 2B, the semicircle diameter of the Nyquist plot for PCN/rGO was obviously smaller than that of pure PCN-224, indicating that the electron transfer resistance of PCN-224 was much higher than that of PCN/rGO. The result demonstrated that the conductivity of PCN-224 increased visibly after coupling with rGO. The high conductivity can improve the kinetic charge transfer and PEC behavior.34 In addition, the photoluminescence (PL) spectra (Fig. 2C) show that the PL intensity of PCN/rGO is lower than that of PCN-224, meanwhile the emission lifetime of PCN/rGO (1.07 ns) is shorter than that of PCN-224 (1.45 ns) in terms of time-resolved PL spectroscopy (Fig. 2D). The PL quenching and lifetime decrease suggested the establishment of the electron transfer pathway from PCN-224 to rGO in a nonradiative quenching channel,35 Hence, sufficient interfacial charge migration and suppression of photoinduced electron–hole recombination were achieved in the PCN/rGO nanocomposite.
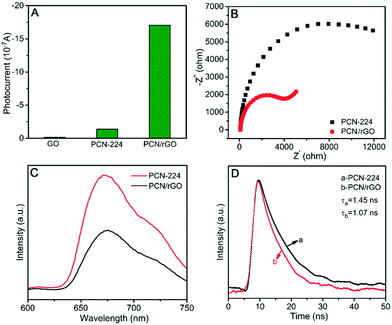 |
| Fig. 2 (A) Photocurrent response of GO, PCN-224 and PCN/rGO; (B) EIS plots of PCN-224 and PCN/rGO; (C) PL emission (λex = 516 nm) spectra of PCN-224 and PCN/rGO in DMF; (D) time-resolved transient PL decay of PCN-224 and PCN/rGO. | |
3.2 Fabrication of the PEC sensor and study of analytical performance
Feasibility of the PEC sensor for p-ASA detection.
Photocurrent response was measured to investigate the feasibility of the developed PEC sensor for p-ASA detection. The home-made PEC measurement system and the detection process of the PEC sensor towards p-ASA are illustrated in Fig. 3A. As seen from Fig. 3B, the photocurrent signal on the sensor electrode increased significantly with the introduction of p-ASA (about 4 times higher than that of the bare PCN/rGO electrode). Furthermore, the binding kinetics of p-ASA on the PCN/rGO electrode was measured by recording the photocurrent–time curve. As shown in Fig. 3C, the photocurrent signal increased over time lasting and tended to level off after 90 min when the sensor electrode was incubated in p-ASA solution, resulting in a smooth binding-curve. The photocurrent–time curve indicated that the photocurrent value was directly proportional to the amounts of p-ASA adsorption on the PCN/rGO electrode, which clearly demonstrated that the PEC sensor could respond to p-ASA in a signal-on mode.
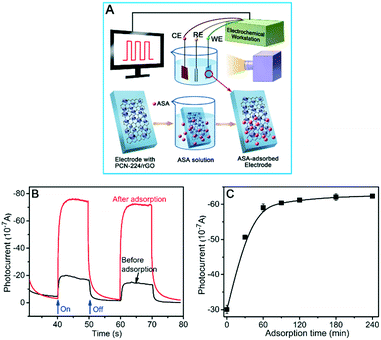 |
| Fig. 3 (A) The scheme of constructing a home-made PEC system and schematic process of adsorbing p-ASA on the PCN/rGO electrode for PEC detection; (B) photocurrent of the PCN/rGO electrode before and after adsorption of p-ASA; (C) temporal evolution of the photocurrent of the PCN/rGO electrode with lasting p-ASA adsorption time. | |
Optimization of experimental conditions.
