Involvement of ethylene signaling in zinc oxide nanoparticle-mediated biochemical changes in Arabidopsis thaliana leaves†
Received
3rd September 2018
, Accepted 5th December 2018
First published on 6th December 2018
Abstract
The growing use of metallic nanoparticles in industry has resulted in their accumulation in agricultural land, which poses a serious threat to the yield and quality of crops worldwide. Various reports showed that plants face environmental stress through hormones; however, the regulation of ethylene under ZnO NP stress is still unknown. In this study, ethylene-signaling defective mutants (etr1-3 and ein2-1) were studied in comparison to Arabidopsis wild-type under ZnO NPs stress. We found that ZnO NPs significantly inhibited the growth of Arabidopsis and induced toxicity by regulating the expression of cell cycle-related genes. More importantly our results showed the involvement of ethylene signaling and biosynthesis in this process. The ZnO NPs affected the biomass, and chlorophyll and sugar contents in both the ethylene-insensitive mutants and wild-type plants. The higher ROS accumulation and increased lipid peroxidation showed that the ZnO NPs induced toxicity in Arabidopsis. The observed high antioxidant enzyme activities and mRNA transcript levels of their corresponding genes in the mutant plants clearly showed the tolerance of the ethylene-insensitive mutants compared to the wild-type plant against oxidative damage caused by ZnO NP stress. Overall, our results showed that the ethylene-insensitive mutants tolerated ZnO NP-induced stress more efficiently than WT. These results suggest that ethylene induces oxidative damage in plants under ZnO NP stress.
Environmental significance
Zinc oxide nanoparticles (ZnO NPs) are significant metallic oxide nanoparticle due to their special physico-chemical properties. The utilization of these nano-particles is increasing in various fields. This study has significance since the number of investigations including phytohormone interaction with ZnO NPs are limited. Our findings showed that ZnO NPs induced toxicity and ethylene is involved in this process, which was further confirmed by the increased activity of the EBS::GUS lines under high exposure to ZnO NPs. These findings reaffirm the involvement of ethylene in ZnO NP toxicity. Furthermore, the significance of this investigation is that it provides insight into the modification of phytohormone pathways, which can be used to enable crops to cope with the alarming toxicity of ZnO NPs in the environment.
|
1. Introduction
Nanoparticles (NPs) are particles with one dimension and diameter ranging from 1 to 100 nm.1 Due to their unique properties and novel features, NPs are utilized in various aspects of daily life and energy production, such as cosmetics, catalysts, semiconductors, drug carriers and in tomato plants.2–4 However, their extensive use may become a health risk when they are released into the atmosphere.5 The use of nanoparticles in fertilizers and pesticides is the main way they enter the soil.6
Zinc oxide nanoparticles (ZnO NPs) are one of the most important metallic nanomaterials, which are used in various fields due to their specific chemical and physical properties.7,8 ZnO NPs are employed in the rubber industry because they can protect rubber composites and enhance the intensity and toughness performance of high polymers.9,10 Furthermore, due to their higher UV absorption ability, ZnO NPs are widely used in personal care products, such as sunscreen and cosmetics.11 These are also used in other industries, including the electronics, concrete production, photocatalysis, and electrotechnology industries.9,12 Moreover, ZnO is the third most widely used metal in NPs, where their estimated global production in between 550 and 33
400 tons per year.13–15 The environmental concentration of ZnO NPs were found to be in the range of 3.1–31 μg kg−1 and 76–760 μg L−1 in soil and water, respectively.16,17 ZnO NPs are the fifth most widely used materials in consumer products, as identified by a nanotechnology consumer product inventory.18
According to transcriptomic studies, nanoparticles up-regulate the expression of stress (biotic and abiotic)-related genes.19,20 In tomato plants, nanoparticles induced ROS generation, affected photosynthetic pigments, and finally resulted in cell death.21 Recently, a comparative study of silver nanoparticles (AgNPs) and Ag+ in Arabidopsis thaliana showed that AgNPs reduced its fresh weight and photosynthetic capacity. Besides, they also showed chronic effects on plant yield and flowering time of Arabidopsis thaliana.22 Copper oxide nanoparticles with different concentrations (2, 5, 10, 20, 50, and 100 mg L−1) increased the accumulation of O2 and H2O2 in the roots and leaves of Arabidopsis thaliana.23 Aluminium oxide nanoparticles (Al2O3 NPs) induced toxicity in wheat roots by increasing H2O2 levels and lipid peroxidation.24 Cerium oxide (CeO2 NPs) and indium oxide nanoparticles (In2O3 NPs) altered the antioxidant defense system in Arabidopsis seedlings.25 A life cycle study on peanuts (Arachis hypogaea L.) demonstrated their physiological changes with different metallic nanoparticles.26 Cerium oxide nanoparticles induced oxidative stress by increasing lipid peroxidation in Asparagus lettuce.27 Besides plants, nanoparticles also showed toxicity in other organisms, such as titanium dioxide nanoparticles (TiO2 NPs) induced toxicity in zebrafish including organ pathology, malformations, delay metamorphogenesis and DNA damage.28
The adverse influence of ZnO NPs on plant growth and development has been reported in several studies. One of the previous studies also reported that ZnO NPs with concentrations of 100 μM and 200 μM enhanced H2O2 and lipid peroxidation in wheat seedlings, respectively.29 Beside toxicity, ZnO NPs at a lower concentration (10 mg kg−1) enhanced the biomass and net photosynthetic rate in lettuce.30 In maize plants, they altered the expression pattern of different genes in their roots and shoots.31 Also, ZnO NPs inhibited root growth in different species of plants (Zea mays L., Brassica napus L., Cucumis sativus L., Raphanus sativus L., Lactuca sativus L., and Lolium perene L.),32 and have the capability to prompt the generation of reactive oxygen species (ROS), which may lead to cell death.3,33 A reduction in chlorophyll production was observed in Zea mays plants grown in soil modified with 800 mg kg−1 of ZnO NPs.34 ZnO NPs also induced oxidative stress and altered the antioxidant enzyme activities in rice seedlings.35 Therefore it is very important to study the antioxidant enzyme activities of plants under ZnO NPs stress.
Plant hormones act as signaling molecules under biotic and abiotic stress.36 Among them, ethylene, which is involved in various physiological, biochemical and molecular processes during the life cycle of plants, enhanced ROS accumulation.37 It was found to enhance ROS accumulation under different abiotic stresses, such as cadmium (cd), drought, high salinity, and low temperature.38–41 A previous report suggested that the effects of oxidative stress decreased by using an ethylene inhibitor or through mutation in ethylene genes.42 In another report it was also mentioned that exogenous use of ethylene could accelerate ROS generation in Arabidopsis plants.43
Ethylene is produced from methionine via S-adenosyl L methionine and 1-aminocyclopropane-1-carboxylic acid (ACC), which is catalysed by (ACS) 1-aminocyclopropane-1-carboxylic acid synthase and (ACO) 1-aminocyclopropane-1-carboxylic acid oxidase.44 Two basic components of ethylene biosynthesis are ACS and ACO. Under heavy metal stress, ethylene production increase due to increased transcript levels of genes encoding these two proteins.45,46 Exposure to heavy metal stress, such as Cd, aluminium (Al) and BPA could regulate ethylene biosynthesis, which confirmed the involvement of ethylene-induced heavy metal toxicity in plants.47–49 Meanwhile, ethylene also plays a key role in plants adapting to abiotic stress.50 In Arabidopsis, ethylene enhanced the accumulation of soluble solutes under drought stress by decreasing oxidative stress.51–53 The aim of the current study was to clarify the antagonistic or synergistic interaction existing between ethylene and ZnO NPs toxicity. Investigation of ethylene-insensitive mutants and WT under ZnO NPs stress at the biochemical and molecular levels is a very convenient approach to explore the interaction between ethylene and ZnO NPs stress.
2. Materials and method
2.1. Characterisation of ZnO NPs
ZnO NPs (purity 99.9%) were purchased from Cw-nano (www.cwnano.com). The ZnO NP suspension were prepared using DI water.
The morphology and particle size of the ZnO NPs were determined via transmission electron microscopy (TEM, FEI Tecnai F20 S-TWIN). The crystal form of the ZnO NPs was detected by X-ray diffraction. The XRD data was collected in the 2θ range of 10–80°. The X-ray diffraction pattern of the ZnO NPs is shown in Fig. S1.†
The size distribution of the ZnO NPs in media (water suspension) with different concentrations (50, 100, 200, and 300 mg L−1) was determined using a Malvern Zetasizer (Nano ZS, Malvern, UK). To minimize agglomeration we sonicated the different concentration of ZnO NP suspensions for 1 h before use.
2.2. Plant material and ZnO nanoparticle treatment
In this study, Arabidopsis thaliana (Columbia ecotype) wild-type and the ethylene signaling defective mutants ein2-1 and etr1-3 together with the ethylene reporter EBS::GUS transgenic lines were used. Seeds of the ethylene-insensitive mutants (etr1-3 and ein2-1) were received from the Arabidopsis Biological Resource Centre Columbus, OH, USA, as described before.54 Seeds of all genotypes were sterilized for 10 minutes in 5% sodium hypochlorite, then washed 5 times with autoclaved distilled water and stored in the dark at 4 °C for 3 days. For six days, the seeds were grown on ½ MS medium at pH 5.8. The seedlings were placed in a growth room under controlled growth conditions for Arabidopsis, as described previously.55–58 After six days the seedlings were transferred from the MS medium to soil pots. After two weeks, the plants were watered twice daily with 30 mL suspensions of ZnO NPs with different concentrations (0, 50, 100, 200, and 300 mg L−1) and supernatant of 300 mg L−1 of ZnO NPs. To obtain the supernatant, 300 mg L−1 of ZnO NPs were centrifuged at 5000 rpm for 15 min. For complete separation of the Zn2+ ions, further supernatant was filtered using 0.22 um filters. The supernatant containing Zn2+ ions released from the suspension with 300 mg L−1 concentration of ZnO NPs was used to evaluate if it had any effect on plant growth.
