Role of organic components in regulating denitrification in the coastal water of Daya Bay, southern China
Received
5th December 2018
, Accepted 5th April 2019
First published on 8th April 2019
Abstract
Both dissolved and particulate organic materials have been proposed to be important factors in regulating heterotrophic denitrification in various aquatic environments. However, the specific pathways and mechanisms remain elusive. In this study, water column samples were collected from Daya Bay, southern China, to examine the relationships between potential denitrification and different organic components in the water column. Bulk dissolved organic carbon (DOC) was categorized into three major components including terrigenous fluorescent (tFDOC), autochthonous fluorescent (bFDOC) and non-fluorescent (nFDOC) fractions, while the bulk particulate organic carbon (POC) was divided into terrigenous (tPOC) and autochthonous (bPOC) fractions based on an isotope mixing model. Potential denitrification derived from in situ incubation experiments under anoxic conditions was evident (ranging from 6 to 107 nmol N2 per L per h) and varied markedly among stations. When normalized to nitrate concentration, the denitrification rate (NDR) followed a positive trend with either the concentration or proportion of tFDOC, and a negative trend with the proportion of nFDOC, suggesting tFDOC was potentially favorable while nFDOC was unfavorable for denitrifying degradation. In comparison, the NDR showed a significant positive correlation with the proportion of bPOC in the bulk POC (p = 0.01), with a predictive power of >70%, indicating that the composition of POC has a substantial impact on potential denitrification. Furthermore, if both bPOC and suspended particulate matter (SPM) were considered as variables concurrently, the variability of NDR can be better predicted with a predictive power as high as 80%. Therefore, denitrifiers may preferentially utilize fresher and labile autochthonous POC instead of DOC especially in coastal waters where particles/colloids are abundant. Our results thus provide new insights for a better understanding of denitrification mechanisms in water columns and the importance of both suspended particles and POC components in regulating denitrification, especially in turbid and productive coastal environments.
Environmental significance
Microbial denitrification is one of the major nitrogen (N) removal processes in various ecosystems. In coastal areas where increased anthropogenic loading of N is becoming a more and more serious environmental issue, denitrification plays a key role not only in regulating the N reservoir but also in relieving environmental eutrophication. For most natural heterotrophic denitrification, both dissolved and particulate organic matter are recognized to be important controllers, whereas the relative importance of these two organic pools has not been well studied. With the use of several state-of-the-art fluorescence and isotope tools, the relationships between water column denitrification and different organic fractions in the coastal China bay are explored in this study. The results reveal a more dominant role of particulate organic carbon (POC) in regulating potential denitrifying activity than that of dissolved organic carbon. They also demonstrate that the combination of marine autochthonous POC and suspended particulate matter contents will well predict the variation of denitrification (as high as 80% predictive power). These results shed light on the new understanding of denitrification pathways and mechanisms in coastal aquatic environments.
|
Introduction
Microbial denitrification, a step-wise anaerobic reduction converting nitrate (NO3−) to dinitrogen gas (N2) via intermediates, including nitrite, nitric oxide and nitrous oxide,1 is widespread among ecological habitats involved in nitrogen (N) transformation and has been recognized as a major sink of global N reservoir.2,3 Both NO3− availability and organic matter supply are considered as important environmental factors controlling denitrification,4,5 and the organic matter usually becomes limiting when the ambient NO3− is sufficient.6,7 During the past few decades, the potential denitrifying capacity has greatly enhanced because of the increased anthropogenic loading of N in aquatic ecosystems.8 As a result, organic matter plays an even more important role in water column denitrification.9 Thus, knowledge of the role of different organic matter components and phases and their influences on denitrification is indispensable to a better understanding of denitrification mechanisms and the coupled biogeochemical processes between carbon (C) and N in a water column, and the improvement of ecological management.
In general, organic matter affects denitrification mainly through both direct and indirect pathways. In the direct pathway, organic matter provides the electron donor for nitrate reduction and drives the denitrification.9,10 In addition, the input of organic matter to the environment facilitates oxygen consumption by aerobic respiration and creates suboxic (or anoxic) conditions conducive to denitrification.2,3 Indeed, a positive relationship between the organic carbon content and denitrification rate is commonly observed in aquatic environments. For example, recent studies in the oxygen-depleted waters of the Arabian Sea11 and Baltic Sea12 have demonstrated that treatment with bulk particulate organic matter (POM) and/or dissolved organic matter (DOM) would significantly enhance the incubated denitrifying activities, indicating the importance of organic matter to marine N budgets. Besides quantity, the quality of organic matter has also been recognized to play a critical role in regulating denitrification. A most recent study in coastal waters showed that autogenic POM from marine algal production could stimulate the denitrification rate more greatly than the POM from terrestrial sources.13 Even though DOM and POM both act as organic carbon sources to fuel environmental denitrification, the relative importance between these two phases is not clear. Little is known about the interrelation between denitrification and the composition of organic matter in marine environments although a few pioneering studies in land-based ecosystems have shown the coupled effects of DOM and POM on denitrification and the fact that the quantity and quality of DOM are related to POM.14,15
Particle-associated denitrification as the N removal pathway in marine waters has received increasing attention over the past years. A model prediction even proposed that anaerobic N metabolism within particles would outweigh the water column denitrification rates on the global scale.16 O2-depleted microenvironments inside particles may create suitable suboxic/anoxic centers for anaerobic respiration.17 From laboratory simulated incubations, it has been demonstrated that cyanobacterial aggregates18 and diatom aggregates19 produced in natural seawater can be hotspots for denitrifying activities. Moreover, in situ observations of chemical tracers in pelagic waters also provided hints for the occurrence of denitrification in sinking particles.20 However, it is still unclear whether particle-associated denitrification can be significant in coastal areas, where particulate matter loading is usually high.
Daya Bay is one of the largest bays on the coast of southern China. The average concentration of dissolved inorganic nitrogen (DIN) across the bay was usually lower than 5 μmol L−1 due to the lack of major river inputs.21 As a result, organic matter in the bay was mostly derived from autochthonous sources.22,23 Over the past 30 years, however, rapid economic development and intensive human activities have led to the release of excess DIN and allochthonous organic matter into the bay,24,25 causing a major impact on the ecological environment.21 In addition, a previous study has confirmed the presence of denitrification in the sediments of Daya Bay.26 Although the water column is well oxygenated throughout the year,21 the dynamic hydrological settings lead to a tight pelagic–benthic coupling in the bay (e.g., sediment resuspension),27 probably allowing the existence of potential denitrification within the water column. From another perspective, the natural oxygenated bay water can inhibit the appearance of reducers such as sulfides, reduced manganese or iron, and methane, which are usually detected in anoxic zones of marine systems and serve as alternative electron donors for lithotrophic or chemolithoautotrophic denitrification.12 Thus, natural reduced organic carbon (both DOC and POC) even in apparent oxygenated water columns can serve as electron donors and promote denitrification. However, little is known about the potential denitrification processes resulting from natural organic matter in oxygenated water columns in marine environments. Knowledge is needed regarding the quantitative relationship between denitrification and organic matter composition, and the impacts on the nitrogen cycle under increased DIN and organic matter input in coastal environments.
In this study, potential denitrifying activity in a water column was determined by incubation with 15N labeling under oxygen degassing treatment, and the source and composition of DOM and POM were characterized by fluorescence spectroscopy and stable isotope analysis, respectively, to quantify the relationship between the denitrification rate and the composition of DOM and POM from different sources. We further compare the relative importance of DOM and POM in regulating potential denitrification and elucidate the coupling effect of DOM, POM and suspended particulate matter (SPM) on coastal denitrification. Our hypothesis is that fresher POM plays a more dominant role than DOM and the reactive organic component in SPM is a key factor in facilitating water column denitrification in coastal environments.