In order to obtain a sensor with reliable, stable and sensitive PEC signal, the ratio of GO in the PCN/rGO composite needs to be optimized. As displayed in Fig. S6,† the photocurrent value rose with the increase of the GO ratio in the range from 0 to 0.16 and then decreased sharply with further GO ratio increment. The reason might be that moderate amounts of GO could increase the conductivity of the sensor electrode and hinder the recombination of electron–hole. While excessive GO damaged the growth pattern of PCN-224 (Fig. 1B for PCN/rGO-0.32) and exceeding GO covering the surface of PCN-224 (Fig. S1D†) would reduce light absorption of PCN-224. Thus, the PCN/rGO complex with the GO to PCN-224 ratio of 0.16 was employed to construct the PEC sensor electrode for p-ASA detection in this work. In addition, the binding efficiency of p-ASA on the PCN/rGO electrode and detection limit were affected by some reaction parameters such as temperature and pH value. Appropriate temperature and pH can guarantee the maximum binding amount and achieve optimal analytical performance. As shown in Fig. S7A,† the response signals were nearly the same within the temperature ranging from 25 °C to 40 °C, while higher temperature inhibited the response signal. Thus, the sensor electrode was incubated in p-ASA samples at room temperature in this work. Fig. S7B† showed that the optimal pH value for incubation of p-ASA with the sensor electrode was 6.
Analytical performance of the developed PEC sensor.
To investigate the detectability of the developed PEC sensor, p-ASA standards with different concentrations were tested by using the above home-made PEC system under optimized conditions. The photocurrent enhancement coefficient (I/I0, where I0 and I are the photocurrent values of the PCN/rGO electrode in the presence of water and p-ASA solution, respectively) was employed as the quantitative criteria. As shown in Fig. 4A, the I/I0 value increased with the increase of p-ASA concentration and was proportional to the logarithm of the p-ASA amount in two consecutive linear ranges. One was from 10 ng L−1 to 100
000 ng L−1 (detailed fitted line shown in Fig. S8A†) with the linear regression equation of I/I0 = 0.124 × log
CASA + 1.04 (R2 = 0.997). The detection limit of 5.47 ng L−1 was calculated based on 3σ/slope (σ, standard deviation of the blank samples). As for the maximum allowable arsenic concentration of 10 μg L−1 in drinking water defined by the World Health Organization (WHO),36 this detection limit is rather lower than the sensitivity demand for drinking water detection. Furthermore, another linear calibration curve ranging from 100 μg L−1 to 10 mg L−1 (detailed fitted line exhibited in Fig. S8B†) was also found, and further increase of p-ASA concentration resulted in a gradual I/I0 value saturation.
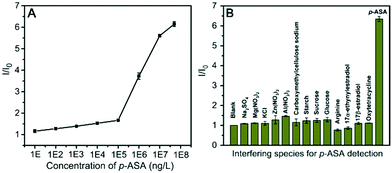 |
| Fig. 4 (A) Photocurrent increment of the PCN/rGO electrode towards different concentrations of p-ASA; (B) the selectivity of the PCN/rGO electrode for p-ASA (10 mg L−1) detection as compared to other interferences including various ionic substances (50 mg L−1), organic nutrients and aromatic compounds (1 mg L−1). | |
Next, the selectivity of the fabricated PEC sensor was investigated by challenging the sensing platform with some common species present in poultry excretion solution and natural water, including ionic substances, organic nutrients and aromatic compounds. As displayed in Fig. 4B, all of these interfering species caused no obvious change in the I/I0 value compared with the blank sample (PCN/rGO electrode incubated in water). Inspiringly, p-ASA caused a significant increase in the I/I0 value. This result demonstrated that the PEC sensor possessed excellent selectivity against interfering substances, which could be ascribed to the specific and strong binding of the PCN/rGO electrode to p-ASA.
Real sample detection.
Natural water contains relatively large amounts of dissolved organic matter (DOM), which may have adverse effect on p-ASA detection.37 Meanwhile, p-ASA is widely present in swine manure lixivium.38 In order to investigate the practicability of the fabricated PEC sensor, a certain concentration of p-ASA in simulated natural water (SNW) and swine manure lixivium (WML) was evaluated with the PCN/rGO electrode. The detailed preparation methods of two sorts of water samples were described in section 1 of the ESI.† Before detection, p-ASA standards with certain concentrations were spiked in the SNW and WML and then assayed using the PEC sensing system. The assay results including recoveries and relative standard deviations (RSD) were summarized in Table 1. The recoveries for SNW samples were 84.7–99.3% (with RSD lower than 7.7%), while the recoveries for WML were 86.2–100.4% (with RSD lower than 3.8%). These results demonstrated that the developed PEC sensor possessed relatively strong environmental tolerance and can promisingly be applied to detect p-ASA in complicated environmental samples.
