Combined effects of dissolved organic matter, pH, ionic strength and halides on photodegradation of oxytetracycline in simulated estuarine waters†
Received
15th October 2018
, Accepted 15th December 2018
First published on 17th December 2018
Abstract
Estuarine waters of variable compositions are sinks for many micropollutants. The varying water properties can impact the photodegradation of organic pollutants. In this study, the combined effects of dissolved organic matter (DOM), pH, ionic strength, and halides on the photodegradation of the model organic pollutant oxytetracycline (OTC) were investigated. Suwannee River natural organic matter (SRNOM) was used as a representative DOM. The results showed that the observed photolysis rate constant (kobs) of OTC increased rapidly upon increase of pH. SRNOM induced a 11.0–17.9% decrease of the kobs for OTC. In the presence of SRNOM, the ionic strength and specific halide effects promote OTC photodegradation with a 39.2–84.2% and 7.1–28.8% increase of the kobs, respectively. The effects of SRNOM, ionic strength and halides on OTC photodegradation are pH-dependent. Direct photolysis half-lives (t1/2) of OTC were estimated in view of the more important role of direct photolysis compared to indirect photolysis. The estimated t1/2 values decreased from 187.4–206.6 d to 34.4–36.6 d as the pH increases in the Yellow River estuarine region. The results of this research demonstrate that the photodegradation rate of OTC increases rapidly in the gradient from river water to marine water in estuarine regions.
Environmental significance
Photodegradation behaviors of organic pollutants would be complex and variable in estuarine waters due to the rapid change of physical and chemical properties and constitution, such as pH, ionic strength, dissolved organic matter (DOM), and halides. The combined effects of these variable factors on the photodegradation of frequently detected antibiotics in estuarine waters are still unclear. Here, we prove that the inhibitory effect of DOM and promotional effects of ionic strength and specific halides on oxytetracycline (OTC) photodegradation are all pH dependent. The combined effects of these factors lead to the decrease of photochemical half-lives for OTC in estuarine regions from river water to marine water.
|
Introduction
Estuaries are dynamic systems due to oceanic and terrestrial influences.1 They play important roles in the accumulation and degradation of micropollutants2,3 which enter estuarine waters via riverine transport, discharge from land-based sources, atmospheric deposition, and mariculture activities.4,5 Among various micropollutants, antibiotics have received increasing attention in response to their widespread occurrence6–8 and relatively higher concentrations were detected in estuarine and coastal areas compared to surrounding river and marine areas.4,9 Alarmingly, antibiotics are able to induce bacterial resistance that is severely harmful to human health.10,11 It is thus of significant importance to investigate the degradation behavior of antibiotics in estuarine waters.
Photochemical transformation was proven to be the predominant elimination pathway of antibiotics in sunlit surface waters.12–15 Antibiotics, such as tetracyclines (TCs), can undergo direct photolysis as well as indirect photolysis mediated by singlet oxygen (1O2) and hydroxyl radicals (·OH).16–18 Meanwhile, dissolved organic matter (DOM), which is ubiquitous in natural waters, plays an important role in the photodegradation of organic pollutants.19–21 Previous studies found that humic acid (HA) inhibits phototransformation of TCs through competitive light absorption or photochemically produced reactive intermediate (PPRI) quenching, but on the other hand the excited triplet HA, and the generated 1O2 and ·OH accelerated the photodegradation of TCs.16,18
The photochemical transformation of ionizable antibiotics was found to be pH dependent.22,23 It may also be affected by ionic strength and halides in surface waters.24 Notably, pH, ionic strength, and halide concentrations can in turn influence the photochemical properties of DOM. Firstly, the pH of the surrounding environment affects the absorbance of DOM.25 Secondly, both pH and ionic strength heavily influence the formation yields of PPRIs by DOM. Dalrymple et al. found that the quantum yields of 1O2 and hydrogen peroxide (H2O2) from DOM exhibited a linear dependence on E2/E3 (A254 nm divided by A365 nm) which decreases as pH increases.26 The quantum yields of excited triplet DOM (3DOM*) also changed significantly with pH and ionic strength.27,28 Thirdly, halides can impact photoexcitation and photobleaching of DOM, which has been described in recent reviews.29,30
In estuarine regions, pH always increases from freshwater to seawater during mixing.31 Accompanied by the pH increase, ionic strength and the concentrations of halogen ions (Cl− and Br−) within estuary systems also increase from river water to seawater.32 Parker et al. investigated the effect of ionic strength and halides with DOM on the photodegradation of microcystins in estuarine systems and emphasized the important role of halides.33 The combined effect of these important factors with DOM on the photodegradation of ionizable antibiotics in estuarine waters seems more complex and unpredictable because of the rapid change of the physical and chemical properties during the mixing of river water with seawater. Estuaries are considered to be the most important path for organic pollutant delivery to oceans.9 It is hence of great environmental significance to investigate the combined effects of dissolved compounds on the phototransformation of antibiotics, which determines their persistence in estuarine waters.