Materials and methods
Geographic and hydrographic description
Daya Bay is a semi-enclosed bay adjacent to the northern South China Sea (23.52–24.83 °N and 113.50–114.83 °E) with an area of 600 km2 and an average water depth of about 10 m.28 It is surrounded by highly urbanized cities such as Shenzhen to the west and Huizhou to the northeast. Daya Bay is also an important aquaculture area with two largest coves, Aotou and Dapeng Cove, situated in the northwest and southwest of the bay, respectively (Fig. 1). Most of the bay water originates from the South China Sea with several small rivers discharging into the western part seasonally.21 Tidal current in Daya Bay is dominated by an irregular semidiurnal tide and generally forms an anticlockwise gyre in the bay.27 According to previous studies, warm water in summer supports high primary production23 and intense benthic denitrification26 in Daya Bay. A high precipitation induced large terrigenous riverine input also occurs in this season.29,30
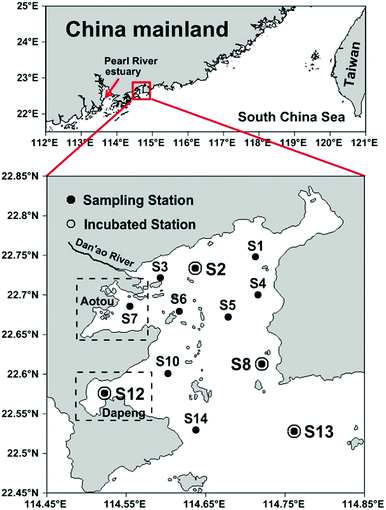 |
| Fig. 1 Sampling locations in Daya Bay. The upper panel shows the geographical environment around Daya Bay. In the lower panel, all sampling stations are shown as black dots, and the stations for incubation are marked as open circles. The rectangles with dashed lines represent the approximate areas of two aquaculture zones, Aotou Cove and Dapeng Cove. | |
Sample collection
A total of 12 stations were sampled during July 2015, among which Stations S2, S8, S12 and S13 were selected for denitrifying incubations (Fig. 1). Seawater samples were collected in 5 L GO-FLO bottles from the surface and bottom layers. Water temperature and salinity were recorded in situ using a YSI 6600 multi-probe sensor (Yellow Springs Instrument Co., USA). In addition, DIN, dissolved oxygen (DO), chlorophyll a (Chl-a), DOM, POM and SPM were concurrently analyzed.
Analysis of DIN, Chl-a and DO
For the measurements of Chl-a and DIN, including nitrate [NO3−], nitrite [NO2−], and ammonium [NH4+], water samples were filtered through Whatman GF/F membranes and frozen immediately at −20 °C in the dark. Concentrations of NO3−, NO2− and NH4+ were determined using a Lachat QuickChem 8500 autoanalyzer (Lachat Instruments, Loveland, CO, USA) using the standard colorimetric methods.31Chl-a concentration was measured by fluorescence using a Turner fluorometer after extracting with 90% acetone.32 Samples for DO measurement were directly transferred into a 120 mL glass bottle on deck and then DO was determined using the standard Winkler titration method immediately.31
DOM measurement and characterization
DOM samples were collected in 60 mL acid-cleaned (1 mol L−1 HCl for 24 h) and pre-combusted (450 °C for 5 h) amber borosilicate glass vials and kept in the dark at −20 °C until analysis.33 Dissolved organic carbon (DOC) was determined by the high-temperature combustion method using a total organic carbon analyzer (Shimadzu TOC-VCPH). DOC working standards were periodically measured as a sample to ensure data quality. Ultrapure water (18.2 MΩ) was used as a blank, and its DOC concentration was lower than 6 μmol C per L. The accuracy of DOC measurement was better than 2%.
To characterize the chemical composition of DOM, 3-D fluorescence spectra were measured using a Cary Eclipse Spectrofluorometer (Varian, Australia) with a 1 cm path-length quartz cuvette. The fluorescence spectra were scanned in 5 nm increments over the excitation (Ex) wavelength range of 200–450 nm and scanned in 2 nm increments over the emission (Em) wavelength range of 230–600 nm. Parallel Factor Analysis (PARAFAC) was then performed to resolve the excitation–emission matrix (EEM) via MATLAB software (MathWorks R2015b) and DOMFluor toolbox processing.34 Based on the EEM-PARAFAC analysis, three major fluorescent components were identified for the bulk DOM pool. Component 1 (C1) was characterized by its double Ex/Em peaks at 260/460 nm and 355/460 nm, corresponding to a typical terrigenous UV humic-like substance.34,35 Component 2 (C2) exhibited two peaks with Ex/Em wavelengths of 285/494 nm and 385/494 nm, similar to the characteristics of terrestrial UVA humic-like or fulvic moieties.34–36 Component 3 (C3) was characterized by a primary and secondary Ex/Em maximum at 240/390 nm and 310/390 nm, respectively, which are attributed to the autochthonous protein-like constituents.35,36 The PARAFAC results were verified using the consistent error distribution at the scanned Ex/Em wavelengths with the four- or five-component modeling.
Interestingly, there is a significant linear relationship between the intensity of each fluorescent component (in Quinine Sulfate Units, Q.S.U.) and DOC, i.e.,
| C1 (Q.S.U.) = 0.064 × DOC − 5.06 (r2 = 0.86, p < 0.0001, n = 26) | (1) |
| C2 (Q.S.U.) = 0.042 × DOC − 3.39 (r2 = 0.92, p < 0.0001, n = 26) | (2) |
| C3 (Q.S.U.) = 0.030 × DOC − 2.45 (r2 = 0.76, p < 0.0001, n = 26) | (3) |
The fitting relationships show that when the fluorescence intensity reaches zero, the non-fluorescent DOC corresponding to eqn (1), (2) and (3) was 79, 81 and 82 μmol L−1, respectively, with an average of 81 ± 1 μmol L−1. Therefore, fluorescent DOC can be estimated to be:
| 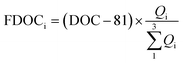 | (4) |
where FDOC
i and
Qi are the DOC concentration (in μmol C per L) and fluorescence intensity of each fluorescent component, respectively. Since C1 and C2 are both from terrestrial sources and C3 is derived from marine autochthonous sources, here the sum of FDOCs in C1 and C2 is defined as terrestrial fluorescent DOC (tFDOC), and the FDOC in C3 is defined as biological fluorescent DOC (bFDOC).
POM measurements and characterization
Suspended particulate matter was sampled by filtering 3 to 5 L of seawater through a pre-weighed and pre-combusted (400 °C, 4 h) 47 mm GF/F membrane. After rinsing with ultrapure water and drying at 60 °C, the membrane containing the particles was weighed to obtain the SPM content. One quarter of the membrane was used and acid-fumed to remove inorganic carbon for subsequent measurements of particulate organic carbon (POC) and its isotopic composition (δ13CPOC). Half of the remaining membrane was untreated to measure particulate nitrogen (PN) and its isotopic composition (δ15NPN). POC and PN contents as well as δ13CPOC and δ15NPN were determined using an isotopic ratio mass spectrometer (Deltaplus XP, Thermo Finnigan) coupled to an elemental analyzer (Flash EA 1112 series, Thermo Finnigan). The isotopic reference standards for C and N are PeeDee Belemnite and atmospheric N2, respectively. To monitor instrument performance and data quality, certified standard samples IAEA-C8 (δ13C = −18.3‰) and USGS40 (δ13C = −26.4‰) were used for δ13C, and IAEA-N2 (δ15N = 20.3‰) and IAEA-N3 (δ15N = 4.7‰) were used for δ15N. The detection limit of both POC and PN is 0.1 μmol, and the analytical precision of δ13C and δ15N is ±0.2‰.29
Sources of POM were distinguished by isotopic compositions to explore the effect of organic composition on denitrification. In the first approximation, POM in Daya Bay during the summer included three major sources, i.e., terrestrial, estuarine aquagenic, and marine autochthonous.29 A three end-member mixing model and their δ13CPOC and δ15NPN signatures were used to quantify the contribution of each component based on the following equations:
| fter + fest + fmar = 1 | (5) |
| δ13Cter × fter + δ13Cest × fest + δ13Cmar × fmar = δ13Cd | (6) |
| δ15Nter × fter + δ15Nest × fest + δ15Nmar × fmar = δ15Nd | (7) |
where
δ13C,
δ15N and
f refer to
δ13C
POC,
δ15N
PN and the proportion of each end-member, respectively. The subscripts ter, est and mar represent terrestrial, estuarine and marine sources, respectively, and
δ13C
d and
δ15N
d denote the determined isotopic composition. As chosen in Mu
et al. (2017),
29 the end-member
δ13C
POC and
δ15N
PN values were −24.5‰ and 0.7‰ for terrestrial, −29.0‰ and 15.0‰ for estuarine, and −16.9‰ and 9.0‰ for marine autochthonous POM, respectively. The POC content of each source was calculated as follows:
where POC
i and
fi denote the organic carbon content and proportion of each source in the bulk POC, respectively. To simplify, POM contents from both terrestrial and estuarine are combined as terrigenous, while marine autochthonous POM is
in situ produced biogenic POM. Accordingly, the POC from terrestrial and estuarine sources was defined as tPOC, and the marine autochthonous POC was defined as bPOC.
Measurements of potential denitrifying activity
Apparent denitrification rate (ADR).