Table 1 Detection of p-ASA in real samples by using the PEC sensor
Samples |
Added (μg L−1) |
Found (μg L−1) |
Recovery (%) |
RSD (%) (n = 3) |
Simulated natural water |
1 |
0.977 |
97.7 |
2.0 |
10 |
9.01 |
90.1 |
3.3 |
100 |
84.7 |
84.7 |
7.7 |
1000 |
933.4 |
99.3 |
0.3 |
Swine manure lixivium |
1 |
1.01 |
100.1 |
0.6 |
10 |
8.62 |
86.2 |
2.5 |
100 |
100.36 |
100.4 |
3.8 |
1000 |
936.0 |
93.6 |
0.7 |
3.3 Mechanism investigation
Study on the interaction between PCN/rGO and p-ASA.
Based on the above experimental results, the PCN/rGO electrode was sensitive to p-ASA. According to Fig. 3, the PCN/rGO electrode can bind the p-ASA molecule and result in enhanced photocurrent. Therefore, it is necessary to investigate the interaction between PCN/rGO and p-ASA. As shown in Fig. S9A,† the As 3d XPS spectra of pure p-ASA peaked at 45.01 eV, which corresponded to the binding energy of As 3d for phenylarsonic acid.39 After being captured by PCN/rGO, the binding energy of As 3d slightly shifted to 45.3 eV, which could be ascribed to the formation of Zr–O–As from the coordination between PCN/rGO and p-ASA.40 According to the XPS spectra of Zr 3d (Fig. S9B†), the position of Zr 3d in PCN/rGO shifted to a higher binding energy after the adsorption of p-ASA, which further confirmed the Zr–O–As coordination interaction.41 In addition, a characteristic peak of π–π* occurred at 291.8 eV in the XPS spectrum of C 1s for PCN/rGO after adsorption of p-ASA (Fig. S9C†), revealing that π–π stacking also played a key role in the interaction between PCN/rGO and p-ASA.42
The mechanism of p-ASA adsorption-induced PEC signal enhancement.
As shown in Fig. 4A, the adsorption of p-ASA on the PCN/rGO electrode resulted in enhanced cathodic photocurrent. Measuring the photocurrent amplitude of the PCN/rGO electrode is the main working principle on the detection of p-ASA in this work. Thus, it is necessary to clarify two issues: i) the cathodic photocurrent related reactions occurring on the PCN/rGO electrode and ii) the enhancement mechanism of photocurrent for p-ASA adsorption. As displayed in Fig. S10,† the surface morphology of the PCN/rGO electrode after p-ASA adsorption does not change after PEC testing by comparing the related SEM images. The intact surface structure after PEC testing suggests that the photocurrent response did not originate from the corrosion reactions on the electrode surface. As shown in Fig. 5A, the cathodic photocurrent of the PCN/rGO electrode was about 1 μA in the argon-saturated electrolyte, while the photocurrent increased to about 6 μA in the air-saturated electrolyte. This result revealed that oxygen (O2) played a key role in the cathodic PEC reaction of the PCN/rGO electrode. The TCPP in PCN-224 is regarded as a light harvesting element, where electrons are pumped from the valence band (HOMO) to the conduction band (LUMO) of PCN-224 by photoexcitation.22 Dissolved O2 might be reduced to a superoxide radical (O2˙−) by photogenerated electrons, thus facilitating the cathodic photocurrent.43 A similar phenomenon was also observed on the PCN/rGO electrode after adsorbing p-ASA (Fig. S11†).