2.3. Biomass measurement
The plants were removed from the soil pots after 8 days of ZnO NPs stress. To remove debris material from the plant surface, the plants were washed with tap and distilled water, respectively. Paper towels were used to remove extra water. A digital balance was used to calculate the fresh weight (FW) of the plants, and their dry weight (DW) was measured after they were dried in a drying oven at 80 °C for 15 minutes followed by incubation at 65 °C in an incubator until completely dry.
2.4. GUS staining
To investigate the effect of ZnO NPs on ethylene biosynthesis and distribution, the expression of the ethylene reporter construct EBS::GUS was tested. GUS staining was carried out using the protocol described previously.54,59 Leaves of the EBS::GUS lines were immersed in GUS substrate supplemented with X-gluc solution overnight in the dark at 37 °C. The stained leaves were washed with following concentrations of ethanol: 30%, 50%, 70%, 95% and 100%, for 1 h at room temperature. GUS staining in the leaves was photographed with a Leica stereo microscope.
2.5. Measurement of chlorophyll and total soluble sugar contents
After 8 days of ZnO NPs treatment, the chlorophyll contents (Chla and Chlb) of the fresh leaves were determined. Pure acetone, pure ethanol and distilled water (4.5
:
4.5
:
1) were used to extract the chlorophyll contents (Chla and Chlb). The chlorophyll (a and b) contents were measured spectrophotometrically (SPECTROstarNano) at wavelengths of 663 and 645 nm, respectively, using the equations reported in ref. 60.
The fresh leaves of the Arabidopsis ethylene mutants and wild-type plants were weighed (0.05 mg) and then crushed in 5 mL 95% ethanol following the method in ref. 61. The homogenized samples were centrifuged at 12
000 rpm to obtain 1 mL supernatant. 4 mL anthrone was mixed with 1 mL supernatant and heated in a water bath for 10 min. After cooling the mixture, the sugar contents were measured using a UV-VIS spectrophotometer (UV-2450, SHIM ADZU) at a wavelength of 620 nm.
2.6. Measurement of anti-oxidant enzymes activity
To measure the anti-oxidant enzymes activity, 0.3 g fresh sample was homogenized in potassium phosphate buffer (6 mL of 50 mM with pH 7.8). The homogenized samples were centrifuged at 4 °C for 15 min at 14
000 rpm and the extracted supernatant was used to further measure enzyme activities using a UV-VIS spectrophotometer (UV-2450, SHIM ADZU).
The activity of SOD was measured using the nitroblue tetrazolium (NBT) photochemical assay and protocol in ref. 62. The activity was measured spectrophotometrically at a wavelength of 560 nm.
POD activity was assessed following the protocol described in ref. 63.
The ascorbate activity (APX) was determined at a wavelength of 290 nm by following the protocol in ref. 64.
The activity of catalase (CAT) was assessed by following the method in ref. 65. The activity was measured at a wavelength of 240 nm.
2.7. Malondialdehyde analysis (MDA)
According to the method in ref. 66, 0.2 g leave sample was homogenized in 1.6 mL 10% trichloroacetic acid (TCA), centrifuged at 12
000 × g for 10 min, then the resulting supernatant was mixed with an equal volume of 0.67% 2-thiobarbituric acid (TBA), then boiled at 100 °C for 30 min and finally cooled in an ice bath. The MDA contents were measured at an OD of 532 nm and 600 nm.
2.8. Histochemical analysis of H2O2 and O2 by DAB and NBT in leaves of Arabidopsis
According to the protocol in ref. 67, accumulation of H2O2 was determined by staining the leaves of control plants and the plants treated with 300 mg L−1 of ZnO NPs with 3,3-diaminobenzidine (DAB) solution. The accumulation of O2 was identified by the staining leaves with nitroblue tetrazolium (NBT) solution according to the method described in ref. 49.
2.9. Quantitative real-time PCR
TRIzol reagent was used to extract total RNA from the leaves of all genotypes. Quality and concentration of RNA were determined with a Nanodrop 2000 UV-vis spectrophotometer. RNA was converted into cDNA using oligo dT 18 primers and M-MLV transcriptase (Promega). The Roche LightCycler 96 quantitative PCR system was used to conduct the gene expression experiment using 96-well plates. Actin2 was used as an internal reference gene to normalize the expression of all genes. The primers used in this study are listed in Table S1.†41,54 The mRNA transcript level of all genes were examined following the method described in ref. 57 and 68.
2.10. Statistical analysis
The data was evaluated via two-way analysis of variance (ANOVA) using the SPSS statistical software (Chicago, USA). Tukey's HSD test at P < 0.05 was used to compare the means. The standard error was illustrated in the figures. The experiments were performed with three replicates with similar results.
3. Results and discussion
3.1. Characterization of ZnO NPs
The TEM images show that the ZnO NPs have a hexagonal shape with an average size of 30 nm (Fig. 1A and B). XRD was also used for the characterization of the ZnO NPs. The peaks in the XRD pattern of the ZnO NPs clearly show their crystalline nature. The sample exhibited diffraction peaks corresponding to the pure ZnO NPs planes of (100), (002), (101), (102), (110), (103) and (112), as shown in Fig. S1.† These diffraction peaks are consistent with the standard peaks of bulk ZnO NPs, which have a hexagonal wurtzite structure.
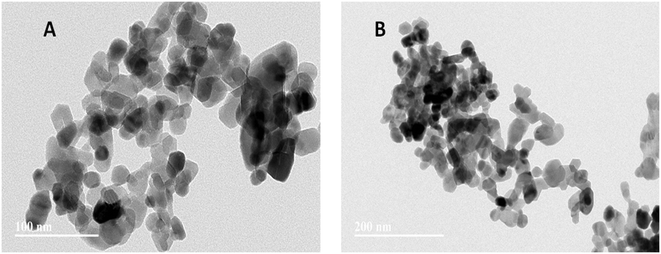 |
| Fig. 1 Characterization of the ZnO NPs. (A and B) TEM images of the ZnO NPs. | |
Based on the particle size distribution in the exposure medium (water suspension), the hydrodynamic size and ζ potential (mV) of the ZnO NPs were investigated using a Malvern Zetasizer (Nano ZS, Malvern, UK). The hydrodynamic particle size and ζ potential at different concentrations of ZnO NPs (50, 100, 200, and 300 mg L−1) are given in Table 1. The hydrodynamic particle size increased in the exposure medium compared to the solid state. Hydration layers capped on the surface of particles can increase their particle size in water. Therefore, the hydrodynamic size of the ZnO NPs was larger in water than in the solid state (TEM size). A change in particle size and agglomeration of ENPs in the exposure medium were previously reported.69–71 Another report also indicated that larger particles formed in the exposure medium, which gravitated and left behind assemblies of smaller particles.72 The ZnO NPs were dispersed in water suspension and had positive ζ potential at all concentrations of ZnO NPs, as shown in (Table 1). However, the overall hydrodynamic size of the ZnO NPs decreased at higher testing concentrations (100, 200, and 300 mg L−1), but their zeta potential slightly increased in comparison to that at a low concentration (50 mg L−1). Similarly, as previously reported, the size of the agglomerated ZnO NPs decreased with an increase in exposure concentration, but their zeta potential did not significantly increase at higher testing concentrations (125, 250, and 500 mg L−1) of ZnO NPs.72 These observations are consistent with our findings. Moreover, in our study, the testing concentrations (100, 200 and 300 mg L−1), with a higher zeta potential proved more toxic to the plants than the lower concentration (50 mg L−1), with a lower zeta potential. Nanoparticles with a positive surface charge show higher cytotoxicity compared to negatively charged nanoparticles. In this case, the uptake and translocation of positive ζ potential nanoparticles are easier compared to negative ζ potential particles.73
Table 1 Size and ζ potential (mV) of the different concentration ZnO NP suspensions in water. Each data value is the mean of triplicate measurements (mean ± SE)
30 nm ZnO NPs in distilled water |
Size (nm) |
Zeta potential (mV) |
50 mg L−1 |
380.4 ± 8.7 |
11.2 ± 0.3 |
100 mg L−1 |
305.06 ± 4.2 |
19.9 ± 0.2 |
200 mg L−1 |
319.1 ± 3.7 |
19.3 ± 0.6 |
300 mg L−1 |
296.6 ± 2.2 |
16.6 ± 0.3 |
3.2. Physiological responses of Arabidopsis under ZnO NPs stress
To illustrate the effect of ZnO NPs on Arabidopsis plants, 22 day-old plants were subjected to different concentrations of ZnO NPs (0, 50, 100, 200 and 300 mg L−1) and supernatant of 300 mg L−1 of ZnO NPs in soil pots for 8 days. The results clearly showed that the rosette size and weight (FW and DW) of the wild-type plants decreased significantly at higher ZnO NPs concentration (Fig. 2A–C). The supernatant (S300) suspension of 300 mg L−1 of ZnO NPs had a negligible effect on the rosette size and weight (FW, DW) of the plants. Therefore, in our further study, we selected different concentrations of ZnO NPs (0, 50, 100, 200 and 300) mg L−1 without supernatant suspension. More importantly, our results revealed that the ZnO NPs significantly down-regulated the expressions of cell cycle-related genes (CYCA2-1, CYCB1-1, CYCD1-1, CYCD2-1, and E2FB) in the leaves of the WT Arabidopsis plants, as shown in Fig. 2D. The mRNA transcript level of CYCA2-1, CYCB1-1, CYCD1-1, CYCD2-1, and E2FB decreased by 8.3, 2.5, 4.16, 3.33, and 2.6 fold in the leaves of the WT seedlings in comparison to that in the control, respectively. These results suggest that ZnO NPs reduce plant growth by down-regulating the expressions of cell cycle-responsive genes.
