Consequential life cycle assessment of carbon capture and utilization technologies within the chemical industry†
Received
20th March 2019
, Accepted 8th May 2019
First published on 8th May 2019
Abstract
Carbon capture and utilization is a promising approach to reduce greenhouse gas emissions and fossil resource depletion in the chemical industry. However, since carbon capture and utilization is an energy and material intensive process, it is unclear whether it allows for a net reduction of environmental impacts from a life cycle perspective. Previous life cycle assessment studies on carbon capture and utilization focused on the production of one specific chemical or the comparison of C1 basic chemicals and applied an attributional approach. This study assesses twelve CO2-conversion technologies to provide decision support on the potential life-cycle environmental impacts of each technology. Consequential life cycle assessment was chosen as the modeling approach to better understand the system-wide environmental consequences of introducing carbon capture and utilization technologies in the chemical industry. This study has identified that in a near- and a long-term scenario the global warming impact for all CO2 conversions technologies, besides dimethoxymethane, electrochemically produced formic acid, and Fischer–Tropsch production, is negative. Formic acid produced via hydrogenation and polyol production are the conversion technologies with the highest potential for reducing the global warming impact from a life cycle perspective. Holistically polyol production is the conversion technology with the highest potential for reducing environmental impacts. In general, it seems recommendable to introduce carbon capture and utilization within the chemical industry from an environmental perspective.
Broader context
As climate change is one of the major challenges for humankind, climate-mitigating strategies are needed. Carbon capture and utilization is a strategy which is supposedly climate mitigating. Carbon capture comprises the capturing of CO2 from point sources, such as coal-fired power plants. Utilization of CO2 comprises the valorization of CO2 by for instance hydrogenation to methanol. Thus, carbon capture and utilization allows one to decrease direct emissions of point sources and provision of chemicals. However, carbon capture and utilization is an energy demanding strategy. First, capturing CO2 needs, when using amine scrubbing, thermal energy, which leads to additional greenhouse gas emissions or a decrease in the efficiency of a plant. Second, the valorization of CO2 is difficult due to its inertness and is an energy intensive process as well. Therefore the CO2 must be activated by a reducing agent. Energy-intensive water electrolysis produces the widely used reducing agent H2, which also could hamper the environmental performance of carbon capture and utilization. Besides the possibility of climate mitigation, carbon capture and utilization is a promising strategy for the chemical industry to shift from crude oil based chemistry to CO2 based chemistry.
|
Introduction
Carbon capture and utilization (CCU) is a promising approach for reducing emissions of greenhouse gases and depletion of fossil resources, especially in the chemical industry. The potential as a climate mitigation practice for CCU is also mentioned in the recently published report from the Intergovernmental Panel on Climate Change.1 CCU comprises “both industrial capture to obtain concentrated CO2, and separate functional utilization of this CO2”.2 The functional utilization contains the direct utilization of CO2, for example in carbonated beverages, or CO2 conversion into more complex chemicals, like polymers.2 However, previous studies have questioned whether CCU provides an environmental benefit, from a life cycle perspective.2–4
Existing life cycle assessments (LCA) of CCU investigate the environmental impacts of the production of a chemical, for example, C1 basic chemicals like carbon monoxide,5 syngas,6,7 formic acid,5,8,9 methane6,7,10–16 and methanol.5,6,12–14,17–19 Other LCAs focused on the assessment of more complex molecules produced via CCU, like methyl propionate,20 DME,11,19,21 DMC,17,22 OME,23 (jet) fuels,24,25 and polymers.4,14,21,26,27 A recurrent conclusion from these studies is that emissions of greenhouse gases are reduced when producing the chemicals via CCU while using electricity from renewable resources compared to fossil-based production. However, in one study22 this is not the case although best-case estimates were taken. Two studies5,14 compared different CCU technologies and concluded that the production of formic acid5 and methane14 achieves the highest environmental impact reductions compared to their conventional production.