In this study, oxytetracycline (OTC) was chosen as the target antibiotic due to its frequency of detection and elevated concentration in estuarine waters.3 The structure and pKa (3.22, 7.46, and 8.94)34 of OTC are shown in Fig. S1 in the ESI.† The effects of DOM on OTC photodegradation were investigated in solutions of varying acidity. The underlying mechanisms by which DOM impacted the photodegradation of four ionic species of OTC [H3L+, H2L, HL−, and L2− (L represents OTC)] were also unveiled. Furthermore, the combined effects of ionic strength and halides with DOM on OTC photodegradation under different pH conditions were also explored. Solutions with different ionic strength, halide concentrations, and acidity were used to mimic the complex and dynamic estuarine environments. To the best of our knowledge, there are to date no other studies investigating the combined effects of DOM, pH, ionic strength, and halides on the photodegradation of OTC.
Materials and methods
Materials
Oxytetracycline dihydrate (OTC, 98%), sodium azide (NaN3, 98%), 2,4,6-trimethylphenol (TMP, 98%), and sorbic acid (SA, 99%) were purchased from J&K Scientific Ltd. (Beijing, China). All organic solvents with chromatographic purity were purchased from TEDIA (USA). Suwannee River natural organic matter (SRNOM) was purchased from the International Humic Substances Society. Other chemicals, such as sodium chloride (NaCl), sodium bromide (NaBr), and sodium sulphate (NaSO4), were all analytically pure and purchased from Tianjin Damao Chemical (Tianjin, China). Ultrapure water (18.2 MΩ) was obtained from a purification system produced by Chengdu Ultrapure Technology Co., Ltd. (China).
Irradiation experiments
OTC was dissolved at an initial concentration of 5.0 mg L−1. H2SO4 and NaOH were applied to adjust the pH to 2.0, 3.2, 5.0, 7.0, 8.0, 9.0, and 12.0. The fractions of H3L+, H2L, HL−, and L2− in these solutions of different pH are listed in Table S1.† The irradiation experiments were performed in an XPA-7 merry-go-round photochemical reactor (Xujiang Electromechanical Plant, Nanjing, China) with quartz tubes containing the reaction solutions. A water-refrigerated 1000 W xenon lamp equipped with 290 nm filters was used to mimic the UV-A and UV-B portions of sunlight. The light intensity at the surface of quartz tubes and the sunlight radiation spectrum were detected with a TriOS-RAMSES spectroradiometer (TriOS GmbH, Germany), and the results are shown in Fig. S2.† The total radiation intensity from 290 to 450 nm of the light source in the position of the quartz cuvettes was measured to be 11.4 mW cm−2, which is slightly lower than that of sunlight (12.8 mW cm−2) measured at noon in mid-summer in Dalian, China. A water bath unit was employed to maintain the temperature at 25 °C.
Quenching experiments were performed with SA (2.0 mM) and TMP (2.0 mM) as excited triplet quenchers, NaN3 (2.0 mM) as the 1O2 quencher, and isopropyl alcohol (IPA, 20.0 mM) as the ·OH quencher, which were also selected in previous studies.35–39 SA and TMP quench the excited triplet state through energy transfer and electron transfer, respectively.36,39 The observed photolysis rate constants (kobs) of OTC in the presence of SA and TMP were corrected by calculating their screening factors. For some experiments, SRNOM was added in OTC solutions with concentrations of 5.0 mg L−1 and 10.0 mg L−1. Na2SO4 (0.020, 0.050, 0.100, and 0.250 mol L−1), NaCl (0.030, 0.075, 0.150, and 0.375 mol L−1) or NaBr (0.075 mol L−1 and 0.80 mmol L−1) was added at different concentrations to investigate the effects of ionic strength and specific halides.
The light screening factor of SRNOM (Sλ) was calculated with the method described by Zhang et al.40Sλ and the integrated screening factor for the wavelength range 290–455 nm (S290–455) were calculated using the following equations:
|  | (1) |
|  | (2) |
where
αλ is the light attenuation coefficient of SRNOM (cm
−1),
ελ is the molar absorption coefficient of OTC (cm
−1 L mol
−1), and
l is the light path length (cm) which was calculated with the method described in previous studies.
20,41 The
αλ and
ελ values in different pH solutions were determined to calculate the
S290–455 of SRNOM. The indirect photolysis rate constant (
kind) as caused by DOM was calculated using the following equation:
| kind = kobs(DOM) − kobs(non-DOM)S290–455 | (3) |
where
kobs(DOM) and
kobs(non-DOM) are the observed photolysis rate constants of the OTC in the presence and absence of SRNOM, respectively.