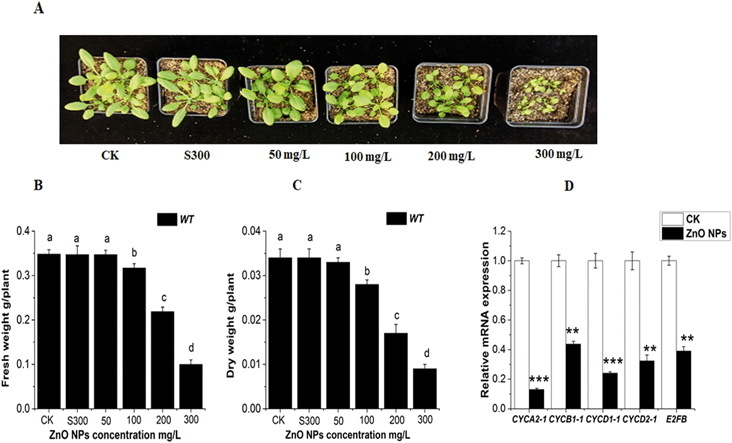 |
| Fig. 2 (A) Effect of the ZnO NPs on the Arabidopsis phenotype. (B) Fresh weight and (C) dry weight of the wild-type Arabidopsis plants subjected to 0, 50, 100, 200 and 300 mg L−1 of ZnO NPs and supernatant of the ZnO NP suspension. (E) Relative mRNA expression of the cell cycle-responsive genes in the wild-type plants subjected to 0 and 300 mg L−1 of ZnO NPs. Error bars illustrate mean ± SE. In the figure, the different letters show the significant difference by applying one way ANOVA with Tukey's HSD test at a probability of P < 0.05. One way ANOVA with LSD test was used at the probability of ** P > 0.01 and *** P > 0.001. | |
Nanoparticles have a negative effect on plant growth and development. They reduce the total biomass, elongation of roots and seed germination.74 It has already been reported that ZnO NPs reduce biomass accumulation and accelerate ROS generation, which result in cell death in plants.3,33 Although previous studies indicated the toxic effects of ZnO NPs on different plant species (lettuce, cucumber, ryegrass, radish and rape),32 to date, the involvement of ethylene in ZnO NP-induced toxicity in plants has not been investigated. According to previous studies, Zn2+ ions released from the supernatant of ZnO NPs did not cause any toxic effect on different plants (radish, rape, and ryegrass).32 Another report also demonstrated that the toxicity induced by ZnO NPs in rice and maize was caused by the nanoparticles themselves, not the Zn2+ ions released from the supernatant of the ZnO NPs.75 Hence, we believe that in this study, the toxic effects in Arabidopsis leaves are due to ZnO NP exposure. Therefore, in our further study we did not use the supernatant of the 300 mg L−1 of ZnO NP suspension.
Plants respond to harsh environments, which are most often responsible for their retarded growth and suppressed development by inhibition of mitosis.76,77 Abiotic stresses inhibit cell progression by changing the transcript levels of cell cycle-related genes.78 Our results demonstrated that the reduction in Arabidopsis growth and biomass could be due to the change in the mRNA transcript level of the genes related to the cell cycle under ZnO NP stress. The ZnO NPs significantly downregulated the expressions of the cell cycle genes CYCA2-1, CYCB1-1, CYCD1-1, CYCD2-1 and E2Fb, as shown in Fig. 2D. The plant hormone ethylene negatively regulates cell development, and it is also reported that ethylene signaling is involved in aluminum, alkaline and chromium Cr(VI) stress in Arabidopsis roots.47,54,79 Recently, in another report, it was highlighted that chromium Cr(VI) stress down-regulates the mRNA expressions of cell cycle-responsive genes and also induces higher ethylene levels in Arabidopsis leaves.80 These findings are consistent with our present study that ZnO NPs induce higher levels of ethylene in Arabidopsis leaves (Fig. 3A–C). From the results, this inhibits the cell division process in the leaves by down-regulating the expressions of cell cycle-responsive genes (Fig. 2D). Thus, it can be considered that ethylene may inhibit plant growth and development by repressing cell cycle-responsive genes expressions under ZnO NP stress. Collectively, it can be concluded that ethylene may inhibit plant growth and development by repressing cell cycle responsive genes.
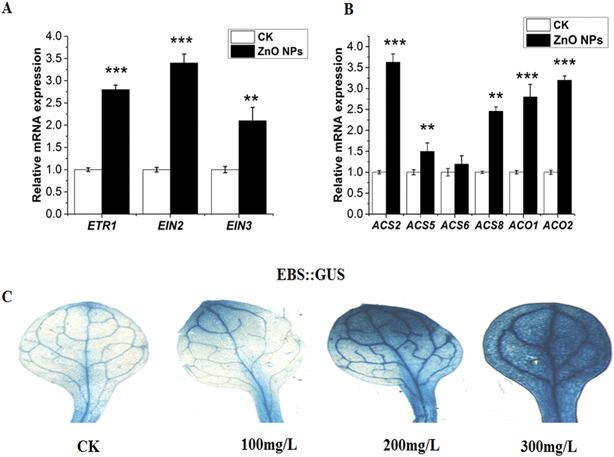 |
| Fig. 3 Involvement of ethylene signaling and biosynthesis in ZnO NP-induced toxicity and growth inhibition. (A) mRNA transcript level of the ethylene signaling genes in the wild-type leaves under 0 and 300 mg L−1 of ZnO NPs, (B) mRNA transcript level of the ethylene biosynthesis genes in the wild-type leaves under 0 and 300 mg L−1 of ZnO NPs, and (C) effect of different concentrations (0, 100, 200 and 300 mg L−1) of ZnO NPs on the accumulation of ethylene in the leaves of the ethylene reporter EBS::GUS transgenic lines. One way ANOVA with LSD test was used at the probability of ** P > 0.01 and *** P > 0.001. | |
3.3. Biosynthesis and signaling of ethylene is responsible for ZnO NP-induced growth regression
The relative expression of the ethylene signaling genes (ETR1, EIN2, and EIN3) was examined under 0 and 300 mg L−1 of ZnO NPs stress. The expression levels of ETR1, EIN2 and EIN3 were significantly up-regulated under 300 mg L−1 of ZnO NP stress (Fig. 3A). Moreover to confirm our hypothesis, we used the EBS::GUS transgenic lines and subjected them to 0, 100, 200 and 300 mg L−1 of ZnO NPs. A noticeable increase in the activity of EBS::GUS was observed, especially at a higher exposure (200 and 300 mg L−1) of ZnO NPs (Fig. 3C). These findings suggest that ethylene signaling is involved in ZnO NP-induced toxicity in Arabidopsis leaves. This increased ethylene signaling-induced inhibition of plant growth under ZnO NPs stress may be due to variations in the biosynthesis of ethylene. For further confirmation of our hypothesis that the overproduction of ethylene may be responsible for growth regression in Arabidopsis under ZnO NPs stress, we examined the mRNA expression levels of the genes (ACS2, ACS5, ACS6, ACS8, ACO1 and ACO2) responsible for ethylene production under 0 and 300 mg L−1 of ZnO NPs stress. All of these ethylene biosynthesis genes were significantly up-regulated at 300 mg L−1 of ZnO NPs stress (Fig. 3B). Overall, these results suggest that ethylene induces ZnO NP inhibition of plant growth.
In previous reports, it was confirmed that aluminum stress increases the ethylene signaling and biosynthesis in Arabidopsis roots and inhibits plant growth.47 However, the involvement of ethylene in ZnO NP-induced toxicity and growth inhibition in Arabidopsis leaves has not been investigated before. Copper stress significantly enhanced the expression pattern of the ACS genes in Arabidopsis thaliana.81 Copper also increased the mRNA expression levels of the ACS and ACO genes in B. oleracea.82 Our results here showed the up-regulation of the mRNA expression level of the ethylene signaling and biosynthesis of genes under 300 mg L−1 ZnO NPs. The up-regulation of these genes in Arabidopsis leaves accounts for the greater production of ethylene under ZnO NPs stress and decreased plant growth (Fig. 3A and B, respectively). An endogenous increase in ethylene production under ZnO NPs stress may cause deleterious effects on the growth and development of Arabidopsis.45 These findings are also in agreement with the previous report by Li et al.,79 who suggested that alkaline stress increased the biosynthesis and signaling of ethylene in Arabidopsis roots and reduced plant growth. Our hypothesis was further confirmed by the increase in activity of the EBS::GUS transgenic line under higher ZnO NPs concentrations (Fig. 3C). This clearly indicates the involvement of ethylene in ZnO NP-induced toxicity and growth inhibition in Arabidopsis.
3.4. Ethylene mutants alleviate ZnO NP-reduced rosette biomass accumulation in Arabidopsis thaliana
To further evaluate the involvement of ethylene in plant biomass reduction under ZnO NP stress, we used ethylene-insensitive mutants for comparison with the wild-type plants. ZnO NPs at lower concentrations did not have a significant effect on the FW and DW of all three genotypes. At (200 and 300 mg L−1) of ZnO NP exposure, the FW and DW of the seedlings were higher in both ethylene-insensitive mutants (etr1-3 and ein2-1) than the WT (Fig. S1†). These results clearly indicate that ethylene is involved in ZnO NP-induced rosette biomass reduction in Arabidopsis.
ZnO NPs at concentration of 2000 mg L−1 decreased the biomass in different plants (lettuce, cucumber, ryegrass, radish and rape).32 Our findings were supported by the role of ethylene in plant leaf growth inhibition.83 Further, another study also reported that ethylene-insensitive mutants in Arabidopsis have a higher rate of rosette biomass than the WT under high BPA stress.49 These findings support our data that ethylene may be involved in Arabidopsis growth inhibition under ZnO NP stress.