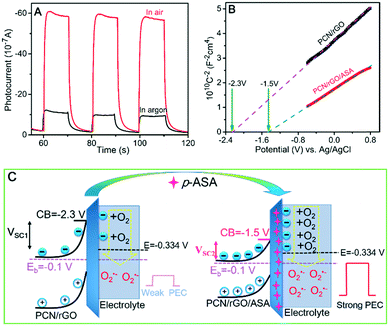 |
| Fig. 5 (A) Photocurrent response of the PCN/rGO electrode in an electrolyte (HEPES buffer) saturated with argon (black curve) and air (red curve); (B) Mott–Schottky plots of the PCN/rGO electrode in an electrolyte (HEPES buffer) before and after adsorption of p-ASA; (C) illustration of the mechanism of photocurrent enhancement through adsorption of p-ASA on the PCN/rGO electrode. | |
It is well-established that the physical properties of semiconductors, such as width of the band gap and the band position relative to the redox potential of the reaction and charge concentration, could substantially influence the PEC reactions. As shown in Fig. S12A,† the band gap of PCN/rGO was 1.58 eV in terms of Tauc plots, indicating that it can absorb the whole spectrum of visible light. After adsorbing p-ASA, the band gap of PCN/rGO shifted to 1.56 eV (Fig. S12B†). The change of the band gap was too small to affect the light absorption capability and photocurrent of the PCN/rGO electrode. Thus, determination of the band position and conductive type of the PCN/rGO becomes important for understanding the enhancement mechanism.
Obtained with a fixed frequency of 10 kHz, the flat band potential (Vfb) of the PCN/rGO electrode calculated from the Mott–Schottky plot was −2.3 V (vs. Ag/AgCl) (Fig. 5B, black line). The Vfb shifted positively to −1.5 V (vs. Ag/AgCl) after adsorption of p-ASA (Fig. 5B, red line). On the other hand, the positive slopes of the Mott–Schottky plots of both electrodes indicate that the PCN/rGO is an n-type semiconductor and the adsorption of p-ASA does not change the conducting type.44 Therefore, upward band bending is expected when the PCN/rGO electrode contacts with the electrolyte based on the principle of semiconductor electrochemistry.45 Thus, the “built-in” electric field at the semiconductor–electrolyte interface, pointing from the bulk of the PCN/rGO electrode to the electrolyte, would hinder the photogenerated electrons from moving across the interface. However, observation of the photocurrent demonstrated that certain amounts of electrons indeed went to the electrolyte to reduce O2. Moreover, the PCN/rGO electrode was immersed in an electrolyte containing different concentrations of K3[Fe(CN)6] for PEC testing. As shown in Fig. S13,† the dark current increased with the increment of K3[Fe(CN)6] concentration, which indicates that the electrons can easily move across the interface to reduce Fe3+ even under dark conditions. This phenomenon confirms that the cathodic photocurrent is ascribed to the reduction reaction by electron transfer in spite of the unfavourable electrical field.
In this case, it is believed that the strength of the electric field (E) and the width of the space charge region (SCR) (or depletion layer) are the main factors determining the amplitude of photocurrent.46 The weaker the E and the shorter the SCR, the higher the expected photocurrent. As illustrated in Fig. 5C, since the bias voltage (Eb) of both PCN/rGO and PCN/rGO/ASA was controlled at −0.1 V (vs. Ag/AgCl) during measurements, the band bending (potential drop across the depletion layer) was solely related to the Vfb of the two electrodes. As shown in Fig. 5C, the band bending at the depletion layer of PCN/rGO was 2.2 V, which was larger than that of the PCN/rGO/ASA of 1.4 V. According to the slope of Mott–Schottky plots and related formulas (see calculation details in section 3 of the ESI†), the width of the SCR of 2.5 × 10−7 cm for PCN/rGO and of 1.69 × 10−7 cm for PCN/rGO/ASA was determined. And the strength of the electric field was 8.84 × 106 V cm−1 and 8.22 × 106 V cm−1 for PCN/rGO and PCN/rGO/ASA, respectively. Thus, the shortened depletion layer and weakened interfacial electric field of PCN/rGO/ASA were considered as the main reasons for strongly enhanced photocurrent of p-ASA in comparison with the bare PCN/rGO electrode. In other words, more photoinduced electrons could easily cross the weaker interfacial electric field over a shorter depletion layer to reduce O2 with its redox potential located at −0.334 V (vs. Ag/AgCl) (see Fig. 5C).47 In summary, the observed cathodic photocurrent on the sensor electrode was attributed to O2 reduction. The shortened depletion layer and weakened electric field caused by adsorption of p-ASA were ascribed to be the underlying mechanism of enhanced photocurrent.