The kobs values of the four species of OTC (H3L+, H2L, HL−, and L2−) were calculated with the determined kobs values of OTC in solutions with a pH of 2.0, 3.2, 5.0, 7.0, 9.0, and 12.0 based on the method described by Jin et al.22 (details are shown in Text S1†). The molar absorptivity from 220 nm to 500 nm for different species of OTC was also calculated based on the same method (details in the ESI†). Eqn (1)–(3) were also used in the case of the different OTC species. In eqn (1), the αλ values of SRNOM at a pH of 2.0, 5.0, 8.0, and 12.0 were used to calculate the Sλ of H3L+, H2L, HL−, and L2−, respectively, as these four species are the main existing forms of OTC in these four pH solutions, respectively (as shown in Table S1†). The ελ of H3L+, H2L, HL−, and L2− was obtained with the method described in Text S1.† The concentrations of the four species were calculated with eqn (4):
where
ci is the concentration of different species and
αi is the fraction of H
3L
+, H
2L, HL
−, and L
2− at pH 2.0, 5.0, 8.0, and 12.0, respectively.
Analytical methods
A Shimadzu LC-20A HPLC system (Shimadzu, Kyoto, Japan) with a UV-Vis detector and an Ultimate™ AQ-C18 column (250 mm × 4.6 mm, 5 μm, Welch Materials, Maryland, USA) was employed for the quantification of OTC. The detection wavelength for OTC was 355 nm. The mobile phase consisted of an acetonitrile and phosphate aqueous solution (0.1%, pH = 2.3) with a ratio of 30
:
70.
Results and discussion
Effects of DOM on OTC photodegradation under different pH conditions
Photodegradation of OTC followed pseudo first-order kinetics (Fig. S3†). No significant loss of OTC was observed in the dark controls, indicating that light irradiation is the driving force for the degradation of OTC in the experiments. The observed photolysis rate constants (kobs) of OTC exhibited a positive dependence on the pH values of the aqueous solutions (Fig. 1, Table S2†). This is in accordance with previous findings stating that the direct photolysis rate constant (kd) of OTC increased with increasing pH, which was attributed to the increased light absorbance at increasing pH.22 A 4.6-fold increase of the kobs for OTC was observed as the pH increased from 6.0 to 9.0, which represents the pH range of surface waters.42
 |
| Fig. 1 Experimental and predicted photolysis rate constants (kobs) and SRNOM-induced indirect photolysis rate constants (kind) of OTC under different pH conditions (The inset figure shows the details of kobs and kind for OTC at pH from 2.0 to 7.0; the error bars represent the 95% confidence interval, n = 3). | |
Quenching and nitrogen saturation experiments were performed to investigate the involvement of self-sensitized photolysis in OTC photodegradation. Addition of NaN3 and IPA significantly reduced the kobs at pH 9.0 and showed no significant effects on the kobs in pH 5.0 and 7.0 solutions (Fig. S4†). Thus, 1O2 and ·OH-initiated self-sensitized oxidation reactions were only observed at pH 9.0 and not at pH 5.0 and 7.0 for the studied photolysis of OTC. Upon nitrogen saturation, the kobs increased significantly in the solutions at pH 5.0 and 7.0 (Fig. S4†), implying that OTC can undergo direct photodegradation through its excited triplet state as dissolved oxygen (O2) is a triplet quencher. However, in solutions at pH 9.0, the kobs decreased with nitrogen saturation. This further confirmed the involvement of self-sensitized oxidation reactions as the quenching of the excited triplet state by O2 leads to the generation of 1O2 that was proven to be involved in the phototransformation of OTC in pH 9.0 solutions.
In the presence of SRNOM (5.0 mg L−1 and 10.0 mg L−1), the kobs of OTC was significantly decreased (p < 0.05) in different pH solutions (Fig. 1). The S290–455 of SRNOM in different pH solutions was calculated (Table S2†). With the S290–455 and kobs values at different pH, the indirect photolysis rate constants (kind) induced by SRNOM were calculated using eqn (3) and the values are listed in Table S2.† As can be seen in Fig. 1, the kind values also increased with increasing pH (Table S2†). Overall, SRNOM induced an 11.0–17.9% decrease of the kobs for OTC in solutions with pH from 6.0 to 9.0.
The kobs values for the four species of OTC were calculated with the method as shown in Text S1.† The calculated kobs for H3L+, H2L, HL−, and L2− was 0.20 ± 0.02, 0.62 ± 0.03, 1.32 ± 0.07, and 5.52 ± 0.15 min−1, respectively, which also decreased in the presence of SRNOM (Table 1). With the calculated kobs and molar absorptivity (Fig. S5†), the SRNOM-induced kind of the four species was calculated, and the results are shown in Table 1. The kind (10.0 mg L−1 SRNOM) increased from about zero for H3L+ to 1.28 ± 0.15 min−1 for L2−. The kobs and kind (10.0 mg L−1 SRNOM) values for OTC at different pH were calculated based on the kobs and kind for the four species and their percentages in different pH solutions. As can be seen in Fig. 1, the calculated kobs and kind of OTC increased with increasing pH and showed good agreement with the determined values at pH 2.0, 3.2, 5.0, 7.0, 8.0, 9.0, and 12.0. Thus, it can be concluded from the results that kobs in the absence and presence of DOM and the DOM-induced kind for ionizable pollutants can be reliably predicted.