Additionally, review studies have been published which comprise the main findings of the environmental assessment of CCU technologies.28–30 Artz et al.28 conclude that already today production of chemicals with CCU technologies reduces global warming impacts (GWI) compared to conventional production of chemicals from fossil resources. This is the case for CO2-based polyol production and formic acid production. Cuéllar-Franca and Azapagic29,30 summarize that the utilization of CO2 for producing DMC could lower the GWI compared to the conventional production by 4.3 times. Moreover, specific methodological guidance on how to conduct LCA for CCU technologies has been developed.2,31
A common trait of previous LCA studies of CCU is that they apply an attributional approach, for example, they use the partitioning method to solve the multi-functionality of the CO2-supply activity and use an average mix of suppliers for each activity. Attributional models focus on retrospectively attributing impacts to the activities of a product's life cycle based on a normative approach. Instead, consequential models are prospective as they attempt to model as closely as possible the consequences of future decisions. Therefore, consequential LCA appears better suited to the assessment of emerging technologies like CCU, as also mentioned in the previous literature.4,32 Hence, this study aims to compare CO2 conversion technologies (cf.Fig. 1), which was to the best of our knowledge not done before.
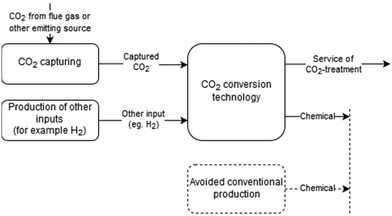 |
| Fig. 1 Generalized scheme of the investigated product system. | |
Method
The functional unit chosen for the comparative analysis is the treatment of 1 kg of CO2. This allows comparing all CO2-conversion technologies. A functional unit based on the input H2 would exclude a broad range of conversion technologies from the comparison, such as electrochemical conversion or CO2-incorporation technologies.6 The analysis was carried out via openLCA 1.7.333 and Brightway234 software using background data from the ecoinvent database 3.4 consequential.35 The scope of the study is limited to German conditions because CCU is already implemented for polyurethane and methane production in Germany. In Germany, several chemical production sites exist and when changing the raw material from fossil-based materials to CO2 decision support is needed. Hence, if available, we have used background data from Germany. Otherwise, we use data on a European or global background. The details of the life cycle inventory (LCI) are provided in the ESI.† The study focuses on the GWI of different CCU technologies. However, results are as well provided for the midpoint impact categories (IC) freshwater and terrestrial acidification, freshwater ecotoxicity, freshwater eutrophication, ionising radiation, marine eutrophication, terrestrial eutrophication, carcinogenic effects, human health (non-carcinogenic effects), human health (ozone layer depletion), photochemical ozone creation, respiratory effects, land use and mineral, fossils and renewables. Results are calculated using the International Life Cycle Data system 1.0.8 (2016) life cycle impact assessment method.36
Fig. 1 shows the structure of the product system under analysis. CO2 from flue gas or any other emitting source is captured and utilized together with other compounds (like H2 or natural gas) for the CO2 conversion. The determining product and main function of the CO2 conversion technologies are the service of treating CO2. The conversion technologies produce then chemicals (like methanol) as dependent co-products. Applying the substitution method, the marginal production of the same amount of an identical chemical is avoided. This is in-line with ISO 14040/44, which standardizes LCA practices and recommends to apply system expansion when subdivision of processes is not possible.37
CO2-Conversion technologies under study
The CO2-conversion technologies were chosen according to their technology readiness level (TRL), based on their potential to substitute the crude oil-based production of a specific chemical with its CO2-based production alternative, and the quality of data. Technologies with a TRL lower than three were excluded. Production of urea is excluded since the commercial production technology is already based on CO2 as a feedstock. Data on technologies available solely on the lab-scale were also excluded. Table S2 (cf. file CCU-SI.docx, ESI†) contains a comprehensive summary of CO2-conversion technologies containing the TRL, reaction parameters and catalysts. The chosen technologies are explained briefly in the following.