4 Conclusions
In summary, we have developed a novel turn-on PEC sensor by employing the n-type PCN/rGO nanocomposite acting as both the photocathode and binding unit for p-ASA detection. In the PCN/rGO composite, rGO can remarkably promote the efficiency of photogenerated electron–hole separation, and the composite achieved a stable and high PEC signal. The PEC sensor was sensitive to p-ASA with a wide detection range from 10 ng L−1 to 10 mg L−1 and a low detection limit of 5.47 ng L−1. The mechanism study demonstrated that the PCN/rGO had strong affinity to p-ASA through Zr–O–As coordination and π–π stacking, and p-ASA as the adsorbate tuned the band position of the PCN/rGO electrode, causing a shorter distance of the depletion layer as well as weaker intensity of the electric field. Thus, there were more electrons passing over the potential barrier to reduce O2 which generated increased photocurrent. This work not only designs a novel electrode material with photoelectric activity and binding characteristic to fabricate a PEC sensor for p-ASA detection but also broadens the application of the functional MOF-based nanocomposite in environmental detection.
Conflicts of interest
There are no conflicts to declare.
Acknowledgements
This work was supported by the National Natural Science Foundation of China (Grant No. 21836002, 21607045 and 21777046), the Guangdong Innovative and Entrepreneurial Research Team Program (No. 2016ZT06N569), Guangzhou Science and Technology Project (No. 201804010189) and Shaoguan special fund for soil pollution prevention and control (No. 2017sgtyfz103). We greatly appreciate Dr. Xinzhou Ma (Foshan University) for his constructive suggestion and modification of the manuscript.
Notes and references
- S. S. Pawitwar, V. S. Nadar, A. Kandegedara, T. L. Stemmler, B. P. Rosen and M. Yoshinaga, Biochemical characterization of arsI: A novel C-As lyase for degradation of environmental organoarsenicals, Environ. Sci. Technol., 2017, 51, 11115–11125 CrossRef CAS PubMed.
- K. P. Mangalgiri, A. Adak and L. Blaney, Organoarsenicals in poultry litter: detection, fate, and toxicity, Environ. Int., 2015, 75, 68–80 CrossRef PubMed.
- G. A. Wasserman, X. Liu, F. Parvez, H. Ahsan, P. Factor-Litvak, J. Kline, A. Van Geen, V. Slavkovich, N. J. LoIacono and D. Levy, Water arsenic exposure and intellectual function in 6-year-old children in Araihazar, Bangladesh, Environ. Health Perspect., 2006, 115, 285–289 CrossRef PubMed.
- C. Wu, J. Tu, W. Liu, J. Zhang, S. Chu, G. Lu, Z. Lin and Z. Dang, The double influence mechanism of pH on arsenic removal by nano zero valent iron: electrostatic interactions and the corrosion of Fe0, Environ. Sci.: Nano, 2017, 4, 1544–1552 RSC.
- D. Chen, H. Zhang, Y. Tao, Y. Wang, L. Huang, Z. Liu, Y. Pan, D. Peng, X. Wang, M. Dai and Z. Yuan, Development of a high-performance liquid chromatography method for the simultaneous quantification of four organoarsenic compounds in the feeds of swine and chicken, J. Chromatogr., B, 2011, 879, 716–720 CrossRef CAS PubMed.
- B. P. Jackson and P. M. Bertsch, Determination of arsenic speciation in poultry wastes by IC-ICP-MS, Environ. Sci. Technol., 2001, 35, 4868–4873 CrossRef CAS PubMed.
- W. W. Zhao, J. J. Xu and H. Y. Chen, Photoelectrochemical bioanalysis: the state of the art, Chem. Soc. Rev., 2015, 44, 729–741 RSC.