Table 1 Calculated kobs and kind for different species of OTC and S290–455 of SRNOM towards different species of OTC (The unit for kobs and kind is ×10−2 min−1)a
Condition |
k
|
H3L+ |
H2L |
HL− |
L2− |
The errors of kobs and kind represent 95% confidence levels, n = 3.
|
Without SRNOM |
k
obs
|
0.20 ± 0.02 |
0.62 ± 0.03 |
1.32 ± 0.07 |
5.52 ± 0.15 |
With 5.0 mg L−1 SRNOM |
k
obs
|
0.19 ± 0.01 |
0.52 ± 0.01 |
1.31 ± 0.05 |
4.99 ± 0.19 |
S
290–455
|
0.736 |
0.708 |
0.728 |
0.702 |
k
ind
|
0.04 ± 0.01 |
0.08 ± 0.03 |
0.35 ± 0.06 |
1.11 ± 0.20 |
With 10.0 mg L−1 SRNOM |
k
obs
|
0.12 ± 0.01 |
0.49 ± 0.01 |
1.14 ± 0.07 |
4.97 ± 0.07 |
S
290–455
|
0.702 |
0.671 |
0.703 |
0.667 |
k
ind
|
−0.02 ± 0.02 |
0.08 ± 0.02 |
0.21 ± 0.08 |
1.28 ± 0.15 |
To better understand the effects of SRNOM on the photolysis of OTC, quenching experiments were performed in pH 5.0, 7.0, and 9.0 solutions, and the results are shown in Fig. 2. The results showed that in the presence of SRNOM, removal of O2 (N2 saturation) increased the kobs of OTC, implying that O2 (a triplet quencher) has a negative effect on the photolysis rate. Meanwhile, addition of SA and TMP significantly decreased the kobs of OTC in solutions at pH 5.0, 7.0, and 9.0 (Fig. 2), implying that excited triplet states of SRNOM (3SRNOM*) were involved in sensitizing the indirect photolysis of OTC. The 3DOM*-sensitized photodegradation was also proven to be involved in the photolysis of other antibiotics.43 Addition of NaN3 and IPA significantly reduced the kobs in pH 7.0 and 9.0 solutions (Fig. 2), implying that 1O2 and ·OH-initiated indirect photolysis by SRNOM was involved in the OTC photolysis in solutions of high pH.
 |
| Fig. 2 Observed first-order photolysis rate constants (kobs) of OTC in the presence of 10.0 mg L−1 SRNOM under different conditions (* represents significant difference, p < 0.05, n = 3; the error bars represent the 95% confidence interval, n = 3). | |
Ionic strength and specific halide effects at different pH
According to previous studies, ionic strength can accelerate the photodegradation of antibiotics by increasing the steady state concentrations of their excited triplet states.24,44 Meanwhile, halides in coastal and estuarine waters can promote the photodegradation of organic micropollutants by generating halogen radicals.45,46 Thus, ionic strength and specific halide effects were investigated using Na2SO4, NaCl, and NaBr. The concentrations of Na2SO4 ranged from 0.020 to 0.250 mol L−1 with the corresponding salinity from 2.8‰ to 35.5‰, which can be applied to mimic the salinity change in estuarine regions. As can be seen in Fig. 3a and b, the kobs of OTC increased with the increasing Na2SO4 concentration at pH 7.0 and 9.0. This indicates that ionic strength has a positive effect on OTC photodegradation.
 |
| Fig. 3 Observed first-order photolysis rate constants (kobs) of OTC in Na2SO4 (a and b) and NaCl (c and d) solutions at pH 7.0 and 9.0 (* represents significant difference, p < 0.05, n = 3; the error bars represent the 95% confidence interval, n = 3). | |
In the presence of SRNOM (10.0 mg L−1), the promotional effect of ionic strength is much higher in pH 9.0 solutions (84.2% increase as the concentration of Na2SO4 increases from 0.020 mol L−1 to 0.250 mol L−1) than in solutions at pH 7.0 (39.2% increase) and also higher than that in the absence of SRNOM at pH 9.0 (54.5%). As shown in Fig. S6,† SRNOM-induced kind values also significantly increased with increasing ionic strength, especially in pH 9.0 solutions. The solutions with high ionic strength can enhance the steady state concentration of 3SRNOM*, leading to the promotion of indirect photolysis initiated by 3SRNOM*, 1O2, and ·OH. As discussed above, SRNOM-induced kind increased with increasing pH. Thus, the promotional effects of ionic strength on indirect photolysis induced by SRNOM are higher in pH 9.0 solutions than in solutions at pH 7.0. In estuarine environments, the ionic strength increases rapidly from river water to seawater due to seawater encroachment.32 The increase of ionic strength led to a 30.6–54.5% and 39.2–84.2% increase of the kobs for OTC in the absence and presence of SRNOM, respectively.