Potential ADR was determined by 15N-labeled incubation under DO-degassed conditions at selected stations. Similar to the procedure described by Zeng et al. (2018),13 a batch of 9 mL bulk water from each depth was collected in a series of 12 mL Exetainer vials (Labco, UK) for time-series incubation. The water samples were purged with ultrapure helium gas (99.999%) for 10 min to remove background N2 and O2 before the 15N amendments, by which the anaerobic potential was activated. 15NO3− and 15NH4+ were added separately up to a final concentration of 1 μmol L−1 to synchronously measure denitrification and anaerobic ammonium oxidation (anammox), another important microbial N2 production route.37 Samples were incubated in the dark at in situ temperature. The time-series points were set at 0, 12, 24, 36, and 48 h. At each termination point, 40 μL of saturated HgCl2 solution was injected to prevent microbial activity. The incubation was conducted in duplicate for samples from each depth. Sample vials after incubation were kept in a water bath at room temperature (∼20 °C) to avoid N2 contamination from the atmosphere until analysis.
When back to the laboratory, the samples were sonicated at 40 °C for 40 min to equilibrate N2 between the headspace and the solution. Concentrations of 15N-labeled N2 species (29N2 and 30N2) were measured using a GasBench II system in combination with IRMS (Deltaplus XP, Thermo Finnigan) with a standard deviation of less than 0.1%.13
In the 15NO3− amended incubation, 15N-labelled N2 (i.e., 29N2 and 30N2) generally began to accumulate in a linear manner after 12 h with a consistent pattern of time-series, indicating that denitrification was mainly responsible for nitrogen removal.13,38 In contrast, the incubation with 15NH4+ showed no production of both 29N2 and 30N2, implying the absence of anammox. Therefore, the ADR with 15NO3−-amendment can be obtained as:
| 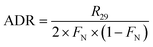 | (9) |
or
|  | (10) |
where
R29 and
R30 denote the production rate of
29N
2 and
30N
2, respectively, and
FN denotes
15N abundance in the bulk nitrate pool.
R29 (or
R30) was calculated
via linear regression of
29N
2 (or
30N
2) concentration against incubation time. The average ADR calculated using
R29 and
R30 was used in this study.
NO3−-normalized denitrification rate (NDR).
The response of the ADR to varying NO3− concentrations was evaluated using the surface sample from Station S2 and the bottom sample from Station S8. The ambient NO3− concentration of the surface water at Station S2 (5.73 μmol L−1) was about 4 times that of the bottom water at Station S8 (1.50 μmol L−1). Prior to incubation, the gradient of NO3− level in the vial was manipulated by the addition of 15NO3− up to a final concentration of 1 μmol L−1, 5 μmol L−1, and 10 μmol L−1, respectively. The measurement of the denitrification rate was carried out under the same conditions and using the same procedures as above.
The response patterns of ADR with NO3− concentration were different in the two samples. The ADR increased obviously with the increase of NO3− concentration in the bottom sample from Station S8, while the ADR declined slightly with the increase of NO3− concentration in the surface samples from Station S2 (Fig. 2). When the samples of the two layers were integrated, the combined data fit well the Michaelis–Menten equation, and the half-saturation constant (Km) was deduced to be 2.3 μmol L−1 (Fig. 2), which is rather similar to the typical values of natural denitrification.39,40 This means that when the ambient NO3− is as high as 6.5 μmol L−1, its effect on denitrifying activity is almost saturated. In order to better discuss the effect of organic matter on denitrification, a linear regression was used to normalize the ADR to a NO3− concentration of 6.5 μmol L−1 by assuming that the denitrification rate follows a first-order relationship when NO3− concentration is lower than Km.
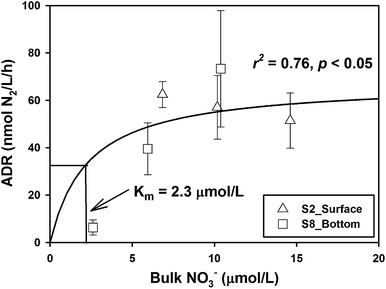 |
| Fig. 2 Response of apparent denitrification rate (ADR) with manipulated NO3− concentration at Stations S2 and S8. The solid line is fitted according to the Michaelis–Menten kinetics. Km represents the half-saturation constant of NO3− demand. | |
For samples from Stations S8 and S13, where the NO3− concentrations were below Km (i.e., 2.3 μmol L−1), the NDR was obtained as follows:
| 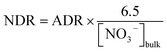 | (11) |
where [NO
3−]
bulk represents the bulk NO
3− concentration within the incubation system. Whereas for samples from Stations S2 and S12, where the NO
3− concentrations were close to 6.5 μmol L
−1, we ignored normalization and considered the measured ADR as saturated.
Data analysis and statistics
Correlation analyses were performed using the SigmaPlot 12.5 software package, and the results were accepted only when the residuals passed the Shapiro–Wilk normality test and the constant variance test. On the basis of the t-test, denitrification rates were adopted only if the slope value of linear regression was significantly greater than zero (p < 0.05). The detection limit for the denitrification rate was 0.68 nmol N2 per L per h. The Km value in the Michaelis–Menten equation was estimated from non-linear least squares fitting. One-way analysis of variance (ANOVA) with the Tukey's HSD test was applied to evaluate statistical differences of environmental parameters among stations using SPSS 16.0.
Results
Hydro-chemical parameters
During the sampling period in summer, the seawater temperature ranged from 24.5 °C to 31.1 °C, and increased monotonically from the outer bay to the inner bay (Fig. 3a). The lowest salinity (as low as 19.4) was recorded in the surface water of Station S3 near the river mouth. The salinity outside the bay was higher than 33.0, reflecting the impact of the shelf water from the northern South China Sea (Fig. 3b).21 Oxidized nitrogen (NO3− + NO2−, abbreviated as NOx−) accounted for the majority of DIN (∼65%). Both NOx− and NH4+ increased toward the inner bay, and the highest concentration was found in the surface water of Station S3 with the lowest salinity, showing the footprint of riverine input (Fig. 3c and d). The variation pattern of Chl-a was similar to that of the nitrogen species, except that the highest value of Chl-a was found in the surface water of Station S6 (Fig. 3e), where an intense algal bloom occurred.30 The SPM contents ranged from 0.70 to 14.16 mg L−1, with higher contents in the bottom water (Fig. 3f), showing a benthic SPM source from sediment resuspension. The water column was well oxygenated during sampling with DO concentrations ranging from 150 to 330 μmol L−1 for all stations. The average DO concentrations in surface and bottom water were 307 μmol L−1 and 191 μmol L−1, respectively.
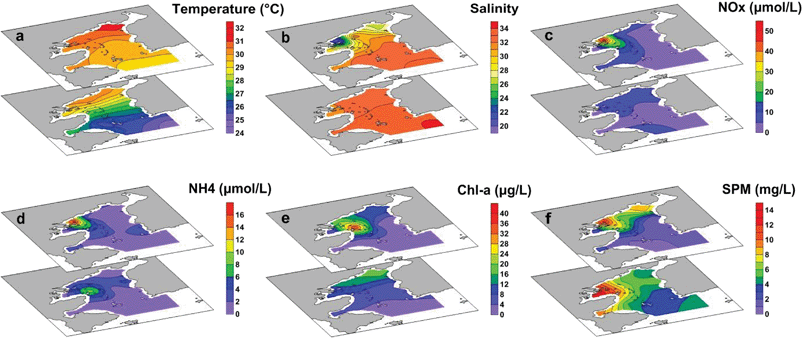 |
| Fig. 3 Spatial distributions of hydro-chemical parameters in the surface layer (the upper plots) and bottom layer (the lower plots) in Daya Bay: (a) temperature (°C); (b) salinity; (c) NO3− + NO2− (μmol L−1); (d) NH4+ (μmol L−1); (e) Chl-a (μg L−1); (f) SPM (mg L−1). | |
DOC and its sources
DOC accounted for the majority of the total organic carbon (TOC) pool in the bay, with concentrations ranging from 80 to 232 μmol L−1 (ave. 122 ± 39 μmol L−1), equivalent to 58–94% of TOC (i.e., TOC = DOC + POC). The distribution of DOC showed a sharp decrease from the inner bay to the outer bay, and the highest concentration was found in the surface water at Station S3, indicating the input from river discharge (Fig. 4a). The FDOC accounted for near-zero to 65% of the bulk DOC, of which tFDOC was the main fluorescent fraction, accounting for an average of 83 ± 7% and 24 ± 13% of the total FDOC and bulk DOC, respectively (Table 1). A significant negative correlation between tFDOC and salinity (p < 0.001) was observed (Fig. 5a), further demonstrating predominant terrestrial sources for tFDOC. The bFDOC concentration ranged from 0 to 17.6 μmol L−1, accounting for ∼0–13% of the bulk DOC (Table 1). A positive correlation between bFDOC and Chl-a (p = 0.001) was observed (Fig. 5b), attesting the marine autochthonous source of bFDOC. Both tFDOC and bFDOC followed the same distribution pattern as the bulk DOC in the bay, which was characterized by a decrease from the inner bay to the outer bay (Fig. 4b and c). Among the four stations where denitrification measurements were conducted, the bulk DOC, tFDOC and bFDOC concentrations were higher at Stations S2 and S12, while those at Stations S8 and S13 were lower (Table 1).