Carbon monoxide (CO).
Carbon monoxide is mostly utilized with H2 as syngas for the production of chemical intermediates (e.g., methanol).38 Conventionally carbon monoxide is produced by gasification of coal, steam reforming or partial oxidation of hydrocarbons.38 The investigated CO2-based production technologies of carbon monoxide in this article are the reverse water gas shift (rWGS) reaction39 and dry reforming of methane (DRM).40
CO2 + H2 ⇌ CO + H2O (rWGS) |
CH4 + CO2 ⇌ 2H2 + 2CO (DRM) |
Formic acid (FA).
Due to its acidity, reducing properties and aldehydic nature, formic acid is applied in a wide range of application fields. European formic acid is mostly used in the feed industry and as a silage aid.41 The potassium salt of formic acid is used on a large scale for the de-icing of aircraft. Today mainly two production technologies are applied for producing formic acid. Methyl formate hydrolysis on the one and preparation of free formic acid from formates on the other hand.41 For the CO2-based reaction to formic acid, two technologies are considered. Firstly, formic acid production via electrochemical reduction of CO28,9,42 and, secondly, the hydrogenation of CO2 is considered.43
CO2 + 2H+ + 2e− → HCOOH (electrochemical reduction (elec)) |
CO2 + H2 ⇌ HCOOH (hydrogenation (hydro)) |
Methanol (MeOH).
Methanol is commercially produced from synthesis gas containing CO and H2.44,45 As an intermediate product methanol is used in a variety of chemical syntheses, mostly for the production of formaldehyde, methyl tert-butyl ether and acetic acid.44 CO2-Based production technologies include the hydrogenation of CO2, which is investigated further.19,46
CO2 + 3H2 ⇌ CH3OH + H2O (hydrogenation) |
Methane (CH4).
Natural gas mainly contains methane with a molar fraction of up to 0.99.47 Conventionally, natural gas is extracted either from reservoirs containing oil and gas or from reservoirs containing only gas.48 Natural gas can be used either as a fuel or as a feedstock in the chemical industry. The application of natural gas as a fuel comprises utilization as a source of heat within residential, commercial and industrial applications. The largest chemical treatment is the production of synthesis gas containing carbon monoxide and H2.48 The investigated technology is based on the so-called power-to-gas or power-to-methane technology and produces a methane-rich gas which is also called synthetic or substitute natural gas.49
CO2 + 4H2 ⇌ CH4 + 2H2O (Sabatier-reaction) |
Ethanol (EtOH).
Fermentation accounts for 90% of the total ethanol production share whereas 10% of the total ethanol production is produced synthetically from ethylene or as a by-product of specific industrial processes.50 Ethanol from fermentation is traditionally used for beverages and specialty chemicals whereas ethanol from chemical synthesis is used for industrial purposes.51 CO2-Based ethanol is produced from CO2 and H2O via synthesis gas using co-electrolysis52 and by fermentation of CO2 containing syngas in particular in steel mills.
Dimethyl ether (DME).
Commercially DME is produced by the dehydration of methanol in the presence of acidic catalysts.53,54 Around 70% of DME is used in the aerosol industry as a propellant and the remaining 30% is used for the production of dimethyl sulfate.53 The chosen CO2-based technology is the direct production of DME via synthesis gas and methanol as intermediates.11
CO2 + CH4 + 2H2 ⇌ CH3OCH3 + H2O |
Dimethyl carbonate (DMC).
Currently, DMC is mostly produced by the Eni process through oxidative carbonylation of methanol, which replaced the traditional manufacturing production of DMC using toxic phosgene.22 The application fields of DMC are the synthesis of pharmaceuticals, agricultural chemicals, dyestuffs and usage as a specialty solvent.55 There are a variety of CO2-based production technologies for DMC.22,28 In this article we consider the direct synthesis from CO2 and methanol to form DMC.56
Dimethoxymethane (DMM).