Specific Cl− effects were studied using NaCl with concentrations from 0.030 to 0.375 mol L−1. In the absence of SRNOM, the kobs of OTC was seen to increase with the increasing NaCl concentration (Fig. 3c and d) due to the ionic strength effect as shown above. However, in the presence of SRNOM (10.0 mg L−1), the kobs of OTC in NaCl solutions with a concentration of 0.375 mol L−1 showed no obvious increase compared to that in NaCl solutions with a concentration of 0.150 mol L−1 at pH 7.0, whereas kobs decreased significantly at pH 9.0. It can be concluded that aside from the ionic strength effects, specific Cl− effects can also influence OTC photolysis. As shown in Fig. S6,† the SRNOM-induced kind of OTC exhibited an increase-decrease trend in NaCl solutions. This was considered to be attributable to the quenching of 3SRNOM* and ·OH by a high concentration of Cl− (0.375 mol L−1).32
The specific effects of Br− and mixtures of Cl− + Br− were also investigated in solutions in the presence of 10.0 mg L−1 SRNOM and with an ionic strength of 0.075 mol L−1. The values of the kobs of OTC under different experimental conditions are listed in Table S3.† The value of the SRNOM-induced kind of OTC was calculated by means of eqn (3), and the results are shown in Fig. 4. As can be seen in Fig. 4, the presence of 0.075 mol L−1 Br− significantly increased the kind at pH 6.0, 7.0, 8.0, and 9.0, and the increase of kind is obviously higher than that in the presence of Cl− at the same concentration. This can be interpreted by the lower oxidation potential of Br− (1.6 eV) as compared to Cl− (2.0 eV), which leads to easier generation of bromine radicals.47 The kind value obviously decreased as the Br− concentration decreased to 0.80 mmol L−1 (the Br− concentration in seawater). However, coexistence of Cl− + Br− with SRNOM further increased the kind as compared to the value of kind in the presence of 0.075 mol L−1 Cl− or 0.075 mol L−1 Br− with SRNOM. The specific halide effects can lead to a 7.1–28.8% increase of the kobs for OTC.
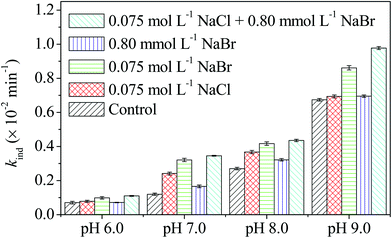 |
| Fig. 4 SRNOM-induced indirect photolysis rate constants (kind) of OTC in Na2SO4, NaCl, and NaBr solutions (* represents significant difference, p < 0.05, n = 3; the error bars represent the 95% confidence interval, n = 3). | |
Estimation of environmental photodegradation half-lives for OTC
As shown above, SRNOM can influence the photodegradation of OTC through light screening and sensitizing effects. Both ionic strength and specific halides can enhance SRNOM-induced indirect photolysis. Nevertheless, direct photolysis is the most important degradation pathway of OTC in solutions of different pH, ionic strength and halide concentrations. Therefore, quantum yields (Φ) for the direct photolysis of the four species of OTC were calculated with the method described in Text S1.† The Φ values for H3L+, H2L, HL−, and L2− are (1.22 ± 0.12) × 10−4, (3.39 ± 0.16) × 10−4, (5.32 ± 0.28) × 10−4, and (17.5 ± 0.48) × 10−4, respectively. Among the four factors, pH plays the most important role in increasing the kobs of OTC. Thus, the Φ values of OTC at different pH were calculated, and the direct photolysis half-lives (t1/2) of OTC in natural waters were predicted with the models established by Zhou et al.48 The t1/2 values of OTC in river, estuarine, and marine waters with a depth of 8 m (average mixed layer depth of the Bohai Sea and the Yellow Sea in summer49) in the Yellow River estuarine region were predicted, and the results are shown in Fig. 5.
 |
| Fig. 5 Estimated direct photolysis half-lives (t1/2) of OTC in river, estuarine, and marine waters in the Yellow River estuarine region. | |
As can be seen in Fig. 5, the t1/2 values of OTC decreased rapidly with the increase of pH and are lowest in marine water as compared to estuarine and river water. Only taking the estuarine water into account, the t1/2 values of OTC decreased from 187.4–206.6 d at pH 6.0 to 34.4–36.6 d at pH 9.0. Besides pH, ionic strength and specific halides can promote the photodegradation of OTC, whereas DOM inhibits the photodegradation. The pH, ionic strength and halide concentrations increased in the estuarine regions from river water to marine water. Meanwhile, the reported concentration of DOM in seawater was lower than that in freshwater.41 Hence, the sunlight relevant t1/2 values of OTC must decrease rapidly from river water to seawater in the estuarine regions.