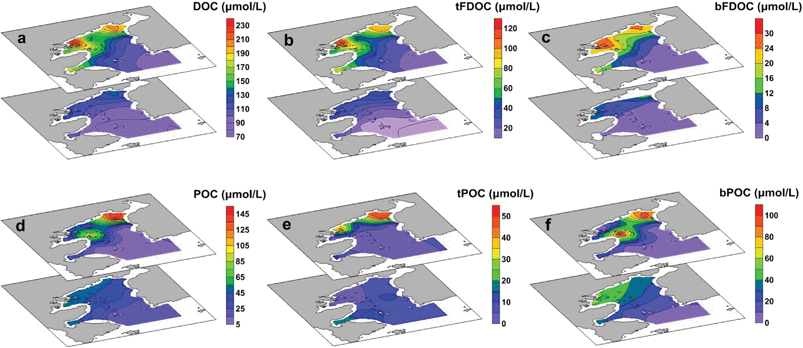 |
| Fig. 4 Spatial distributions of the bulk DOC and POC and their different components in the surface layer (the upper plots) and bottom layer (the lower plots) in Daya Bay: (a) bulk DOC (μmol C per L); (b) tFDOC from terrestrial sources (μmol C per L); (c) bFDOC from marine biogenic sources (μmol C per L); (d) bulk POC (μmol C per L); (e) tPOC from terrestrial sources (μmol C per L); (f) bPOC from marine biogenic sources (μmol C per L). | |
Table 1 Concentrations of DOC and its components, POC and its components, SPM, and nitrate, and potential denitrification rates in Daya Bay
Station |
Bottom depth (m) |
Sampling depth (m) |
DOCa (μmol C per L) |
POCb (μmol C per L) |
SPM (mg L−1) |
NO3− (μmol L−1) |
ADR (nmol N2 per L per h) |
NDRc (nmol N2 per L per h) |
Bulk |
tFDOC |
bFDOC |
Bulk |
tPOC |
bPOC |
The values in parentheses represent the proportion of the identified component in bulk DOC.
The values in parentheses represent the proportion of the identified component in bulk POC.
u.d. denotes under detection.
|
S2 |
7.0 |
0 |
136 |
39 (29%) |
18 (13%) |
36.28 |
9.39 (26%) |
26.89 (74%) |
5.58 |
5.73 |
62.4 ± 5.4 |
62.4 ± 5.4 |
6 |
113 |
27 (24%) |
6 (5%) |
47.47 |
3.10 (7%) |
44.37 (93%) |
6.21 |
7.16 |
107.1 ± 35.5 |
107.1 ± 35.5 |
S8 |
8.4 |
0 |
102 |
20 (20%) |
2 (2%) |
11.39 |
3.55 (31%) |
7.84 (69%) |
1.39 |
0.76 |
16.5 ± 5.3 |
57.2 ± 8.6 |
7.5 |
93 |
12 (12%) |
2 (2%) |
20.72 |
8.90 (43%) |
11.82 (57%) |
3.09 |
1.50 |
6.3 ± 1.2 |
15.7 ± 3.0 |
S12 |
8.0 |
0 |
174 |
76 (44%) |
16 (10%) |
34.12 |
10.07 (30%) |
24.05 (70%) |
2.25 |
8.26 |
u.d. |
u.d. |
7 |
104 |
20 (19%) |
3 (3%) |
51.86 |
19.84 (38%) |
32.02 (62%) |
9.29 |
4.03 |
32.1 ± 3.9 |
32.1 ± 3.9 |
S13 |
19.6 |
0 |
94 |
13 (13%) |
1 (1%) |
9.86 |
4.99 (51%) |
4.87 (49%) |
1.67 |
0.84 |
15.0 ± 9.2 |
50.1 ± 14.1 |
19 |
80 |
0 (0%) |
0 (0%) |
18.03 |
9.35 (52%) |
8.68 (48%) |
5.02 |
2.57 |
15.7 ± 0.7 |
27.7 ± 1.3 |
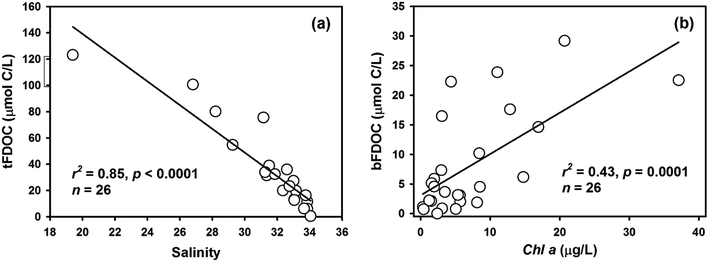 |
| Fig. 5 The relationship between tFDOC and salinity (a) and between bFDOC and Chl-a (b) in Daya Bay. | |
POC and its sources
The POC concentration varied greatly from 6.6 to 148.3 μmol L−1 (average 37.6 ± 32.3 μmol L−1) and decreased from the inner bay to the outer bay, which was similar to the DOC distribution (Fig. 4d). Unlike DOC, which was mainly from terrestrial sources, POC was mainly contributed by in situ biogenic sources with bPOC accounting for 68 ± 15% of the bulk POC pool (Table 1). It is worth noting that both the concentration and proportion of bPOC correlated fairly well with those of Chl-a (Fig. 6), indicating that bPOC was predominantly from algal production. The high proportion of bPOC was consistent with the earlier recognition that marine biological production was the major source of POM in Daya Bay.22 The spatial distribution of POM from different sources indicated that the contents of tPOC and bPOC in the inner bay were higher than those in the outer bay, and the tPOC and bPOC contents in surface water were generally lower than those of bottom water (Fig. 4e and f). The concentrations of bulk POC and bPOC were significantly different among the four incubation stations (p < 0.05). The bPOC concentrations at Stations S2 and S12 were, on average, 3.8 times higher than those at Stations S8 and S13, while the tPOC was less variable except for the higher concentrations observed at Station S12 (Table 1).
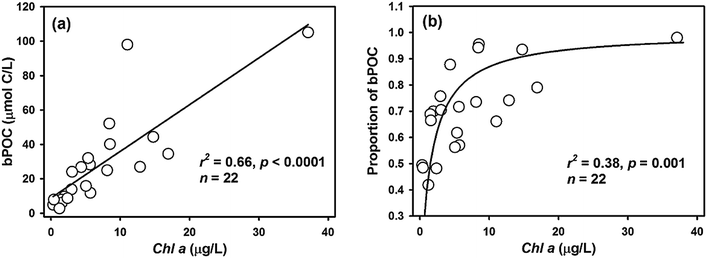 |
| Fig. 6 The relationship between concentration of bPOC and Chl-a (a) and between proportion of bPOC and Chl-a (b) in Daya Bay. | |
Variability of denitrifying activity
Except for the surface at Station S12, the ADRs at the four incubation stations ranged from 6.3 ± 1.2 to 107.1 ± 35.5 nmol N2 per L per h with an average of 36.4 nmol N2 per L per h (Table 1). These ADRs were at the upper end of the reported denitrification rates in typical marine suboxic waters,39–41 indicating an intense potential denitrification in the bay. The highest denitrifying activity was observed at Station S2, and its ADR was about 6 times higher than that at other stations. After normalizing to the NO3− concentration, the NDRs at Stations S2 and S12 were close to the ADRs, while the NDRs at Stations S8 and S13 were 2–3 times greater than the ADRs (Table 1). There was no significant correlation between the NDR and bulk DOC or bulk POC (p > 0.2), indicating that the quantity of organic matter alone could not explain the denitrifying variability in Daya Bay, and its control factors were more complicated.
Discussion
Effect of DOM from different sources on denitrification
When NO3− is abundant in the environment, the availability of organic matter usually becomes a limitation to denitrifying activity.6,7,42 In most heterotrophic bacteria, organic substrates are directly utilized in dissolved form through transmembrane transport.43 Thus, DOC is reasonably assumed to be the primary carbon source for denitrification. In a recent study at the oxic–anoxic interface in the Baltic Sea, it was proposed that DOC might be the main driver of water column denitrification.12 However, the bulk DOC concentration cannot adequately explain the potential denitrification changes in Daya Bay. In fact, the lack of positive stimulation of denitrification by DOC was sometimes observed in land-based aquatic ecosystems, where the ambient DOC loading was relatively high.44,45 According to the classic stoichiometry of the denitrification reaction,2 the amount of organic carbon required for complete nitrogen removal was estimated to be only 7.0 μmol C per L in Daya Bay, which is less than 10% of the bulk DOC. In addition, as reported for the anoxic Baltic Sea, the half-saturation constant for DOC demand of denitrification was only <1 μmol L−1, suggesting high affinity of heterotrophic denitrification for DOC.12 This means that the supply of DOC in our experiments was sufficient for denitrification, and the bulk DOC concentration might not be the primary factor limiting denitrification.