3.5. ZnO NPs caused reduction of chlorophyll and sugar contents in WT
Leaves of the WT and ethylene-insensitive mutants (etr1-3 and ein2-1) were used to measure the chlorophyll and sugar contents. No significant chlorophyll reduction was observed in the leaves at lower concentrations of ZnO NPs. Higher doses (200 and 300 mg L−1) of ZnO NPs significantly degraded the chlorophyll contents in all genotypes. This chlorophyll damage at a concentration of 300 mg L−1 of ZnO NPs was observed as a reduction in chl a of 60%, 41% and 29% and chl b of 80%, 61% and 37% in WT, etr1-3 and senescence ein2-1 (Fig. 4A and B), respectively. The total chlorophyll contents were significantly reduced in the WT compared to both ethylene-insensitive mutants (Fig. 4C). Furthermore, in response to ZnO NPs, the total soluble sugar content reduction was more pronounced in WT than both ethylene-insensitive mutants (Fig. 4D). At 300 mg L−1 of ZnO NPs, 38%, 35% and 25% reduction was observed in WT, etr1-3 and ein2-1, respectively. Overall, these results indicate that the ethylene mutants showed more resistance against chlorophyll degradation and sugar content reduction induced by ZnO NPs stress than the wild-type plants.
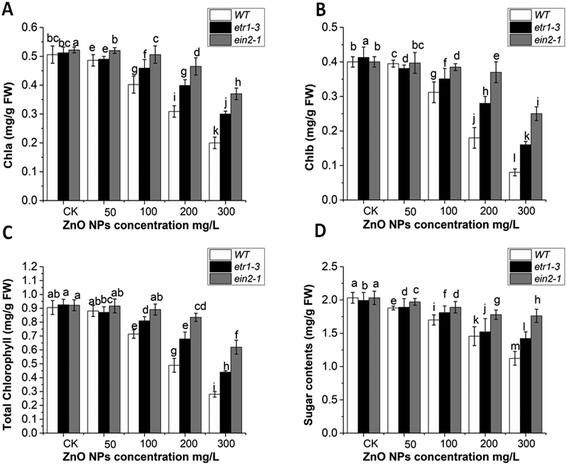 |
| Fig. 4 Effect of ZnO NPs on the light-harvesting pigments and total soluble sugar contents. (A) Chlorophyll a, (B) chlorophyll b, (C) total chlorophyll contents, and (D) total soluble sugar contents in the Arabidopsis ethylene mutants and wild-type plants subjected to (0, 50, 100, 200 and 300 mg L−1) of ZnO NPs. Error bars illustrate ± SE. In the figure, the different letters show the statistically significant difference. Two way ANOVA was used at the probability of P < 0.05. | |
Various reports investigated the involvement of nanoparticles in chlorophyll degradation.84,85 In Arabidopsis, 0.5 mg L−1 concentration of AgNPs was reported to inhibit chlorophyll synthesis and plant growth.86 In another report, it was also mentioned that AgNPs with different concentrations (0.2, 0.5, and 3 mg L−1) induced chlorophyll degradation.87 A significant decrease in chlorophyll content under copper oxide nanoparticles (CuO NPs) was also reported in Arabidopsis.23 This was the same for titanium dioxide nanoparticles (TiO2 NPs). Titanium dioxide nanoparticles (TiO2 NPs) reduced growth by reducing the chlorophyll pigments in Arabidopsis.88 Furthermore, the application of cerium dioxide nanoparticles with different concentrations (1000 and 2000 ppm) resulted in almost 60% and 85% chlorophyll reduction, respectively, in Arabidopsis.89 Senescence of plants naturally results in chlorophyll degradation,90 and ethylene is considered as the key modulator of senescence.91,92 This perspective of ethylene is quite consistent with our report that under ZnO NPs stress, both ethylene mutants had a higher chlorophyll content in comparison to their WT. This indicates that ethylene may enhance chlorophyll degradation in WT plants under ZnO NP stress. These findings are also consistent with an earlier report, which showed the involvement of ethylene-induced chlorophyll degradation under bisphenol A (BPA) stress.49 In conclusion, the lower chlorophyll contents in the WT plants strongly indicate the involvement of ethylene signaling-induced chlorophyll degradation under ZnO NP stress.
3.6. Involvement of ethylene in ZnO NP-induced ROS and MDA content accumulation
ROS accumulation and lipid peroxidation are the parameters of oxidative stress commonly used as cell damage indicators under environmental stress conditions. NBT and DAB staining were used to measure the reactive oxygen species (O2 and H2O) accumulation in the leaves of the ethylene mutants and WT plants, respectively. After exposure to 300 mg L−1 of ZnO NPs, all the genotypes had higher accumulation of H2O2 and O2 compared to their untreated control plants. Moreover, the accumulation was more pronounced in the leaves of the WT compared to the ethylene mutants (Fig. 5A).
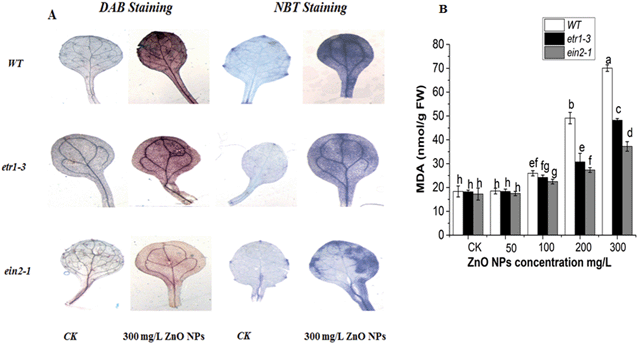 |
| Fig. 5 Involvement of ethylene in oxidative damage under ZnO NP stress. (A) DAB and NBT assays under 0 and 300 mg L−1 of ZnO NPs, (B) MDA content accumulation under different concentrations of ZnO NPs (0, 50, 100, 200 and 300 mg L−1) in the leaves of the ethylene mutants and WT plants. Error bars illustrate ± SE. In the figure, the different letters show the statistically significant difference at the probability of P < 0.05. | |
The production of MDA contents in the tissues of plants indicates ROS formation. Our results showed that at lower ZnO NPs concentrations (50 and 100) mg L−1, the MDA contents remained almost the same as that in the control plants, but significantly increased at higher doses (200 and 300 mg L−1) of ZnO NPs stress in all genotypes. This increase was 244%, 165% and 115% in the leaves of the WT, etr1-3 and ein2-1 plants, respectively (Fig. 4B). Thus, the reduced MDA contents in the ethylene mutants under 300 mg L−1 of ZnO NPs compared to the WT indicate that ethylene is involved in ZnO NP-induced toxicity in Arabidopsis (Fig. 5B).
Small-sized zinc oxide nanoparticles have the ability to enter and diffuse into plant cells and accelerate ROS production in plants.3 Similarly, in this study, the ZnO NPs accelerated ROS production in the Arabidopsis leaves (Fig. 5A and B). The higher rate of ROS cause lipid, protein and DNA damage, which led to cell death.93 Thus, the accumulation of ROS in Arabidopsis leaves under ZnO NP stress proves to be an important factor for plant growth inhibition. Therefore, it is very important to balance the ROS in plant cells. ROS are closely associated with the signaling network of hormones.94,95 Various studies illustrated the role of ethylene in the induction of oxidative burst.96 In the present study, MDA accumulation in the Arabidopsis leaves proved the toxicity of the ZnO NPs (Fig. 5B). A higher MDA content was recorded in the wild-type plants than both ethylene mutants, and previous reports also illustrated the involvement of ethylene in lipid peroxidation.97–99 It is considered that enhanced MDA contents in wild-type leaves may be toxic to cell organelles.100 These results suggested that ethylene may be involved in the induction of oxidative damage and increased lipid peroxidation under ZnO NPs stress. The results of the present study are in full agreement with the previous reports about the role of ethylene in regulating MDA content accumulation under aluminium stress.101 Similarly, histochemical staining clearly indicated that O2 and H2O2 were more accumulated in the leaves of the WT plants than both ethylene mutants (especially ein2-1) under 300 mg L−1 of ZnO NP stress (Fig. 5A). The generation of ROS in response to different stress situations is common in plants,102 which is deleterious for different types of biological molecules.103 Thus, herein, the higher accumulation of ROS in the wild-type leaves may result in toxicity to biological molecules and reduced plant growth under ZnO NP stress. These findings are consistent with the study of Cao et al.,42 who reported that ein2-1 mutants showed lower H2O2 and O2 accumulation under paraquat. The higher accumulation of ROS in the WT leaves revealed that ethylene amplifies oxidative damage under ZnO NPs.
3.7. Ethylene-insensitive mutants tolerate ZnO NP-toxicity more efficiently
To examine the possible role of antioxidant enzymes against ZnO NP-induced ROS accumulation, the SOD, POD, APX and CAT activities were analyzed in the leaves of etr1-3 and ein2-1 and compared with that in the WT plants. The ZnO NP stress altered the SOD, POD, CAT and APX activities in all the genotypes. These activities were found to be significantly higher in the ethylene signaling-defective mutants than the WT plants. This indicated that ethylene participates in ZnO NP-induced ROS accumulation in Arabidopsis. Under ZnO NPs stress, an increasing trend was observed in SOD activity except at 100 mg L−1 in both mutants and wild-type plants. However, as the dose of ZnO NPs increased (200 and 300 mg L−1), it was significantly higher in both ethylene mutants than in the WT plants (Fig. 6A). Higher POD activity was observed in the etr1-3 mutant than both the wild type and ein2-1 mutant at all ZnO NPs doses. However, at 50 mg L−1 of ZnO NP exposure, higher POD activity was recorded in WT than in the ein2-1 plants (Fig. 6B). In the control and at 50 mg L−1 of ZnO NP exposure, the APX activity remained higher in WT than etr1-3, but at higher exposure, it significantly declined in the WT plants compared to that in both mutants (Fig. 6C). In comparison to both ethylene mutants, the CAT activity in the wild-type was higher at exposure less than 100 mg L−1 ZnO NPs. However at 100, 200 and 300 mg L−1 of ZnO NPs, the CAT activity was significantly higher in both mutants than in the WT plants (Fig. 6D). Overall, our results showed higher antioxidant enzyme activities in the ethylene-insensitive mutants under 300 mg L−1 of ZnO NPs than that in the WT. This indicates that ethylene mutants can withstand ZnO NP-induced oxidative stress more efficiently than WT.