- J. Su, X. Feng, J. D. Sloppy, L. Guo and C. A. Grimes, Vertically aligned WO3 nanowire arrays grown directly on transparent conducting oxide coated glass: synthesis and photoelectrochemical properties, Nano Lett., 2011, 11, 203–208 CrossRef CAS PubMed.
- B. Zhang, L. Lu, Q. Hu, F. Huang and Z. Lin, ZnO nanoflower-based photoelectrochemical DNAzyme sensor for the detection of Pb2+, Biosens. Bioelectron., 2014, 56, 243–249 CrossRef CAS PubMed.
- J. Zhuang, W. Lai, M. Xu, Q. Zhou and D. Tang, Plasmonic AuNP/g-C3N4 nanohybrid-based photoelectrochemical sensing platform for ultrasensitive monitoring of polynucleotide kinase activity accompanying DNAzyme-catalyzed precipitation amplification, ACS Appl. Mater. Interfaces, 2015, 7, 8330–8338 CrossRef CAS PubMed.
- W. X. Dai, L. Zhang, W. W. Zhao, X. D. Yu, J. J. Xu and H. Y. Chen, Hybrid PbS quantum dot/nanoporous NiO film nanostructure: preparation, characterization, and application for a self-powered cathodic photoelectrochemical biosensor, Anal. Chem., 2017, 89, 8070–8078 CrossRef CAS PubMed.
- J. Zhuang, D. Tang, W. Lai, M. Xu and D. Tang, Target-induced nano-enzyme reactor mediated hole-trapping for high-throughput immunoassay based on a split-type photoelectrochemical detection strategy, Anal. Chem., 2015, 87, 9473–9480 CrossRef CAS PubMed.
- R. Wu, G. C. Fan, L. P. Jiang and J. J. Zhu, Peptide-based photoelectrochemical cytosensor using a hollow-TiO2/EG/ZnIn2S4 cosensitized structure for ultrasensitive detection of early apoptotic cells and drug evaluation, ACS Appl. Mater. Interfaces, 2018, 10, 4429–4438 CrossRef CAS PubMed.
- S. Wu, Y. Zhu, Y. Huo, Y. Luo, L. Zhang, Y. Wan, B. Nan, L. Cao, Z. Wang, M. Li, M. Yang, H. Cheng and Z. Lu, Bimetallic organic frameworks derived CuNi/carbon nanocomposites as efficient electrocatalysts for oxygen reduction reaction, Sci. China Mater., 2017, 60, 654–663 CrossRef CAS.
- T. He, S. Chen, B. Ni, Y. Gong, Z. Wu, L. Song, L. Gu, W. Hu and X. Wang, Zirconium-porphyrin-based metal-organic framework hollow nanotubes for immobilization of noble-metal single atoms, Angew. Chem., 2018, 130, 3551–3556 CrossRef.
- H. Ting, H.-Y. Chi, C. H. Lam, K.-Y. Chan and D.-Y. Kang, High-permeance metal–organic framework-based membrane adsorber for the removal of dye molecules in aqueous phase, Environ. Sci.: Nano, 2017, 4, 2205–2214 RSC.
- M. Jian, H. Wang, R. Liu, J. Qu, H. Wang and X. Zhang, Self-assembled one-dimensional MnO2@ zeolitic imidazolate framework-8 nanostructures for highly efficient arsenite removal, Environ. Sci.: Nano, 2016, 3, 1186–1194 RSC.
- F. Y. Yi, M. Gu, S. C. Wang, J. Q. Zheng, L. Pan and L. Han, A gual-functional luminescent MOF sensor for phenylmethanol molecule and Tb3+ cation, Inorg. Chem., 2018, 57, 2654–2662 CrossRef CAS PubMed.
- S. M. J. Rogge, A. Bavykina, J. Hajek, H. Garcia, A. I. Olivos-Suarez, A. Sepulveda-Escribano, A. Vimont, G. Clet, P. Bazin, F. Kapteijn, M. Daturi, E. V. Ramos-Fernandez, I. X. F. X. Llabres, V. Van Speybroeck and J. Gascon, Metal-organic and covalent organic frameworks as single-site catalysts, Chem. Soc. Rev., 2017, 46, 3134–3184 RSC.