DOC quality, not just quantity, is increasingly recognized as an important factor in regulating denitrification. For example, laboratory incubations with substrate additions have shown that simple organic molecules such as acetate and glucose can greatly stimulate denitrifying activity in a wide range of habitats, including river sediments,5,46 hyporheic zone of land streams7 and typical marine oxygen-depleted waters.11,12 However, the composition of natural DOM is far more complex than the ones used in experiments. Based on fluorescence spectra and PARAFAC modeling, the DOM in our study area was divided into a fluorescent fraction and non-fluorescent fraction, of which the former includes terrigenous and marine autochthonous moieties. In general, organic matter from terrestrial sources is usually rich in lignin or humic substances and thus is more recalcitrant and energetically less favorable to break down compared with the moieties from algal autochthonous production.35,47 However, in our study, the NDR broadly positively correlated with the concentration or proportion of tFDOC although not significant (r = 0.64, p = 0.06) (Fig. 7a and b), which is much better than the relationship between the NDR and bFDOC (r = 0.44, p > 0.2). It seems that tFDOC may be more beneficial for denitrifying degradation than bFDOC in the bulk DOC pool. Similarly, previous studies also showed that typical terrestrial organic matter, especially aromatic, humic or fulvic substances, can maintain or even stimulate the denitrifying activity of certain bacterial strains (e.g., Nozawa and Maruyama, 1988; Pfenning and McMahon, 1996).46,48 Actually, the terrestrial DOM is not necessarily refractory. In a study from boreal lakes, it was revealed that the absolute amount of the labile fraction in a terrestrial DOC pool was even larger than that in an autochthonous algal DOC pool, and the former part fueled not only short-term but also long-term microbial carbon consumption in ecosystems.49
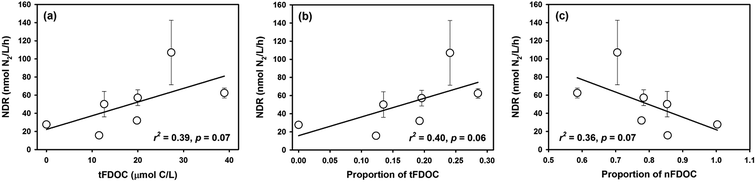 |
| Fig. 7 The relationship between NDR and DOM components from different sources in Daya Bay: (a) NDR versus tFDOC; (b) NDR versus proportion of tFDOC; (c) NDR versus proportion of nFDOC. | |
Our result was somewhat inconsistent with that of Barnes et al. (2012),42 whose study depicted a negative correlation between denitrification and the percentage of terrigenous quinone-type components, and a positive correlation between denitrification and the percentage of biogenic amino acids, in the sediments of Boulder Creek watershed. On the one hand, the inconsistency could be probably caused by the distinct environmental media between stream sediments and marine waters, in which denitrification rates were measured. On the other hand, different correlations between studies may also result from a difference in DOM properties. In Barnes et al.'s (2012) study,42 their sampling areas ranged from alpine to plains with relatively low vegetation coverage and the ecosystem was primitive. Unlike the Boulder Creek watershed, a variety of highly productive habitats exist along the coast of Daya Bay, especially mangroves and intertidal mudflats.21 Furthermore, a large amount of anthropogenic organic input has had a great impact on the Daya Bay ecosystem.25 It is well documented that the land use and vegetation cover markedly influence the quantity and quality of DOM delivered into the adjacent watersheds or aquatic systems.50 For example, the DOM originating from soils with higher organic share is recognized to be more labile for microbial degradation.51 In addition, the source and quality of DOM would have an impact on the patterns of microbial metabolism. It was proposed that humic DOC from forest headstreams generally supports bacterial growth instead of their respiration in freshwater systems.52 Therefore it is reasonable to speculate that the terrestrial DOM entering Daya Bay may be more degradable or favorable for microbial respiration than that entering the Boulder Creek watershed, thus favoring denitrification in Daya Bay. With regard to autochthonous components, they are mainly recognized as protein-like substances (or amino acids) and are generally considered to be bio-favorable. The lack of correlation between the NDR and bFDOC in Daya Bay can be attributed to the low concentration of biogenic components. For example, the bFDOC only accounts for a minor fraction (average 5%) of the bulk DOC in seawater samples from the four NDR stations (Table 1). Due to the deficiency of autochthonous DOM, its regulation on denitrifying activity was limited. It should be pointed out that photochemical processes can oxidize DOC to CO2 and consume O2 in aqueous environments.53 Thus, it is possible that the tFDOC follows these reactions and creates a low O2 condition to stimulate denitrifying activity instead of its degradation for denitrification. However, dissolved O2 concentrations in the water column during our cruise were generally higher than 150 μmol L−1 (refer to the Results section), which is far exceeding the typical O2 threshold of 2 μmol L−1 for denitrification.37 In other words, the photochemical oxygen consumption of tFDOC, if any, may only have little effect on enhancing denitrification potentials in Daya Bay. On the other hand, some other research studies even suggested that dissolved O2 at nanomolar levels suppresses denitrifying N2 production and the related gene expressions.54
In our study, a negative correlation, although not significant (r = −0.60, p = 0.07), between the NDR and the proportion of non-fluorescent DOC (nFDOC) was observed concurrently (Fig. 7c). It implies that nFDOC may be less degradable for denitrifying bacteria relative to tFDOC and bFDOC. Even though the chemical properties and biodegradability of the non-fluorescent DOM fraction remain poorly characterized, black carbon, produced from incomplete combustion of fossil fuel and biomass and primarily composed of condensed aromatics,55 is recognized as one of the most chemically recalcitrant fractions of organic carbon in aquatic environments.56 The riverine transport significantly contributes black carbon from terrestrial sources to the oceans.57,58 It has been shown that dissolved black carbon in aquatic systems is very susceptible to partial photooxidation and its fluorescence is greatly reduced under light exposure.59 Therefore, the partially photooxidized dissolved black carbon should probably account for the non-fluorescent DOM in our study area. However, dissolved black carbon in marine ecosystems usually represents a minor proportion of bulk DOC.60 In a recent study, it has been shown that dissolved black carbon in coastal waters of China only accounted for less than 5% of DOC pools.61 If this fraction is used for a first-order calculation, the maximum concentration of dissolved black carbon in Daya Bay is estimated to be 12 μmol C per L, which is far from explaining the identified nFDOC concentration of 81 μmol L−1. Although there are still many unknowns for the non-fluorescent DOM, our results show for the first time that non-fluorescent DOM has an impact on regulating denitrification in coastal environments.
Effect of POM from different sources on denitrification
A negative trend between the NDR and terrigenous POC (tPOC) concentration (r = −0.55, p = 0.10) and a positive trend between the NDR and autochthonous POC (bPOC) concentration (r = 0.63, p = 0.06) were observed (Fig. 8a and b), although both of them lack statistical significance. In comparison, there was a significant and positive correlation between the NDR and the bPOC fraction in the bulk POC (r = 0.86, p = 0.01) (Fig. 8c), similar to what was observed recently in the Beibu Gulf of southern China.13 These relationships suggest that marine autochthonous POM not only favors denitrification reactions, but also plays a dominant role in regulating denitrifying activity in coastal environments. Similar findings have been reported for other terrestrial ecosystems. For example, Dodla et al. (2008)62 observed in wetland soils that the measured denitrification rate increased linearly with increasing labile polysaccharide content, but was negatively correlated with the percentage of recalcitrant phenolic carbon in the total organic carbon pool. In addition, POM with low C/N ratio induced higher denitrifying activity in wetland and stream sediments,14,63 pointing to the effectiveness of labile POM. In contrast, terrigenous POM is usually a mixture of plant debris and degraded organic matter, characterized by high contents of lignin or cellulose components with a higher C/N ratio, and thus is more resistant to biodegradation47 and less effective in promoting denitrification.