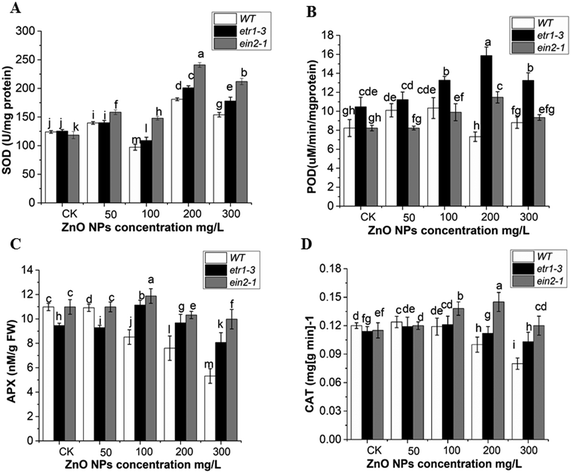 |
| Fig. 6 Effect of the ZnO NPs on enzyme activity. (A) SOD, (B) POD, (C) APX, and (D) CAT activities in the leaves of the Arabidopsis ethylene mutants and wild-type plants subjected to 0, 50, 100, 200 and 300 mg L−1 of ZnO NPs. Error bars illustrate ± SE. In the figure, the different letters show the statistically significant difference at the probability of P < 0.05. | |
For the conformation of the antioxidant enzymes response under ZnO NP stress at the molecular level, we examined the mRNA transcript levels of the genes related to the SOD, POD, APX, and CAT enzymes in the leaves of the wild-type and both ethylene mutants (Fig. 7). In all the genotypes, the relative mRNA expression level of SOD-related genes (MSD1 and CSD2) was maximum at high doses of ZnO NP stress (Fig. 7A and B). However, in comparison with WT, the transcript level of these genes remained significantly higher in both mutants. The mRNA transcript level of the POD3 gene was more up-regulated in the mutant plants exposed to ZnO NPs (especially at 200 mg L−1), while the WT showed a lower transcript level of POD3 gene at high doses of ZnO NPs (Fig. 7C). In case of the ethylene mutants, the transcript level of CAT2 increased in a dose-dependent manner, while the trend of the CAT2 gene showed a decline at 300 mg L−1 of ZnO NPs in all the genotypes, but it was significantly reduced in the WT than ethylene mutants (Fig. 7D). The mRNA expression level of the APX1 gene showed no significant changes in the WT at all doses of ZnO NP exposure. However, at 200 mg L−1 of ZnO NP exposure, significant up-regulation of the APX1 gene was observed for ein2-1 than etr1-3 and WT (Fig. 7E). Moreover, at a higher concentration (300 mg L−1) of ZnO NPs, a decrease in the expression pattern of the POD3, CAT2, and APX1 genes was observed. Taken together, our results demonstrated that WT had a lower transcript level of antioxidant enzyme-related genes than the ethylene mutants under ZnO NP stress.
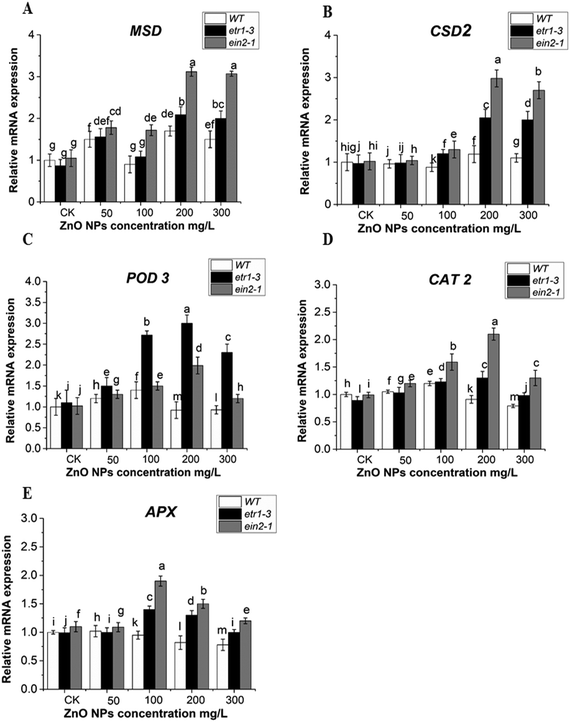 |
| Fig. 7 Effect of ZnO NPs on the transcript level of antioxidant enzyme synthesis genes. (A) MSD, (B) CSD2, (C) POD3, (D) CAT2, and (E) APX genes expression under different concentrations (0, 50, 100, 200 and 300 mg L−1) of ZnO NPs in the leaves of the Arabidopsis ethylene mutants and wild-type plants. Error bars illustrate ± SE. In the figure, the different letters show the statistically significant difference at the probability of P < 0.05. | |
ZnO NPs enhance the production of ROS in plants.3 Plants have a defense system in the form of antioxidant enzymes, which give protection against oxidative damage.104 This is similar to our data that ZnO NP exposure altered the antioxidant enzymes activities in Arabidopsis leaves. Previous reports illustrated that the coordination of CAT and SOD activities plays a protective role against oxidative stress induced by ROS (O2 and H2O2).105 The POD enzyme has the ability to detoxify H2O2.106 Our data showed that the activities of this antioxidant enzyme remained significantly higher in the ethylene mutants than in the wild-type. This clearly indicated the tolerance of the ethylene mutants to oxidative damage caused by ZnO NP stress (Fig. 5A–D). The increment in these antioxidant enzyme activities is supported by the greater up-regulation of their corresponding genes in the mutant plants (Fig. 7A–E). A previous report also suggested that the ein2-1 mutant had a higher mRNA transcript level of SOD- and CAT-responsive genes under paraquat stress.42 The decrease in the expression patterns of the POD3, CAT2, and APX1 genes at higher exposure (300 mg L−1) of ZnO NPs may be due to the higher rate of ROS accumulation in the Arabidopsis leaves. The expression patterns of some antioxidant enzyme-related genes have also been reported to be decreased under high chromium Cr(VI) and BPA stress in Arabidopsis seedlings.49,80 The increased antioxidant activities in ethylene mutants can decrease the ROS level by converting them into oxygen and water.107 Thus, the increased activation of the antioxidant defense system in the mutant plants revealed that the ethylene mutants can withstand ZnO NP toxicity more efficiently than WT.
4. Conclusions
ZnO NPs are generally toxic to plants because they induce biochemical changes, as observed by the alternations in sugar contents and chlorophyll contents, DAB and NBT staining and anti-oxidant defense system in all the genotypes. However, the WT Arabidopsis plants were more sensitive to ZnO NP toxicity compared to the ethylene-insensitive mutants. Ethylene is responsible for the down-regulation of cell cycle-related genes and reduced Arabidopsis growth under ZnO NP stress. This was confirmed by the up-regulation of the ethylene biosynthesis and signaling genes under ZnO NP exposure in the WT leaves, which was further supported by the increased activity of the EBS::GUS transgenic lines exposed to ZnO NPs. These results demonstrated the novel role of ethylene in response to ZnO NP stress. Moreover, further research on the molecular mechanisms related to the interaction between ethylene and ZnO NPs needs to be conducted.
Conflicts of interest
There are no conflicts of interest.
Acknowledgements
We are thankful to Dr. Javed Iqbal from College of Life Sciences and Oceanography, Shenzhen University, Shenzhen, P. R. China for reviewing this manuscript. The research was funded by the National Key R & D Program of China (2016YFD0100701); Major State Basic Research Development Program (973 Program, grant no. 2015CB150200); Zhejiang Provincial Natural Science Foundation of China (Grant No. LZ15C020001) and National Natural Science Foundation of China (Grant No. 31570183; 31529001; 31661143004) and China Postdoctoral Science Foundation (Grant No. 2018M632476).
References
- M. Auffan, J. Rose, J.-Y. Bottero, G. V. Lowry, J.-P. Jolivet and M. R. Wiesner, Towards a definition of inorganic nanoparticles from an environmental, health and safety perspective, Nat. Nanotechnol., 2009, 4, 634 CrossRef CAS PubMed.
- T. Xia, M. Kovochich, J. Brant, M. Hotze, J. Sempf, T. Oberley, C. Sioutas, J. I. Yeh, M. R. Wiesner and A. E. Nel, Comparison of the abilities of ambient and manufactured nanoparticles to induce cellular toxicity according to an oxidative stress paradigm, Nano Lett., 2006, 6, 1794–1807 CrossRef CAS PubMed.
- A. Nel, T. Xia, L. Mädler and N. Li, Toxic potential of materials at the nanolevel, Science, 2006, 311, 622–627 CrossRef CAS PubMed.
- M. Zaka, B. H. Abbasi, L.-u. Rahman, A. Shah and M. Zia, Synthesis and characterisation of metal nanoparticles and their effects on seed germination and seedling growth in commercially important Eruca sativa, IET Nanobiotechnol., 2016, 10, 134–140 CrossRef PubMed.
- A. A. Shvedova, V. E. Kagan and B. Fadeel, Close encounters of the small kind: adverse effects of man-made materials interfacing with the nano-cosmos of biological systems, Annu. Rev. Pharmacol. Toxicol., 2010, 50, 63–88 CrossRef CAS PubMed.
- T. Y. Sun, F. Gottschalk, K. Hungerbühler and B. Nowack, Comprehensive probabilistic modelling of environmental emissions of engineered nanomaterials, Environ. Pollut., 2014, 185, 69–76 CrossRef CAS PubMed.
- T. G. Smijs and S. Pavel, Titanium dioxide and zinc oxide nanoparticles in sunscreens: focus on their safety and effectiveness, Nanotechnol., Sci. Appl., 2011, 4, 95 CrossRef CAS PubMed.