Conclusion
In this study, the combined effects of SRNOM, pH, ionic strength, and halides on the photodegradation of OTC were investigated. The results showed that SRNOM can inhibit the photodegradation of OTC, and pH, ionic strength, and halides promote the photodegradation of OTC. The effects of SRNOM, ionic strength, and halides are all pH-dependent. Meanwhile, ionic strength and specific halides can enhance the indirect photolysis induced by SRNOM, and the enhancement effect is also pH-dependent. Thus, among the four factors investigated, pH plays the most important role in promoting the photodegradation of OTC. In waters of different compositions (different pH, ionic strength, and halide or DOM concentrations), direct photolysis plays a dominant role in OTC photodegradation as compared to indirect photolysis. The direct photolysis half-lives were estimated to be rapidly decreasing in the estuarine regions along the gradient from river water to marine water. This study provided profound insights into the combined effects of SRNOM, pH, ionic strength, and halides on the photodegradation of OTC. It is of great significance to elucidate the photochemical transformation behaviors of ionizable micropollutants in estuarine regions where the environmental conditions (e.g. pH, ionic strength, and halide concentrations) are dynamic.
Conflicts of interest
There are no conflicts of interest to declare.
Acknowledgements
This study was supported by the National Natural Science Foundation of China (21707017, 41877364 and 51479005), the China Postdoctoral Science Foundation (2017M621194), the Fundamental Research Funds for the Central Universities (2412017QD025) and the Jilin Province Science and Technology Development Projects (20180520079JH).
References
-
K. R. Dyer, Estuaries: a Physical Introduction, John Wiley and Sons, West Sussex, 1997, p. 195 Search PubMed.
- Y. Aminot, K. Le Menach, P. Pardon, H. Etcheber and H. Budzinski, Inputs and seasonal removal of pharmaceuticals in the estuarine Garonne River, Mar. Chem., 2016, 185, 3–11 CrossRef CAS.
- C. Yan, Y. Yang, J. Zhou, M. Liu, M. Nie, H. Shi and L. Gu, Antibiotics in the surface water of the Yangtze Estuary: Occurrence, distribution and risk assessment, Environ. Pollut., 2013, 175, 22–29 CrossRef CAS PubMed.
- K. V. Thomas and M. J. Hilton, The occurrence of selected human pharmaceutical compounds in UK estuaries, Mar. Pollut. Bull., 2004, 49, 436–444 CrossRef CAS PubMed.
- W. H. Xu, W. Yan, X. D. Li, Y. D. Zou, X. X. Chen, W. X. Huang, L. Miao, R. J. Zhang, G. Zhang and S. C. Zou, Antibiotics in riverine runoff of the pearl river delta and pearl river estuary, China: Concentrations, mass loading and ecological risks, Environ. Pollut., 2013, 182, 402–407 CrossRef CAS PubMed.
- J. Du, H. Zhao, S. Liu, H. Xie, Y. Wang and J. Chen, Antibiotics in the coastal water of the south Yellow Sea in China: occurrence, distribution and ecological risks, Sci. Total Environ., 2017, 595, 521–527 CrossRef CAS PubMed.
- Y. Luo, L. Xu, M. Rysz, Y. Wang, H. Zhang and P. J. J. Alvarez, Occurrence and transport of tetracycline, sulfonamide, quinolone, and macrolide antibiotics in the Haihe River basin, China, Water Res., 2011, 45, 1827–1833 CAS.
- S. Rodriguez-Mozaz, S. Chamorro, E. Marti, B. Huerta, M. Gros, A. Sànchez-Melsió, C. M. Borrego, D. Barceló and J. L. Balcázar, Occurrence of antibiotics and antibiotic resistance genes in hospital and urban wastewaters and their impact on the receiving river, Water Res., 2015, 69, 234–242 CrossRef CAS PubMed.
- S. Li, W. Shi, M. Li, N. Xu, R. Zhang, X. Chen, W. Sun, D. Wen, S. He, J. Pan, Z. He and Y. Fan, Antibiotics in water and sediments of rivers and coastal area of Zhuhai city, Pearl River estuary, south China, Sci. Total Environ., 2018, 636, 1009–1019 CrossRef CAS PubMed.
- M. Baym, L. K. Stone and R. Kishony, Multidrug evolutionary strategies to reverse antibiotic resistance, Science, 2016, 351, aad3292 CrossRef PubMed.
- P. M. C. Huijbers, H. Blaak, M. C. M. de Jong, E. A. M. Graat, C. M. J. E. Vandenbroucke-Grauls and A. M. de Roda Husman, Role of the environment in the transmission of antimicrobial resistance to humans: A review, Environ. Sci. Technol., 2015, 49, 11993–12004 CrossRef CAS PubMed.
- R. M. Baena-Nogueras, E. González-Mazo and P. A. Lara-Martín, Photolysis of antibiotics under simulated sunlight irradiation: Identification of photoproducts by high-resolution mass spectrometry, Environ. Sci. Technol., 2018, 51, 3148–3156 CrossRef PubMed.
- S. Bahnmüller, U. von Gunten and S. Canonica, Sunlight-induced transformation of sulfadiazine and sulfamethoxazole in surface waters and wastewater effluents, Water Res., 2014, 57, 183–192 CrossRef PubMed.