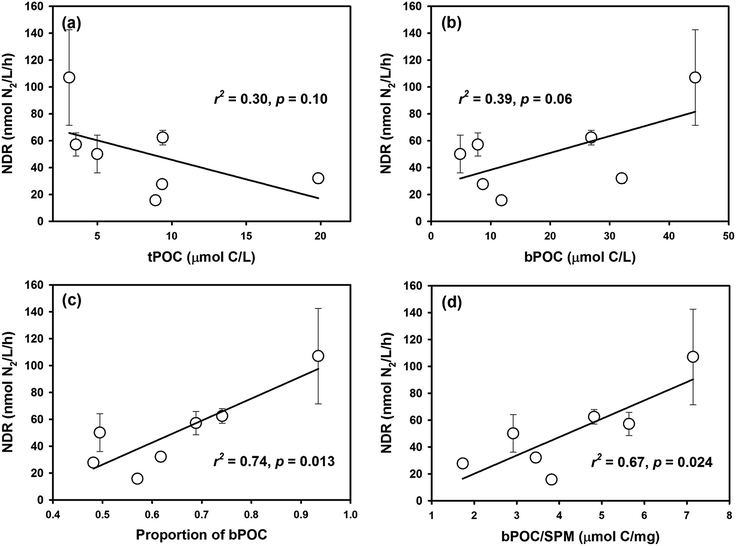 |
| Fig. 8 The relationship between NDR and POM components from different sources in Daya Bay: (a) NDR versus tPOC; (b) NDR versus bPOC; (c) NDR versus proportion of bPOC; (d) NDR versus bPOC/SPM ratio. | |
There are two pathways for POM to regulate denitrification in water columns. One is to directly affect the number of particle-associated denitrifiers (e.g., Liu et al., 2013),64 and the other is to affect the supply of organic matter for the growth of ambient free-living denitrifiers (e.g., Stelzer et al., 2015).14 In aquatic systems, bacteria preferentially adhere to suspended particles or aggregates,65 where suboxic or anoxic micro-sites usually exist and favor anaerobic respiration.17,18 It was proposed that SPM and aggregates in seawater could be hotspots for denitrification and may contribute significantly to global N removal.16,38,64 In order to better reveal the coupled effect of particulate matter on denitrifying activity, the bPOC concentration was normalized to SPM content to gauge the POM quality in terms of μmol C per mg particles. Compared with the relationship between the NDR and non-normalized bPOC, the significance of the positive correlation between the NDR and SPM-normalized bPOC (bPOC/SPM) was significantly improved, and the bPOC/SPM parameter can explain about 70% of the variability of the NDR (Fig. 8d). In other words, the component of POM or the relative organic carbon content in SPM is a more effective factor in controlling denitrification in coastal environments.
In addition to DOM and POM, the denitrification rate correlated to single cell activity and bacterial abundance. For example, the denitrifier amount per gram of particles in the turbid river water positively correlated with the organic carbon content in suspended particles.64 Obviously, particulate matter with high organic carbon should facilitate the attachment of denitrifying bacteria and subsequent denitrification.
If multiple linear regressions were applied using both bPOC (in μmol C per L) and SPM (in mg L−1) as independent variables, the NDR can be well predicted in Daya Bay with a better predictive power (r2 = 80%; p = 0.02):
| NDR = 45.1 + 2.77 × bPOC − 10.6 × SPM | (12) |
It can be seen from the regression that the NDR exhibits a positive correlation with bPOC and a negative correlation with SPM with both of their coefficients being significant (p ≤ 0.05). Therefore, high SPM but low bPOC will not enhance denitrifying activity. In other words, it is the quality of SPM that is essential for promoting denitrification. A recent study has found that the bottom nepheloid layer in coastal marine environments may be a potential hotspot for N removal, and sediment resuspension is likely the key mechanism promoting denitrification.13 In Daya Bay, the SPM contents in the bottom layers were higher than those in the surface layers, indicating the prevalence of sediment resuspension. Resuspension of sediments provides large amounts of particles and likely denitrifying bacteria into the overlying water column, which may increase the abundance of particle-associated denitrifiers and denitrifying activity.13,64 However, denitrification was observed to be inhibited by the abundant suspended particles derived from sediment resuspension (eqn (12)). Our hypothesis is that lithogenic materials introduced by sediment resuspension could dilute the organic matter content in the SPM, and reduce the energy source for the denitrification reaction. Therefore, both POC composition (especially the autochthonous component) and SPM are important factors in regulating coastal denitrifying activity, although in different directions, in highly turbid and productive ecosystems. These two parameters should be incorporated into future modeling and taken into consideration in future studies.
The relative role of DOM vs. POM in regulating denitrification
As discussed above, POM plays a more dominant role than DOM in promoting denitrifying activity in coastal environments. However, DOM has been shown to be the dominant organic matter pool and outweighs POM in seawater (e.g., Guo et al., 1995).66 Our observations that denitrifiers preferentially utilize POM over DOM by extracellular enzymes are seemingly contradictory. One plausible reason is that POM has a larger labile carbon pool than DOM. Indeed, bPOC was the major biogenic organic pool with an average concentration (27.3 μmol L−1) three times as high as that of bFDOC (an average of 8 μmol L−1) in Daya Bay (Table 1). We also found a positive linear relationship between bFDOC and bPOC (r = 0.71, p < 0.0002, n = 22) throughout the bay, indicating that bPOC is a major source of bFDOC, consistent with the carbon flow direction from particulate to colloidal to dissolved phases observed in marine environments.67,68 Additionally, fitting eqn (12) with the concentration or proportion of DOM components did not give rise to a significant regression. Thus, both DOM and its components did not seem to be relevant parameters for predicting the spatial distribution of denitrification in coastal waters. This is consistent with previous studies documenting that in estuarine and coastal systems, DOM typically contains more aged-carbon and higher C/N ratio, while the carbon in POM is often characterized as that of modern age and with lower C/N ratio.67,69 This implied that DOM contains more reworked or refractory organic materials while POM is fresher and likely more labile, which could enhance denitrification in the water column (Fig. 9).
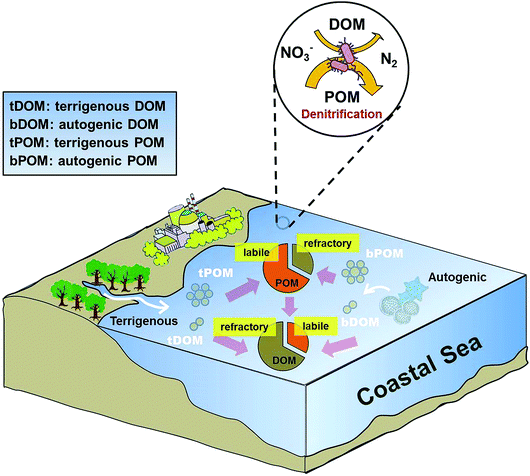 |
| Fig. 9 Conceptual diagram showing the role of organic matter in regulating denitrification in Daya Bay. | |
Conclusions
In this study, the relative importance of DOM vs. POM and the influence of different organic matter components on aquatic denitrification were investigated. Our results indicate that in coastal environments the composition of POM has a substantial impact on potential denitrification activity, while DOM and its components have little effect on it. Based on the results of multiple linear regressions, we further found that combining both SPM and autochthonous POC abundance can better predict the variability of denitrification in the study area. Therefore, both bPOC and SPM should be taken into account as variables when modelling denitrifying N removal, especially in highly turbid and productive aquatic systems. Although the water column in the study area was dominantly oxygenated and the measured denitrification rates were merely potential, our novel findings here provide new insight into the coupled effects of DOM–POM–SPM on coastal denitrification and the controlling mechanisms of marine nitrogen cycling. Further studies are needed to elucidate seasonal changes in the relationship between the denitrification rate and natural organic matter composition and to test our hypothesis. In addition, similar investigations should be conducted in typically oxygen-depleted marine waters to better understand the effects of organic matter composition on denitrification.
Author contributions
M. Chen and J. Zeng co-designed the study. J. Zeng and L. Fan were responsible for carrying out 15N-labelling incubations on board and the measurement of 15N–N2 production in laboratory. H. Lin was responsible for DOM sampling and analysis. X. Mu was responsible for POM sampling and analysis. M. Zheng and Y. Qiu contributed the experimental tools. J. Zeng, M. Chen and L. Guo co-wrote the manuscript. All authors have read and approved the final manuscript.
Conflicts of interest
The authors declare no conflicts of interest.
Acknowledgements
We wish to thank Dr Xiaoping Huang and Dr Yehui Tan for generously providing nutrient data and chlorophyll a data, respectively, of the same cruise. We also sincerely thank the whole crew for their support during the field sampling. Constructive comments from two anonymous reviewers are greatly appreciated, which improved the manuscript. This work was supported by the National Program on Key Basic Research Project (No. 2015CB452903), the Public Science and Technology Research Funds Projects of Ocean (No. 201505034), and the Chinese Natural Science Foundation (41721005).
References
- R. Knowles, Denitrification, Microbiol. Rev., 1982, 46(1), 43–70 CAS.