- J. A. Ruszkiewicz, A. Pinkas, B. Ferrer, T. V. Peres, A. Tsatsakis and M. Aschner, Neurotoxic effect of active ingredients in sunscreen products, a contemporary review, Toxicol. Rep., 2017, 4, 245–259 CrossRef CAS PubMed.
- A. Kolodziejczak-Radzimska and T. Jesionowski, Zinc oxide—from synthesis to application: a review, Materials, 2014, 7, 2833–2881 CrossRef CAS PubMed.
- S. Sahoo, M. Maiti, A. Ganguly, J. Jacob George and A. K. Bhowmick, Effect of zinc oxide nanoparticles as cure activator on the properties of natural rubber and nitrile rubber, J. Appl. Polym. Sci., 2007, 105, 2407–2415 CrossRef CAS.
- M. D. Newman, M. Stotland and J. I. Ellis, The safety of nanosized particles in titanium dioxide–and zinc oxide–based sunscreens, J. Am. Acad. Dermatol., 2009, 61, 685–692 CrossRef CAS PubMed.
- F.-X. Xiao, S.-F. Hung, H. B. Tao, J. Miao, H. B. Yang and B. Liu, Spatially branched hierarchical ZnO nanorod-TiO 2 nanotube array heterostructures for versatile photocatalytic and photoelectrocatalytic applications: towards intimate integration of 1D–1D hybrid nanostructures, Nanoscale, 2014, 6, 14950–14961 RSC.
- O. Bondarenko, K. Juganson, A. Ivask, K. Kasemets, M. Mortimer and A. Kahru, Toxicity of Ag, CuO and ZnO nanoparticles to selected environmentally relevant test organisms and mammalian cells in vitro: a critical review, Arch. Toxicol., 2013, 87, 1181–1200 CrossRef CAS PubMed.
- M. Connolly, M. Fernández, E. Conde, F. Torrent, J. M. Navas and M. L. Fernández-Cruz, Tissue distribution of zinc and subtle oxidative stress effects after dietary administration of ZnO nanoparticles to rainbow trout, Sci. Total Environ., 2016, 551, 334–343 CrossRef PubMed.
- Y.-H. Peng, Y.-C. Tsai, C.-E. Hsiung, Y.-H. Lin and Y.-h. Shih, Influence of water chemistry on the environmental behaviors of commercial ZnO nanoparticles in various water and wastewater samples, J. Hazard. Mater., 2017, 322, 348–356 CrossRef CAS PubMed.
-
A. B. Boxall, Q. Chaudhry, C. Sinclair, A. Jones, R. Aitken, B. Jefferson and C. Watts, Current and future predicted environmental exposure to engineered nanoparticles, Central Science Laboratory, Department of the Environment and Rural Affairs, London,
UK, 2007, vol. 89 Search PubMed.
- M. Ghosh, S. Sinha, M. Jothiramajayam, A. Jana, A. Nag and A. Mukherjee, Cyto-genotoxicity and oxidative stress induced by zinc oxide nanoparticle in human lymphocyte cells in vitro and Swiss albino male mice in vivo, Food Chem. Toxicol., 2016, 97, 286–296 CrossRef CAS PubMed.
- A. Mukherjee, Y. Sun, E. Morelius, C. Tamez, S. Bandyopadhyay, G. Niu, J. C. White, J. R. Peralta-Videa and J. L. Gardea-Torresdey, Differential toxicity of bare and hybrid ZnO nanoparticles in green pea (Pisum sativum L.): A life cycle study, Front. Plant Sci., 2016, 6, 1242 Search PubMed.
- M. Thiruvengadam, S. Gurunathan and I.-M. Chung, Physiological, metabolic, and transcriptional effects of biologically-synthesized silver nanoparticles in turnip (Brassica rapa ssp. rapa L.), Protoplasma, 2015, 252, 1031–1046 CrossRef CAS PubMed.
- P. Landa, S. Prerostova, S. Petrova, V. Knirsch, R. Vankova and T. Vanek, The transcriptomic response of Arabidopsis thaliana to zinc oxide: A comparison of the impact of nanoparticle, bulk, and ionic zinc, Environ. Sci. Technol., 2015, 49, 14537–14545 CrossRef CAS PubMed.
- B. Ahmed, M. S. Khan and J. Musarrat, Toxicity assessment of metal oxide nano-pollutants on tomato (Solanum lycopersicon): A study on growth dynamics and plant cell death, Environ. Pollut., 2018, 240, 802–816 CrossRef CAS PubMed.
- M. Ke, Q. Qu, W. Peijnenburg, X. Li, M. Zhang, Z. Zhang, T. Lu, X. Pan and H. Qian, Phytotoxic effects of silver nanoparticles and silver ions to Arabidopsis thaliana as revealed by analysis of molecular responses and of metabolic pathways, Sci. Total Environ., 2018, 644, 1070–1079 CrossRef CAS.
- P. M. G. Nair and I. M. Chung, Impact of copper oxide nanoparticles exposure on Arabidopsis thaliana growth, root system development, root lignificaion, and molecular level changes, Environ. Sci. Pollut. Res., 2014, 21, 12709–12722 CrossRef CAS PubMed.
- F. Yanık and F. Vardar, Oxidative stress response to aluminum oxide (Al2O3) nanoparticles in Triticum aestivum, Biologia, 2018, 73, 129–135 Search PubMed.
- C. Ma, H. Liu, H. Guo, C. Musante, S. H. Coskun, B. C. Nelson, J. C. White, B. Xing and O. P. Dhankher, Defense mechanisms and nutrient displacement in Arabidopsis thaliana upon exposure to CeO2 and In2O3 nanoparticles, Environ. Sci.: Nano, 2016, 3, 1369–1379 RSC.
- M. Rui, C. Ma, J. C. White, Y. Hao, Y. Wang, X. Tang, J. Yang, F. Jiang, A. Ali and Y. Rui, Metal oxide nanoparticles alter peanut (Arachis hypogaea L.) physiological response and reduce nutritional quality: a life cycle study, Environ. Sci.: Nano, 2018, 5, 2088–2102 RSC.
- D. Cui, P. Zhang, Y. Ma, X. He, Y. Li, J. Zhang, Y. Zhao and Z. Zhang, Effect of cerium oxide nanoparticles on asparagus lettuce cultured in an agar medium, Environ. Sci.: Nano, 2014, 1, 459–465 RSC.
- O. Bar-Ilan, C. C. Chuang, D. J. Schwahn, S. Yang, S. Joshi, J. A. Pedersen, R. J. Hamers, R. E. Peterson and W. Heideman, TiO2 nanoparticle exposure and illumination during zebrafish development: Mortality at parts per billion concentrations, Environ. Sci. Technol., 2013, 47, 4726–4733 CrossRef CAS PubMed.
- D. K. Tripathi, R. K. Mishra, S. Singh, S. Singh, V. P. Singh, P. K. Singh, D. K. Chauhan, S. M. Prasad, N. Dubey and A. C. Pandey, Nitric oxide ameliorates zinc oxide nanoparticles phytotoxicity in wheat seedlings: implication of the ascorbate-glutathione cycle, Front. Plant Sci., 2017, 8, 1 Search PubMed.
- J. Xu, X. Luo, Y. Wang and Y. Feng, Evaluation of zinc oxide nanoparticles on lettuce (Lactuca sativa L.) growth and soil bacterial community, Environ. Sci. Pollut. Res., 2018, 25, 6026–6035 CrossRef CAS PubMed.
- H. Xun, X. Ma, J. Chen, Z. Yang, B. Liu, X. Gao, G. Li, J. Yu, L. Wang and J. Pang, Zinc oxide nanoparticle exposure triggers different gene expression patterns in maize shoots and roots, Environ. Pollut., 2017, 229, 479–488 CrossRef CAS PubMed.
- D. Lin and B. Xing, Phytotoxicity of nanoparticles: inhibition of seed germination and root growth, Environ. Pollut., 2007, 150, 243–250 CrossRef CAS PubMed.
- S. W. Ryter, H. P. Kim, A. Hoetzel, J. W. Park, K. Nakahira, X. Wang and A. M. Choi, Mechanisms of cell death in oxidative stress, Antioxid. Redox Signaling, 2007, 9, 49–89 CrossRef CAS PubMed.
- L. Zhao, J. A. Hernandez-Viezcas, J. R. Peralta-Videa, S. Bandyopadhyay, B. Peng, B. Munoz, A. A. Keller and J. L. Gardea-Torresdey, ZnO nanoparticle fate in soil and zinc bioaccumulation in corn plants (Zea mays) influenced by alginate, Environ. Sci.: Processes Impacts, 2013, 15, 260–266 RSC.
- J. Chen, X. Liu, C. Wang, S.-S. Yin, X.-L. Li, W.-J. Hu, M. Simon, Z.-J. Shen, Q. Xiao and C.-C. Chu, Nitric oxide ameliorates zinc oxide nanoparticles-induced phytotoxicity in rice seedlings, J. Hazard. Mater., 2015, 297, 173–182 CrossRef CAS PubMed.
- C. C. Monteiro, R. F. Carvalho, P. L. Gratão, G. Carvalho, T. Tezotto, L. O. Medici, L. E. Peres and R. A. Azevedo, Biochemical responses of the ethylene-insensitive Never ripe tomato mutant subjected to cadmium and sodium stresses, Environ. Exp. Bot., 2011, 71, 306–320 CrossRef CAS.
- M. B. Montero-Palmero, C. Ortega-Villasante, C. Escobar and L. E. Hernández, Are plant endogenous factors like ethylene modulators of the early oxidative stress induced by mercury?, Front. Environ. Sci., 2014, 2, 34 Search PubMed.
- Q. Chen, K. Wu, Z. Tang, Q. Guo, X. Guo and H. Wang, Exogenous ethylene enhanced the cadmium resistance and changed the alkaloid biosynthesis in Catharanthus roseus seedlings, Acta Physiol. Plant., 2017, 39, 267 CrossRef.