- C. Tian, J. Zhao, X. Ou, J. Wan, Y. Cai, Z. Lin, Z. Dang and B. Xing, Enhanced adsorption of p-arsanilic acid from water by amine-modified UiO-67 as examined using extended X-ray absorption fine structure, X-ray photoelectron spectroscopy, and density functional theory calculations, Environ. Sci. Technol., 2018, 52, 3466–3475 CrossRef CAS PubMed.
- T. Tachikawa, J. R. Choi, M. Fujitsuka and T. Majima, Photoinduced charge-transfer processes on MOF-5 nanoparticles: elucidating differences between metal-organic frameworks and semiconductor metal oxides, J. Phys. Chem. C, 2008, 112, 14090–14101 CrossRef CAS.
- Y. Z. Chen, Z. U. Wang, H. Wang, J. Lu, S. H. Yu and H. L. Jiang, Singlet oxygen-engaged selective photo-oxidation over Pt nanocrystals/porphyrinic MOF: the roles of photothermal effect and Pt electronic state, J. Am. Chem. Soc., 2017, 139, 2035–2044 CrossRef CAS PubMed.
- D. Feng, W. C. Chung, Z. Wei, Z. Y. Gu, H. L. Jiang, Y. P. Chen, D. J. Darensbourg and H. C. Zhou, Construction of ultrastable porphyrin Zr metal-organic frameworks through linker elimination, J. Am. Chem. Soc., 2013, 135, 17105–17110 CrossRef CAS PubMed.
- W. Salomon, C. Roch-Marchal, P. Mialane, P. Rouschmeyer, C. Serre, M. Haouas, F. Taulelle, S. Yang, L. Ruhlmann and A. Dolbecq, Immobilization of polyoxometalates in the Zr-based metal organic framework UiO-67, Chem. Commun., 2015, 51, 2972–2975 RSC.
- W. S. Hummers and R. E. Offeman, Preparation of graphitic oxide, J. Am. Chem. Soc., 1958, 80, 1339 CrossRef CAS.
- J. Park, Q. Jiang, D. Feng, L. Mao and H. C. Zhou, Size-controlled synthesis of porphyrinic metal-organic framework and functionalization for targeted photodynamic therapy, J. Am. Chem. Soc., 2016, 138, 3518–3525 CrossRef CAS PubMed.
- M. Jahan, Q. Bao, J. X. Yang and K. P. Loh, Structure-directing role of graphene in the synthesis of metal-organic framework nanowire, J. Am. Chem. Soc., 2010, 132, 14487–14495 CrossRef CAS PubMed.
- J. W. Liu, Y. Zhang, X. W. Chen and J. H. Wang, Graphene oxide-rare earth metal-organic framework composites for the selective isolation of hemoglobin, ACS Appl. Mater. Interfaces, 2014, 6, 10196–10204 CrossRef CAS PubMed.
- M. Jahan, Q. Bao and K. P. Loh, Electrocatalytically active graphene-porphyrin MOF composite for oxygen reduction reaction, J. Am. Chem. Soc., 2012, 134, 6707–6713 CrossRef CAS PubMed.
- R. Bissessur, P. K. Y. Liu and S. F. Scully, Intercalation of polypyrrole into graphite oxide, Synth. Met., 2006, 156, 1023–1027 CrossRef CAS.
- N. A. Travlou, K. Singh, E. Rodríguez-Castellón and T. J. Bandosz, Cu–BTC MOF–graphene-based hybrid materials as low concentration ammonia sensors, J. Mater. Chem. A, 2015, 3, 11417–11429 RSC.
- F. Zhang and M. P. Srinivasan, Self-assembled molecular films of aminosilanes and their immobilization capacities, Langmuir, 2004, 20, 2309–2314 CrossRef CAS PubMed.
- B. Sun, L. Chen, Y. Xu, M. Liu, H. Yin and S. Ai, Ultrasensitive photoelectrochemical immunoassay of indole-3-acetic acid based on the MPA modified CdS/RGO nanocomposites decorated ITO electrode, Biosens. Bioelectron., 2014, 51, 164–169 CrossRef CAS PubMed.