- L. A. Codispoti, J. A. Brandes, J. P. Christensen, A. H. Devol, S. W. A. Naqvi, H. W. Paerl and T. Yoshinari, The oceanic fixed nitrogen and nitrous oxide budgets: Moving targets as we enter the anthropocene?, Sci. Mar., 2001, 65(S2), 85–105 CrossRef CAS.
- S. P. Seitzinger, Denitrification in freshwater and coastal marine ecosystems: Ecological and geochemical significance, Limnol. Oceanogr., 1988, 33(4), 702–724 CAS.
- M. J. Kemp and W. K. Dodds, The influence of ammonium, nitrate, and dissolved oxygen concentration on uptake, nitrification, and denitrification rates associated with prairie stream substrata, Limnol. Oceanogr., 2002, 47(5), 1380–1393 CrossRef CAS.
- W. V. Sobczak, S. Findlay and S. Dye, Relationships between DOC bioavailability and nitrate removal in an upland stream: an experimental approach, Biogeochemistry, 2003, 62(3), 309–327 CrossRef CAS.
- C. P. Arango, J. L. Tank, J. L. Schaller, T. V. Royer, M. J. Bernot and M. B. David, Benthic organic carbon influences denitrification in streams with high nitrate concentration, Freshwater Biol., 2007, 52(7), 1210–1222 CrossRef CAS.
- J. P. Zarnetske, R. Haggerty, S. M. Wondzell and M. A. Baker, Labile dissolved organic carbon supply limits hyporheic denitrification, J. Geophys. Res., 2011, 116(G4), G04036 CrossRef.
- J. N. Galloway, A. R. Townsend, J. W. Erisman, M. Bekunda, Z. Cai, J. R. Freney, L. A. Martinelli, S. P. Seitzinger and M. A. Sutton, Transformation of the nitrogen cycle: Recent trends, Questions, and Potential Solutions, Science, 2008, 320(5878), 889–892 CrossRef CAS PubMed.
- P. G. Taylor and A. R. Townsend, Stoichiometric control of organic carbon–nitrate relationships from soils to the sea, Nature, 2010, 464, 1178–1181 CrossRef CAS PubMed.
- W. G. Zumft, Cell biology and molecular basis of denitrification, Microbiol. Mol. Biol. Rev., 1997, 61(4), 533–616 CAS.
- B. X. Chang, J. R. Rich, A. Jayakumar, H. Naik, A. K. Pratihary, R. G. Keil, B. B. Ward and A. H. Devol, The effect of organic carbon on fixed nitrogen loss in the eastern tropical South Pacific and Arabian Sea oxygen deficient zones, Limnol. Oceanogr., 2014, 59(4), 1267–1274 CrossRef CAS.
- S. Bonaglia, I. Klawonn, L. De Brabandere, B. Deutsch, B. Thamdrup and V. Brüchert, Denitrification and DNRA at the Baltic Sea oxic-anoxic interface: Substrate spectrum and kinetics, Limnol. Oceanogr., 2016, 61(5), 1900–1915 CrossRef CAS.
- J. Zeng, M. Chen, M. Zheng, Y. Qiu, W. He, Y. He and X. Liu, Effects of particles on potential denitrification in the coastal waters of the Beibu Gulf in China, Sci. Total Environ., 2018, 624, 1274–1286 CrossRef CAS PubMed.
- R. S. Stelzer, J. T. Scott, L. A. Bartsch and T. Parr, Particulate organic matter quality influences nitrate retention and denitrification in stream sediments: evidence from a carbon burial experiment, Biogeochemistry, 2014, 119(1–3), 387–402 CrossRef CAS.
- C. Yoshimura, M. Fujii, T. Omura and K. Tockner, Instream release of dissolved organic matter from coarse and fine particulate organic matter of different origins, Biogeochemistry, 2010, 100(1–3), 151–165 CrossRef.
- D. Bianchi, T. S. Weber, R. Kiko and C. Deutsch, Global niche of marine anaerobic metabolisms expanded by particle microenvironments, Nat. Geosci., 2018, 11, 263–268 CrossRef CAS.
- H. Ploug, Small-scale oxygen fluxes and remineralization in sinking aggregates, Limnol. Oceanogr., 2001, 46(7), 1624–1631 CrossRef CAS.
- I. Klawonn, S. Bonaglia, V. Brüchert and H. Ploug, Aerobic and anaerobic nitrogen transformation processes in N2-fixing cyanobacterial aggregates, ISME J., 2015, 9, 1456–1466 CrossRef CAS PubMed.
- P. Stief, A. Kamp, B. Thamdrup and R. N. Glud, Anaerobic nitrogen turnover by sinking diatom aggregates at varying ambient oxygen levels, Front. Microbiol., 2016, 7, 98 Search PubMed.
- C. A. Fuchsman, B. Paul, J. T. Staley, E. V. Yakushev and J. W. Murray, Detection of transient denitrification during a high organic matter event in the Black Sea, Global Biogeochem. Cycles, 2019, 33(2), 143–162 CAS.
- Y.-S. Wang, Z.-P. Lou, C.-C. Sun and S. Sun, Ecological environment changes in Daya Bay, China, from 1982 to 2004, Mar. Pollut. Bull., 2008, 56(11), 1871–1879 CrossRef CAS PubMed.
- X. Gao, S. Chen and A. Long, Composition and sources of organic matter and its solvent extractable components in surface sediments of a bay under serious anthropogenic influences: Daya Bay, China, Mar. Pollut. Bull., 2008, 56(6), 1066–1075 CrossRef CAS PubMed.
- X. Song, L. Huang, J. Zhang, X. Huang, J. Zhang, J. Yin, Y. Tan and S. Liu, Variation of phytoplankton biomass and primary production in Daya Bay during spring and summer, Mar. Pollut. Bull., 2004, 49(11–12), 1036–1044 CrossRef CAS PubMed.
- M.-L. Wu, Y.-S. Wang, Y.-T. Wang, J.-P. Yin, J.-D. Dong, Z.-Y. Jiang and F.-L. Sun, Scenarios of nutrient alterations and responses of phytoplankton in a changing Daya Bay, South China Sea, J. Mar. Syst., 2017, 165, 1–12 CrossRef.
- J. L. Zhou and K. Maskaoui, Distribution of polycyclic aromatic hydrocarbons in water and surface sediments from Daya Bay, China, Environ. Pollut., 2003, 121(2), 269–281 CrossRef CAS PubMed.
- J. Xu, Y. Wang, J. Yin, C. Sun, F. Zhang, Q. Wang, L. He and J. Dong, Nitrification and denitrification in sediment of the Daya Bay, China, Oceanol. Limnol. Sinica, 2007, 38(3), 206–211 CAS.
- C. Wang, J. Lin, P. Chen and S. Zhang, Numerical simulation on water exchange in Daya Bay, South China Fish. Sci., 2008, 4, 8–15 Search PubMed.
-
G. Z. Xu, Environments and Resources of Daya Bay, Anhui Science and Technology Publishing House, Hefei, China, 1989, pp. 10–16 Search PubMed.
- X. Mu, M. Chen, K. Zhang, J. Zeng, W. Yang, R. Zhang, M. Zheng and Y. Qiu, Stable carbon and nitrogen isotopes as tracers of sources of suspended particulate organic matter in the Daya Bay in summer, Haiyang Xuebao, 2017, 39(2), 39–52 Search PubMed.
- Z. Ke, Y. Tan, L. Huang, C. Zhao and X. Jiang, Spatial distributions of δ13C, δ15N and C/N ratios in suspended particulate organic matter of a bay under serious anthropogenic influences: Daya Bay, China, Mar. Pollut. Bull., 2017, 114(1), 183–191 CrossRef CAS PubMed.
-
K. Grasshoff, M. Ehrardt and K. Kremling, Methods of Seawater Analysis, Verlag Chemie, Weinheim, Germany, 2nd edn, 1983 Search PubMed.
-
T. R. Parsons, Y. Maita and C. M. Lalli, A manual of chemical and biological methods for seawater analyses, Pergamon Press, Oxford, 1984 Search PubMed.
- H. Lin, M. Chen, J. Zeng, Q. Li, R. Jia, X. Sun, M. Zheng and Y. Qiu, Size characteristics of chromophoric dissolved organic matter in the Chukchi Sea, J. Geophys. Res.: Oceans, 2016, 121(8), 6403–6417 CAS.
- C. A. Stedmon, S. Markager and R. Bro, Tracing dissolved organic matter in aquatic environments using a new approach to fluorescence spectroscopy, Mar. Chem., 2003, 82(3–4), 239–254 CrossRef CAS.
- Y. Yamashita, R. Jaffé, N. Maie and E. Tanoue, Assessing the dynamics of dissolved organic matter (DOM) in coastal environments by excitation emission matrix fluorescence and parallel analysis (EEM-PARAFAC), Limnol. Oceanogr., 2008, 53(5), 1900–1908 CrossRef CAS.