- B. Steffens, The role of ethylene and ROS in salinity, heavy metal, and flooding responses in rice, Front. Plant Sci., 2014, 5, 685 Search PubMed.
- M. Zhang, J. A. C. Smith, N. P. Harberd and C. Jiang, The regulatory roles of ethylene and reactive oxygen species (ROS) in plant salt stress responses, Plant Mol. Biol., 2016, 91, 651–659 CrossRef CAS PubMed.
- G.-q. Chen, L. Ren, J. Zhang, B. M. Reed, D. Zhang and X.-h. Shen, Cryopreservation affects ROS-induced oxidative stress and antioxidant response in Arabidopsis seedlings, Cryobiology, 2015, 70, 38–47 CrossRef CAS PubMed.
- S. Cao, S. Jiang and R. Zhang, Evidence for a role of Ethylene-Insensitive 2 gene in the regulation of the oxidative stress response in Arabidopsis, Acta Physiol. Plant., 2006, 28, 417–425 CrossRef CAS.
- K. Overmyer, H. Tuominen, R. Kettunen, C. Betz, C. Langebartels, H. Sandermann and J. Kangasjärvi, Ozone-sensitive Arabidopsis rcd1 mutant reveals opposite roles for ethylene and jasmonate signaling pathways in regulating superoxide-dependent cell death, Plant Cell, 2000, 12, 1849–1862 CrossRef CAS.
- H. Kende, Ethylene biosynthesis, Annu. Rev. Plant Biol., 1993, 44, 283–307 CrossRef CAS.
- K. Schellingen, D. Van Der Straeten, F. Vandenbussche, E. Prinsen, T. Remans, J. Vangronsveld and A. Cuypers, Cadmium-induced ethylene production and responses in Arabidopsis thaliana rely on ACS2 and ACS6 gene expression, BMC Plant Biol., 2014, 14, 214 CrossRef PubMed.
- M. I. R. Khan, F. Nazir, M. Asgher, T. S. Per and N. A. Khan, Selenium and sulfur influence ethylene formation and alleviate cadmium-induced oxidative stress by improving proline and glutathione production in wheat, J. Plant Physiol., 2015, 173, 9–18 CrossRef CAS PubMed.
- P. Sun, Q.-Y. Tian, J. Chen and W.-H. Zhang, Aluminium-induced inhibition of root elongation in Arabidopsis is mediated by ethylene and auxin, J. Exp. Bot., 2009, 61, 347–356 CrossRef PubMed.
- K. Schellingen, D. Van Der Straeten, T. Remans, C. Loix, J. Vangronsveld and A. Cuypers, Ethylene biosynthesis is involved in the early oxidative challenge induced by moderate Cd exposure in Arabidopsis thaliana, Environ. Exp. Bot., 2015, 117, 1–11 CrossRef CAS.
- I. Ali, M. Jan, A. Wakeel, A. Azizullah, B. Liu, F. Islam, A. Ali, M. Daud, Y. Liu and Y. Gan, Biochemical responses and ultrastructural changes in ethylene insensitive mutants of Arabidopsis thialiana subjected to bisphenol A exposure, Ecotoxicol. Environ. Saf., 2017, 144, 62–71 CrossRef CAS PubMed.
- A. Masood, N. Iqbal and N. A. Khan, Role of ethylene in alleviation of cadmium-induced photosynthetic capacity inhibition by sulphur in mustard, Plant, Cell Environ., 2012, 35, 524–533 CrossRef CAS PubMed.
- J. M. Watkins, P. J. Hechler and G. K. Muday, Ethylene-induced flavonol accumulation in guard cells suppresses reactive oxygen species and moderates stomatal aperture, Plant Physiol., 2014, 164, 1707–1717 CrossRef CAS PubMed.
- M. Cui, Y. Lin, Y. Zu, T. Efferth, D. Li and Z. Tang, Ethylene increases accumulation of compatible solutes and decreases oxidative stress to improve plant tolerance to water stress in Arabidopsis, J. Plant Biol., 2015, 58, 193–201 CrossRef CAS.
- R. Desikan, K. Last, R. Harrett-Williams, C. Tagliavia, K. Harter, R. Hooley, J. T. Hancock and S. J. Neill, Ethylene-induced stomatal closure in Arabidopsis occurs via AtrbohF-mediated hydrogen peroxide synthesis, Plant J., 2006, 47, 907–916 CrossRef CAS PubMed.
- A. Wakeel, I. Ali, S. Upreti, A. Ullah, B. Liu, A. R. Khan, L. Huang, M. Wu and Y. Gan, Ethylene mediates dichromate induced inhibition of primary root growth by altering AUX1 expression and auxin accumulation in Arabidopsis thaliana, Plant, Cell Environ., 2018, 1453–1467 CrossRef CAS PubMed.
- B. Liu, C. Hua, G. Song, M. Wu, R. Cui, A. Zhang, Y. Liu, L. Huang, A. Yan and I. Ali, The SPATULA transcription factor regulates seed oil content by controlling seed specific genes in Arabidopsis thaliana, Plant Growth Regul., 2017, 82, 111–121 CrossRef CAS.
- L. Sun, A. Zhang, Z. Zhou, Y. Zhao, A. Yan, S. Bao, H. Yu and Y. Gan, GLABROUS INFLORESCENCE STEMS3 (GIS3) regulates trichome initiation and development in Arabidopsis, New Phytol., 2015, 206, 220–230 CrossRef CAS PubMed.
- Z. Zhou, L. Sun, Y. Zhao, L. An, A. Yan, X. Meng and Y. Gan, Zinc Finger Protein 6 (ZFP6) regulates trichome initiation by integrating gibberellin and cytokinin signaling in Arabidopsis thaliana, New Phytol., 2013, 198, 699–708 CrossRef CAS PubMed.
- L. An, Z. Zhou, L. Sun, A. Yan, W. Xi, N. Yu, W. Cai, X. Chen, H. Yu and J. Schiefelbein, A zinc finger protein gene ZFP5 integrates phytohormone signaling to control root hair development in Arabidopsis, Plant J., 2012, 72, 474–490 CrossRef CAS PubMed.
- Y. Liu, D. Liu, R. Hu, C. Hua, I. Ali, A. Zhang, B. Liu, M. Wu, L. Huang and Y. Gan, AtGIS, a C2H2 zinc-finger transcription factor from Arabidopsis regulates glandular
trichome development through GA signaling in tobacco, Biochem. Biophys. Res. Commun., 2017, 483, 209–215 CrossRef CAS PubMed.
- H. K. Lichtenthaler, [34] Chlorophylls and carotenoids: pigments of photosynthetic biomembranes, Methods Enzymol., 1987, 148, 350–382 CrossRef CAS.
-
R. L. Whistler and R. L. Whistler, Methods in carbohydrate chemistry, 1962 Search PubMed.
- W. Zhang, F. Zhang, R. Raziuddin, H. Gong, Z. Yang, L. Lu, Q. Ye and W. Zhou, Effects of 5-aminolevulinic acid on oilseed rape seedling growth under herbicide toxicity stress, J. Plant Growth Regul., 2008, 27, 159–169 CrossRef CAS.
- W. Zhou and M. Leul, Uniconazole-induced tolerance of rape plants to heat stress in relation to changes in hormonal levels, enzyme activities and lipid peroxidation, Plant Growth Regul., 1999, 27, 99–104 CrossRef CAS.
- G. J. Ahammed, B.-B. He, X.-J. Qian, Y.-H. Zhou, K. Shi, J. Zhou, J.-Q. Yu and X.-J. Xia, 24-Epibrassinolide alleviates organic pollutants-retarded root elongation by promoting redox homeostasis and secondary metabolism in Cucumis sativus L, Environ. Pollut., 2017, 229, 922–931 CrossRef CAS PubMed.
- H. Aebi, [13] Catalase in vitro, Methods Enzymol., 1984, 105, 121–126 CAS.
- R. L. Heath and L. Packer, Photoperoxidation in isolated chloroplasts: I. Kinetics and stoichiometry of fatty acid peroxidation, Arch. Biochem. Biophys., 1968, 125, 189–198 CrossRef CAS PubMed.
- H. Thordal-Christensen, Z. Zhang, Y. Wei and D. B. Collinge, Subcellular localization of H2O2 in plants. H2O2 accumulation in papillae and hypersensitive response during the barley—powdery mildew interaction, Plant J., 1997, 11, 1187–1194 CrossRef CAS.
- Y. Gan, S. Filleur, A. Rahman, S. Gotensparre and B. G. Forde, Nutritional regulation of ANR1 and other root-expressed MADS-box genes in Arabidopsis thaliana, Planta, 2005, 222, 730 CrossRef CAS PubMed.
- A. M. E. Badawy, T. P. Luxton, R. G. Silva, K. G. Scheckel, M. T. Suidan and T. M. Tolaymat, Impact of environmental conditions (pH, ionic strength, and electrolyte type) on the surface charge and aggregation of silver nanoparticles suspensions, Environ. Sci. Technol., 2010, 44, 1260–1266 CrossRef PubMed.
- S.-W. Bian, I. A. Mudunkotuwa, T. Rupasinghe and V. H. Grassian, Aggregation and dissolution of 4 nm ZnO nanoparticles in aqueous environments: influence of pH, ionic strength, size, and adsorption of humic acid, Langmuir, 2011, 27, 6059–6068 CrossRef CAS PubMed.
- J. M. Unrine, B. P. Colman, A. J. Bone, A. P. Gondikas and C. W. Matson, Biotic and abiotic interactions in aquatic microcosms determine fate and toxicity of Ag nanoparticles. Part 1. Aggregation and dissolution, Environ. Sci. Technol., 2012, 46, 6915–6924 CrossRef CAS PubMed.