- R. Li, C. Zhao, B. Yao, D. Li, S. Yan, K. E. O'Shea and W. Song, Photochemical transformation of aminoglycoside antibiotics in simulated natural waters, Environ. Sci. Technol., 2016, 50, 2921–2930 CrossRef CAS PubMed.
- K. H. Wammer, A. R. Korte, R. A. Lundeen, J. E. Sundberga, M. Kristopher and W. A. Arnold, Direct photochemistry of three fluoroquinolone antibacterials: norfloxacin, ofloxacin, and enrofloxacin, Water Res., 2013, 47, 439–448 CrossRef CAS PubMed.
- Y. Chen, C. Hu, J. Qu and M. Yang, Photodegradation of tetracycline and formation of reactive oxygen species in aqueous tetracycline solution under simulated sunlight irradiation, J. Photochem. Photobiol., A, 2008, 197, 81–87 CrossRef CAS.
- J. Jeong, W. Song, W. J. Cooper, J. Jung and J. Greaves, Degradation of tetracycline antibiotics: Mechanisms and kinetic studies for advanced oxidation/reduction processes, Chemosphere, 2010, 78, 533–540 CrossRef CAS PubMed.
- J. Niu, Y. Li and W. Wang, Light-source-dependent role of nitrate and humic acid in tetracycline photolysis: Kinetics and mechanism, Chemosphere, 2013, 92, 1423–1429 CrossRef CAS PubMed.
- K. McNeill and S. Canonica, Triplet state dissolved organic matter in aquatic photochemistry: reaction mechanisms, substrate scope, and photophysical properties, Environ. Sci.: Processes Impacts, 2016, 18, 1381–1399 RSC.
- J. Wenk, U. von Gunten and S. Canonica, Effect of dissolved organic matter on the transformation of contaminants induced by excited triplet states and the hydroxyl radical, Environ. Sci. Technol., 2011, 45, 1334–1340 CrossRef CAS PubMed.
- Y. Zhang, Q. Xie, G. Sun, K. Yang, S. Song, J. Chen, C. Zhou and Y. Li, Effects of dissolved organic matter on phototransformation rates and dioxin products of triclosan and 2′-HO-BDE-28 in estuarine water, Environ. Sci.: Processes Impacts, 2016, 18, 1177–1184 RSC.
- X. Jin, H. Xu, S. Qiu, M. Jia, F. Wang, A. Zhang and X. Jiang, Direct photolysis of oxytetracycline: Influence of initial concentration, pH and temperature, J. Photochem. Photobiol., A, 2017, 332, 224–231 CrossRef CAS.
- J. J. Werner, W. A. Arnold and K. McNeill, Water hardness as a photochemical parameter: Tetracycline photolysis as a function of calcium concentration, magnesium concentration, and pH, Environ. Sci. Technol., 2006, 40, 7236–7241 CrossRef CAS PubMed.
- Y. Li, X. Qiao, Y. Zhang, C. Zhou, H. Xie and J. Chen, Effects of halide ions on photodegradation of sulfonamide antibiotics: Formation of halogenated intermediates, Water Res., 2016, 102, 405–412 CrossRef CAS PubMed.
- Y. Gao, M. Q. Yan and G. V. Korshin, Effects of ionic strength on the chromophores of dissolved organic matter, Environ. Sci. Technol., 2015, 49, 5905–5912 CrossRef CAS PubMed.
- R. Dalrymple, A. K. Carfagno and C. M. Sharpless, Correlations between dissolved organic matter optical properties and quantum yields of singlet oxygen and hydrogen peroxide, Environ. Sci. Technol., 2010, 44, 5824–5829 CrossRef CAS PubMed.
- A. C. Maizel and C. K. Remucal, The effect of probe choice and solution conditions on the apparent photoreactivity of dissolved organic matter, Environ. Sci.: Processes Impacts, 2017, 19, 1040–1050 RSC.
- K. M. Parker, J. J. Pignatello and W. A. Mitch, Influence of ionic strength on triplet-state natural organic matter loss by energy transfer and electron transfer pathways, Environ. Sci. Technol., 2013, 47, 10987–10994 CrossRef CAS PubMed.
- Y. Yang and J. J. Pignatello, Participation of the halogens in photochemical reactions in natural and treated waters, Molecules, 2017, 22(10), 1684 CrossRef PubMed.
- K. Zhang and K. M. Parker, Halogen radical oxidants in natural and engineered aquatic systems, Environ. Sci. Technol., 2018, 52(17), 9579–9594 CrossRef CAS PubMed.
- S. A. Timko, M. Gonsior and W. J. Cooper, Influence of pH on fluorescent dissolved organic matter photo-degradation, Water Res., 2015, 85, 266–274 CrossRef CAS PubMed.
- C. M. Glover and F. L. Rosario-Ortiz, Impact of halides on the photoproduction of reactive intermediates from organic matter, Environ. Sci. Technol., 2013, 47(24), 13949–13956 CrossRef CAS PubMed.