- C. A. Stedmon and S. Markager, Resolving the variability in dissolved organic matter fluorescence in a temperate estuary and its catchment using PARAFAC analysis, Limnol. Oceanogr., 2005, 50(2), 686–697 CrossRef CAS.
- P. Lam and M. M. M. Kuypers, Microbial nitrogen cycling processes in oxygen minimum zones, Annu. Rev. Mar. Sci., 2011, 3, 317–345 CrossRef PubMed.
- J. Zeng, M. Chen, M. Zheng, W. Hu and Y. Qiu, A potential nitrogen sink discovered in the oxygenated Chukchi Shelf waters of the Arctic, Geochem. Trans., 2017, 18, 5 CrossRef PubMed.
- T. Dalsgaard, L. De Brabandere and P. O. J. Hall, Denitrification in the water column of the central Baltic Sea, Geochim. Cosmochim. Acta, 2013, 106, 247–260 CrossRef CAS.
- M. M. Jensen, J. Petersen, T. Dalsgaard and B. Thamdrup, Pathways, rates, and regulation of N2 production in the chemocline of an anoxic basin, Mariager Fjord, Denmark, Mar. Chem., 2009, 113(1–2), 102–113 CrossRef CAS.
- A. Galán, J. Faúndez, B. Thamdrup, J. F. Santibáñez and L. Farías, Temporal dynamics of nitrogen loss in the coastal upwelling ecosystem off central Chile: Evidence of autotrophic denitrification through sulfide oxidation, Limnol. Oceanogr., 2014, 59(6), 1865–1878 CrossRef.
- R. T. Barnes, R. L. Smith and G. R. Aiken, Linkages between denitrification and dissolved organic matter quality, Boulder Creek watershed, Colorado, J. Geophys. Res., 2012, 117(G1), G01014 CrossRef.
- T. J. Battin, L. A. Kaplan, S. Findlay, C. S. Hopkinson, E. Marti, A. I. Packman, J. D. Newbold and F. Sabater, Biophysical controls on organic carbon fluxes in fluvial networks, Nat. Geosci., 2009, 1, 95–100 CrossRef.
- M. A. Baker and P. Vervier, Hydrological variability, organic matters supply and denitrification in the Garonne River ecosystem, Freshwater Biol., 2004, 49(2), 181–190 CrossRef CAS.
- K. S. Herrman, V. Bouchard and R. H. Moore, Factors affecting denitrification in agricultural headwater streams in northeast Ohio, USA, Hydrobiologia, 2008, 598(1), 305–314 CrossRef CAS.
- K. S. Pfenning and P. B. McMahon, Effect of nitrate, organic carbon, and temperature on potential denitrification rates in nitrate-rich riverbed sediments, J. Hydrol., 1996, 187(3–4), 283–295 Search PubMed.
- K. Sanderson, Lignocellulose: a chewy problem, Nature, 2011, 474, S12–S14 CrossRef CAS PubMed.
- T. Nozawa and Y. Maruyama, Denitrification by a soil bacterium with phthalate and other aromatic compounds as substrate, J. Bacteriol., 1988, 170(6), 2501–2505 CrossRef CAS PubMed.
- F. Guillemette, S. L. McCallister and P. A. del Giorgio, Differentiating the degradation dynamics of algal and terrestrial carbon with within complex natural dissolved organic carbon in temperate lakes, J. Geophys. Res.: Biogeosci., 2013, 118(3), 963–973 CrossRef CAS.
- H. F. Wilson and M. A. Xenopoulos, Ecosystem and seasonal control of stream dissolved organic carbon along a gradient of land use, Ecosystems, 2008, 11(4), 555–568 CrossRef CAS.
- E. Asmala, R. Autio, H. Kaartokallio, L. Pitkänen, C. A. Stedmon and D. Thomas, Bioavailability of riverine dissolved organic matter in three Baltic Sea estuaries and the effect of catchment land use, Biogeosciences, 2013, 10(11), 6969–6986 CrossRef CAS.
- M. Berggren and P. A. del Giorgio, Distinct patterns of microbial metabolism associated to riverine dissolved organic carbon of different source and quality, J. Geophys. Res.: Biogeosci., 2015, 120(6), 989–999 CrossRef CAS.
- H. Xie, O. C. Zafiriou, W.-J. Cai, R. G. Zepp and Y. Wang, Photooxidation and its effects on carboxyl content of dissolved organic matter in two coastal rivers in the southeastern United State, Environ. Sci. Technol., 2004, 38(15), 4113–4119 CrossRef CAS PubMed.
- T. Dalsgaard, F. J. Stewart, B. Thamdrup, L. De Brabanere, N. P. Revsbech, O. Ulloa, D. E. Canfield and E. F. DeLong, Oxygen at nanomolar levels reversibly suppresses process rates and gene expression in anammox and denitrification in the Oxygen Minimum Zone off northern Chile, mBio, 2014, 5(6), e01966–14 CrossRef CAS PubMed.
-
E. D. Goldberg, Black Carbon in the Environment: Properties and Distribution, Wiley, New York, 1985 Search PubMed.
- M. I. Bird, C. Moyo, E. M. Veenendaal, J. Lloyd and P. Frost, Stability of elemental carbon in a savanna soil, Global Biogeochem. Cycles, 1999, 13(4), 923–932 CrossRef CAS.
- A. I. Coppola, D. B. Wiedemeier and V. Galy,
et al., Global-scale evidence for the refractory nature of riverine black carbon, Nat. Geosci., 2018, 11, 584–588 CrossRef CAS.
- R. Jaffé, Y. Ding, J. Niggemann, A. V. Vähätalo, A. Stubbins, R. G. M. Spencer, J. Campbell and T. Dittmar, Global charcoal mobilization from soils via dissolution and riverine transport to the oceans, Science, 2013, 340(6130), 345–347 CrossRef PubMed.
- C. P. Ward, R. L. Sleighter, P. G. Hatcher and R. M. Cory, Insights into the complete and partial photooxidation of black carbon in surface waters, Environ. Sci.: Processes Impacts, 2014, 16(4), 721–731 RSC.
- T. Dittmar, The molecular level determination of black carbon in marine dissolved organic matter, Org. Geochem., 2008, 39(4), 396–407 CrossRef CAS.
- X. Wang, C. Xu, E. M. Druffel, Y. Xue and Y. Qi, Two black carbon pools transported by the Changjiang and Huanghe Rivers in China, Global Biogeochem. Cycles, 2016, 30(12), 1778–1790 CrossRef.
- S. K. Dodla, J. J. Wang, R. D. DeLaune and R. L. Cook, Denitrification potential and its relation to organic carbon quality in three coastal wetland soil, Sci. Total Environ., 2008, 407(1), 471–480 CrossRef CAS PubMed.
- S. K. Bastviken, P. G. Eriksson, A. Premrov and K. Tonderski, Potential denitrification in wetland sediments with different plant species detritus, Ecol. Eng., 2005, 25(2), 183–190 CrossRef.
- T. Liu, X. Xia, S. Liu, X. Mou and Y. Qiu, Acceleration of denitrification in turbid rivers due to denitrification occurring on suspended sediment in oxic waters, Environ. Sci. Technol., 2013, 47(9), 4053–4061 CrossRef CAS PubMed.
- C. T. E. Kellogg and J. W. Deming, Particle-associated extracellular enzyme activity and bacterial community composition across the Canadian Arctic Ocean, FEMS Microbiol. Ecol., 2014, 89(2), 360–375 CrossRef CAS PubMed.
- L. Guo, P. H. Santschi and K. W. Warnken, Dynamics of dissolved organic carbon (DOC) in oceanic environments, Limnol. Oceanogr., 1995, 40(8), 1392–1403 CrossRef CAS.
- L. Guo, P. H. Santschi, L. A. Cifuentes, S. E. Trumbore and J. Southon, Cycling of high-molecular-weight dissolved organic matter in the Middle Atlantic Bight as revealed by carbon isotopic (13C and 14C) signatures, Limnol. Oceanogr., 1996, 41(6), 1242–1252 CrossRef CAS.
- L. Guo and P. H. Santschi, Isotopic and elemental characterization of colloidal organic matter from the Chesapeake Bay and Galveston Bay, Mar. Chem., 1997, 59(1–2), 1–15 CrossRef CAS.
- P. H. Santschi, L. Guo, M. Baskaran, S. Trumbore, J. Southon, T. S. Bianchi, B. Honeyman and L. Cifuentes, Isotopic evidence for the contemporary origin of high-molecular weight organic matter in oceanic environments, Geochim. Cosmochim. Ac., 1995, 59(3), 625–631 CrossRef CAS.
|
This journal is © The Royal Society of Chemistry 2019 |