- A. Mukherjee, J. R. Peralta-Videa, S. Bandyopadhyay, C. M. Rico, L. Zhao and J. L. Gardea-Torresdey, Physiological effects of nanoparticulate ZnO in green peas (Pisum sativum L.) cultivated in soil, Metallomics, 2014, 6, 132–138 RSC.
- K. Luyts, D. Napierska, B. Nemery and P. H. Hoet, How physico-chemical characteristics of nanoparticles cause their toxicity: complex and unresolved interrelations, Environ. Sci.: Processes Impacts, 2013, 15, 23–38 RSC.
- J. Yang, W. Cao and Y. Rui, Interactions between nanoparticles and plants: phytotoxicity and defense mechanisms, J. Plant Interact., 2017, 12, 158–169 CrossRef CAS.
- Z. Yang, J. Chen, R. Dou, X. Gao, C. Mao and L. Wang, Assessment of the phytotoxicity of metal oxide nanoparticles on two crop plants, maize (Zea mays L.) and rice (Oryza sativa L.), Int. J. Environ. Res. Public Health, 2015, 12, 15100–15109 CrossRef CAS PubMed.
- J. de Almeida Engler, L. De Veylder, R. De Groodt, S. Rombauts, V. Boudolf, B. De Meyer, A. Hemerly, P. Ferreira, T. Beeckman and M. Karimi, Systematic analysis of cell-cycle gene expression during
Arabidopsis development, Plant J., 2009, 59, 645–660 CrossRef PubMed.
- A. Skirycz, H. Claeys, S. De Bodt, A. Oikawa, S. Shinoda, M. Andriankaja, K. Maleux, N. B. Eloy, F. Coppens and S.-D. Yoo, Pause-and-stop: the effects of osmotic stress on cell proliferation during early leaf development in Arabidopsis and a role for ethylene signaling in cell cycle arrest, Plant Cell, 2011, 23, 1876–1888 CrossRef CAS PubMed.
- L. Zhao, P. Wang, H. Hou, H. Zhang, Y. Wang, S. Yan, Y. Huang, H. Li, J. Tan and A. Hu, Transcriptional regulation of cell cycle genes in response to abiotic stresses correlates with dynamic changes in histone modifications in maize, PLoS One, 2014, 9, e106070 CrossRef PubMed.
- J. Li, H. Xu, W. Liu, X. Zhang and Y.-T. Lu, Ethylene inhibits root elongation during alkaline stress through AUX1 and associated changes in auxin accumulation, Plant Physiol., 2015, 00523.02015 Search PubMed.
- A. Wakeel, I. Ali, M. Wu, A. R. Kkan, M. Jan, A. Ali, Y. Liu, S. Ge, J. Wu and Y. Gan, Ethylene mediates dichromate induced oxidative stress and regulation of the enzymatic antioxidant system-related transcriptome in Arabidopsis thaliana, Environ. Exp. Bot., 2018 Search PubMed , in press.
- M. Weber, A. Trampczynska and S. Clemens, Comparative transcriptome analysis of toxic metal responses in Arabidopsis thaliana and the Cd2+-hypertolerant facultative metallophyte Arabidopsis halleri, Plant, Cell Environ., 2006, 29, 950–963 CrossRef CAS.
- M. Jakubowicz, H. Gałgańska, W. Nowak and J. Sadowski, Exogenously induced expression of ethylene biosynthesis, ethylene perception, phospholipase D, and Rboh-oxidase genes in broccoli seedlings, J. Exp. Bot., 2010, 61, 3475–3491 CrossRef CAS PubMed.
- X. Qu, B. P. Hall, Z. Gao and G. E. Schaller, A strong constitutive ethylene-response phenotype conferred on Arabidopsis plants containing null mutations in the ethylene receptors ETR1 and ERS1, BMC Plant Biol., 2007, 7, 3 CrossRef PubMed.
- R. Amooaghaie, M. Norouzi and M. Saeri, Impact of zinc and zinc oxide nanoparticles on the physiological and biochemical processes in tomato and wheat, Botany, 2016, 95, 441–455 CrossRef.
- L. Zhao, J. R. Peralta-Videa, C. M. Rico, J. A. Hernandez-Viezcas, Y. Sun, G. Niu, A. Servin, J. E. Nunez, M. Duarte-Gardea and J. L. Gardea-Torresdey, CeO2 and ZnO nanoparticles change the nutritional qualities of cucumber (Cucumis sativus), J. Agric. Food Chem., 2014, 62, 2752–2759 CrossRef CAS PubMed.
- X. Li, M. Ke, M. Zhang, W. Peijnenburg, X. Fan, J. Xu, Z. Zhang, T. Lu, Z. Fu and H. Qian, The interactive effects of diclofop-methyl and silver nanoparticles on Arabidopsis thaliana: growth, photosynthesis and antioxidant system, Environ. Pollut., 2018, 232, 212–219 CrossRef CAS PubMed.
- H. Qian, X. Peng, X. Han, J. Ren, L. Sun and Z. Fu, Comparison of the toxicity of silver nanoparticles and silver ions on the growth of terrestrial plant model Arabidopsis thaliana, J. Environ. Sci., 2013, 25, 1947–1956 CrossRef CAS.
- R. Szymańska, K. Kołodziej, I. Ślesak, P. Zimak-Piekarczyk, A. Orzechowska, M. Gabruk, A. Żądło, I. Habina, W. Knap and K. Burda, Titanium dioxide nanoparticles (100–1000 mg/l) can affect vitamin E response in Arabidopsis thaliana, Environ. Pollut., 2016, 213, 957–965 CrossRef PubMed.
- C. Ma, S. Chhikara, B. Xing, C. Musante, J. C. White and O. P. Dhankher, Physiological and molecular response of Arabidopsis thaliana (L.) to nanoparticle cerium and indium oxide exposure, ACS Sustainable Chem. Eng., 2013, 1, 768–778 CrossRef CAS.
- S. Hörtensteiner, Chlorophyll degradation during senescence, Annu. Rev. Plant Biol., 2006, 57, 55–77 CrossRef PubMed.
- F. B. Abeles, W. L. Hershberger and L. J. Dunn, Hormonal regulation, and intracellular localization of a 33-kD cationic peroxidase in excised cucumber cotyledons, Plant Physiol., 1989, 89, 664–668 CrossRef CAS PubMed.
- S. Munné-Bosch, J. Penuelas, D. Asensio and J. Llusia, Airborne ethylene may alter antioxidant protection and reduce tolerance of holm oak to heat and drought stress, Plant Physiol., 2004, 136, 2937–2947 CrossRef PubMed.
- K. Apel and H. Hirt, Reactive oxygen species: metabolism, oxidative stress, and signal transduction, Annu. Rev. Plant Biol., 2004, 55, 373–399 CrossRef CAS PubMed.
- K. Overmyer, M. Brosché and J. Kangasjärvi, Reactive oxygen species and hormonal control of cell death, Trends Plant Sci., 2003, 8, 335–342 CrossRef CAS PubMed.
- P. Diaz-Vivancos, G. Barba-Espín and J. A. Hernández, Elucidating hormonal/ROS networks during seed germination: insights and perspectives, Plant Cell Rep., 2013, 32, 1491–1502 CrossRef CAS PubMed.
- B. Steffens, The role of ethylene and ROS in salinity, heavy metal, and flooding responses in rice, Front. Plant Sci., 2014, 5, 685 Search PubMed.
- V. Flors, M. Paradís, J. García-Andrade, M. Cerezo, C. González-Bosch and P. García-Agustín, A tolerant behavior in salt-sensitive tomato plants can be mimicked by chemical stimuli, Plant Signaling Behav., 2007, 2, 50–57 CrossRef.
- D. M. Hodges and C. F. Forney, The effects of ethylene, depressed oxygen and elevated carbon dioxide on antioxidant profiles of senescing spinach leaves, J. Exp. Bot., 2000, 51, 645–655 CrossRef CAS PubMed.
- I. Sylvestre, Effect of the ethylene rise on the peroxidation of membrane lipids during the senescence of cut carnations, Plant Physiol. Biochem., 1989, 27, 407–413 CAS.
- N. Ghosh, M. Adak, P. Ghosh, S. Gupta, D. S. Gupta and C. Mandal, Differential responses of two rice varieties to salt stress, Plant Biotechnol. Rep., 2011, 5, 89–103 CrossRef.
- Y. Zhang, Q. He, S. Zhao, L. Huang and L. Hao, Arabidopsis ein2-1 and npr1-1 response to Al stress, Bull. Environ. Contam. Toxicol., 2014, 93, 78–83 CrossRef CAS PubMed.
- P. Jaspers and J. Kangasjärvi, Reactive oxygen species in abiotic stress signaling, Physiol. Plant., 2010, 138, 405–413 CrossRef CAS PubMed.
- V. D. Petrov and F. Van Breusegem, Hydrogen peroxide—a central hub for information flow in plant cells, AoB Plants, 2012, 2012, pls014 CrossRef PubMed.
- I. Saidi, Y. Chtourou and W. Djebali, Selenium alleviates cadmium toxicity by preventing oxidative stress in sunflower (Helianthus annuus) seedlings, J. Plant Physiol., 2014, 171, 85–91 CrossRef CAS PubMed.
- M. P. Benavides, S. M. Gallego and M. L. Tomaro, Cadmium toxicity in plants, Braz. J. Plant Physiol., 2005, 17, 21–34 CrossRef CAS.
- Z. S. Zhou, S. J. Wang and Z. M. Yang, Biological detection and analysis of mercury toxicity to alfalfa (Medicago sativa) plants, Chemosphere, 2008, 70, 1500–1509 CrossRef CAS PubMed.
- F. K. Choudhury, R. M. Rivero, E. Blumwald and R. Mittler, Reactive oxygen species, abiotic stress and stress combination, Plant J., 2017, 90, 856–867 CrossRef CAS PubMed.
Footnote |
† Electronic supplementary information (ESI) available. See DOI: 10.1039/c8en00971f |
|
This journal is © The Royal Society of Chemistry 2019 |