- K. M. Parker, E. S. Reichwaldt, A. Ghadouani and W. A. Mitch, Halogen radicals promote the photodegradation of microcystins in estuarine systems, Environ. Sci. Technol., 2016, 50(16), 8505–8513 CrossRef CAS PubMed.
- Z. Qiang and C. Adams, Potentiometric determination of acid dissociation constants (pKa) for human and veterinary antibiotics, Water Res., 2004, 38, 2874–2890 CrossRef CAS PubMed.
- A. L. Boreen, W. A. Arnold and K. McNeill, Photochemical fate of sulfa drugs in the aquatic environment: sulfa drugs containing five-membered heterocyclic groups, Environ. Sci. Technol., 2004, 38, 3933–3940 CrossRef CAS PubMed.
- J. E. Grebel, J. J. Pignatello and W. A. Mitch, Sorbic acid as a quantitative probe for the formation, scavenging and steady-state concentrations of the triplet excited state of organic compounds, Water Res., 2011, 45, 6535–6544 CrossRef CAS PubMed.
- D. E. Latch, B. L. Stender, J. L. Packer, W. A. Arnold and K. McNeill, Photochemical fate of pharmaceuticals in the environment: cimetidine and ranitidine, Environ. Sci. Technol., 2003, 37, 3342–3350 CrossRef CAS PubMed.
- A. Ter Halle and C. Richard, Simulated solar light irradiation of mesotrione in natural waters, Environ. Sci. Technol., 2006, 40, 3842–3847 CrossRef CAS PubMed.
- S. Canonica, B. Hellrung and J. Wirz, Oxidation of Phenols by Triplet Aromatic Ketones in Aqueous Solution, J. Phys. Chem. A, 2000, 104, 1226–1232 CrossRef CAS.
- S. Zhang, J. Chen, X. Qiao, L. Ge, X. Cai and G. Na, Quantum chemical investigation and experimental verification on the aquatic photochemistry of the sunscreen 2-phenylbenzimidazole-5-sulfonic acid, Environ. Sci. Technol., 2010, 44, 7484–7490 CrossRef CAS PubMed.
- Y. Zhang, J. Wang, J. Chen, C. Zhou and Q. Xie, Phototransformation of 2,3-dibromopropyl-2,4,6-tribromophenyl ether (DPTE) in natural waters: Important roles of dissolved organic matter and chloride ion, Environ. Sci. Technol., 2018, 52, 10490–10499 CrossRef CAS PubMed.
- A. Franco, A. Ferranti, C. Davidsen and S. Trapp, An unexpected challenge: ionizable compounds in the REACH chemical space, Int. J. Life Cycle Assess., 2010, 15, 321–325 CrossRef CAS.
- D. Vione, D. Fabbri, M. Minella and S. Canonica, Effects of the antioxidant moieties of dissolved organic matter on triplet-sensitized phototransformation processes: Implications for the photochemical modeling of sulfadiazine, Water Res., 2017, 128, 38–48 CrossRef PubMed.
- G. J. Janz, B. G. Oliver, G. R. Lakshminarayanan and G. E. Mayer, Electrical conductance, diffusion, viscosity, and density of sodium nitrate, sodium perchlorate, and sodium thiocyanate in concentrated aqueous solutions, J. Phys. Chem., 1970, 74, 1285–1289 CrossRef CAS.
- K. M. Parker and W. A. Mitch, Halogen radicals contribute to photooxidation in coastal and estuarine waters, Proc. Natl. Acad. Sci. U. S. A., 2016, 113, 5868–5873 CrossRef CAS PubMed.
- Q. Zhao, H. Zhao, X. Quan, S. Chen and Y. Zhang, Photochemical transformation of 2,2',4,4'-tetrabromodiphenyl ether (BDE-47) in surface coastal waters: Effects of chloride and ferric ions, Mar. Pollut. Bull., 2014, 86, 76–83 CrossRef CAS PubMed.
- T. Beitz, W. Bechmann and R. Mitzner, Investigations of reactions of selected azaarenes with radicals in water. 2. Chlorine and bromine radicals, J. Phys. Chem. A, 1998, 102, 6766–6771 CrossRef CAS.
- C. Zhou, J. Chen, H. Xie, Y. Zhang, Y. Li, Y. Wang, Q. Xie and S. Zhang, Modeling photodegradation kinetics of organic micropollutants in water bodies: A case of the Yellow River estuary, J. Hazard. Mater., 2018, 349, 60–67 CrossRef CAS PubMed.
- C. Bian, W. Jiang, J. G. Richard and H. Ding, The suspended sediment concentration distribution in the Bohai Sea, Yellow Sea and East China Sea, J. Ocean Univ. China, 2013, 12(3), 345–354 CrossRef.
Footnote |
† Electronic supplementary information (ESI) available. See DOI: 10.1039/c8em00473k |
|
This journal is © The Royal Society of Chemistry 2019 |