- Z. Kang, Y. Gu, X. Yan, Z. Bai, Y. Liu, S. Liu, X. Zhang, Z. Zhang, X. Zhang and Y. Zhang, Enhanced photoelectrochemical property of ZnO nanorods array synthesized on reduced graphene oxide for self-powered biosensing application, Biosens. Bioelectron., 2015, 64, 499–504 CrossRef CAS PubMed.
- M. Q. Yang, Y. J. Xu, W. Lu, K. Zeng, H. Zhu, Q. H. Xu and G. W. Ho, Self-surface charge exfoliation and electrostatically coordinated 2D hetero-layered hybrids, Nat. Commun., 2017, 8, 14224 CrossRef CAS PubMed.
- B. E. Erickson, Field kits fail to provide accurate measure of arsenic in groundwater, Environ. Sci. Technol., 2003, 37, 35A–38A CrossRef CAS PubMed.
- X. Wang, J. Lu and B. Xing, Sorption of organic contaminants by carbon nanotubes: influence of adsorbed organic matter, Environ. Sci. Technol., 2008, 42, 3207–3212 CrossRef CAS PubMed.
- X. Xie, Y. Hu and H. Cheng, Rapid degradation of p-arsanilic acid with simultaneous arsenic removal from aqueous solution using Fenton process, Water Res., 2016, 89, 59–67 CrossRef CAS PubMed.
- M. K. Bahl, R. O. Woodall, R. L. Watson and K. J. Irgolic, Relaxation during photoemission and LMM Auger decay in arsenic and some of its compounds, J. Chem. Phys., 1976, 64, 1210–1218 CrossRef CAS.
- C. O. Audu, H. G. T. Nguyen, C. Y. Chang, M. J. Katz, L. Mao, O. K. Farha, J. T. Hupp and S. T. Nguyen, The dual capture of As(V) and As(III) by UiO-66 and analogues, Chem. Sci., 2016, 7, 6492–6498 RSC.
- B. Li, X. Zhu, K. Hu, Y. Li, J. Feng, J. Shi and J. Gu, Defect creation in metal-organic frameworks for rapid and controllable decontamination of roxarsone from aqueous solution, J. Hazard. Mater., 2016, 302, 57–64 CrossRef CAS PubMed.
- A. M. Puziy, O. I. Poddubnaya, R. P. Socha, J. Gurgul and M. Wisniewski, XPS and NMR studies of phosphoric acid activated carbons, Carbon, 2008, 46, 2113–2123 CrossRef CAS.
- D. Schlettwein, N. I. Jaeger and D. Wöhrle, Photoelectrochemical investigations of molecular semiconductors: characterization of the conduction type of various substituted porphyrins, Berichte der Bunsengesellschaft für physikalische Chemie, 1991, 95, 1526–1530 CrossRef CAS.
- J. Yan, T. Wang, G. Wu, W. Dai, N. Guan, L. Li and J. Gong, Tungsten oxide single crystal nanosheets for enhanced multichannel solar light harvesting, Adv. Mater., 2015, 27, 1580–1586 CrossRef CAS PubMed.
-
K. Rajeshwar, in Fundamentals of semiconductor electrochemistry and photoelectrochemistry, ed. S. Licht, Wiley-VCH, Weinheim, 2001, pp. 8–9 Search PubMed.
- J. Krüger, U. Bach and M. Grätzel, Modification of TiO2 heterojunctions with benzoic acid derivatives in hybrid molecular solid-state devices, Adv. Mater., 2000, 12, 447–451 CrossRef.
- J. Petlicki and T. G. M. V. D. Ven, The equilibrium between the oxidation of hydrogen peroxide by oxygen and the dismutation of peroxyl or superoxide radicals in aqueous solutions in contact with oxygen, J. Chem. Soc., Faraday Trans., 1998, 94, 2763–2767 RSC.
Footnote |
† Electronic supplementary information (ESI) available. See DOI: 10.1039/c8en00913a |
|
This journal is © The Royal Society of Chemistry 2019 |
Click here to see how this site uses Cookies. View our privacy policy here.