Conventionally DMM is produced via acid-catalyzed condensation of formaldehyde and methanol.57 The main applications of DMM are as a precursor to several polymers, as a solvent, and as an ion exchange resin.28 CO2-based DMM production starts from H2 and CO2 and methanol as a solvent.23
Fischer–Tropsch (FT)-products.
The FT-process enables the direct production of liquid hydrocarbons using CO2 and H2.28 Depending on the reaction temperature of the FT-process the product composition changes. With high-temperature FT-processes, fuels can be produced.58 In this article, we consider an FT-process which produces jet fuel (kerosene) with naphtha as a by-product.24
nCO2 + (3n + 1)H2 → CnH2n+2 + 2nH2O |
nCO + (2n + 1)H2 → CnHn+2 + nH2O |
Polyols.
Polyether polyols conventionally are produced by a synthesis of propylene oxide, glycerol, and monopropylene glycol.59 Polyols are used for the production of polyurethane foams and potentially as transparent films and elastomers.28,60 CO2-based polyether polyol is formed similarly to the conventional synthesis; however, propylene oxide is partly substituted by CO2.27
Marginal suppliers
An LCI model built according to the consequential approach61 should reflect the consequences of a specific decision.62 Only suppliers that are affected by the decision should be included in the system. These are called marginal suppliers, technically defined as suppliers that can respond to a marginal increase in demand for the product.63 In order to identify the marginal suppliers for CO2 and H2, we followed the five-step-procedure explained in Weidema et al.63
Carbon dioxide.
CO2 can be either captured from point sources or the atmosphere.2 CO2-point sources are stationary industrial processes such as fermentation, H2 production or combustion of fossil fuels.64 Additionally CO2 could be obtained from the atmosphere via so-called direct air capture.65 CO2 is currently used in applications such as a feedstock, chilling agent, and solvent in industrial applications like food and beverage manufacturing, pharmaceutical manufacturing, polymer and chemical synthesis, pH control, welding, solvent extraction, and heat pumps.
For the supply of CO2, a distinction must be made between merchant and captive markets. In the merchant market suppliers sell their CO2 on the free market. In industrialized countries, CO2 for the merchant market is mostly captured as a by-product from the production of ammonia, H2, ethanol, ethylene oxide, and titanium dioxide in high purity. CO2 is captured from the flue gases of fossil fuel combustion only in pilot plant facilities.66 On the captive market, the supplier and buyer of a commodity are usually owned by the same entity or distribute the commodity in bulk to a niche market.66
According to Supekar and Skerlos (2014),66 the current capacity of merchant CO2 exceeds the demand and many high-purity sources are currently operating without CO2 capture and could supply additional demand for CO2 in the future. In the near-term, a marginal increase in the demand of CO2 will be satisfied by high-purity sources currently operating without capture of CO2. In the long-term, a marginal increase in CO2 demand will be satisfied via the installation of additional plants for CO2 capture. Thus, this study considers two scenarios for CO2 supply: near-term and long-term. According to Naims (2016), the demand in the near-term could be up to 250 Mt and in the long-term up to 2300 Mt of CO2.67 Aligning the respective costs for captured CO2 depending on the point sources and the potential capturable CO2 emissions per year leads to the CO2 cost curve in Fig. 2 reproduced from Naims (2016). Matching with the amount of the near- and long-term scenarios leads to the marginal suppliers for each scenario. For the near-term scenario, the marginal supply of CO2 is from fermentation,66 bioenergy,68 production of H266 and production of ammonia.66 For the long-term scenario, the marginal supply of CO2 is from the production of ammonia,66 iron and steel,69 ethylene70 and cement.71 The relative share of each supplier in the total supply (marginal mix) is calculated based on the potentially suppliable amount of CO2. For the marginal supplier in the long-term scenario, we assume that emitting sources considered in the near-term will not be able to supply any more CO2 since their capacity will be already fully utilized. The composition of the marginal supply mix for captured-CO2 is provided in Table S3 (cf. file CCU-SI.docx, ESI†).
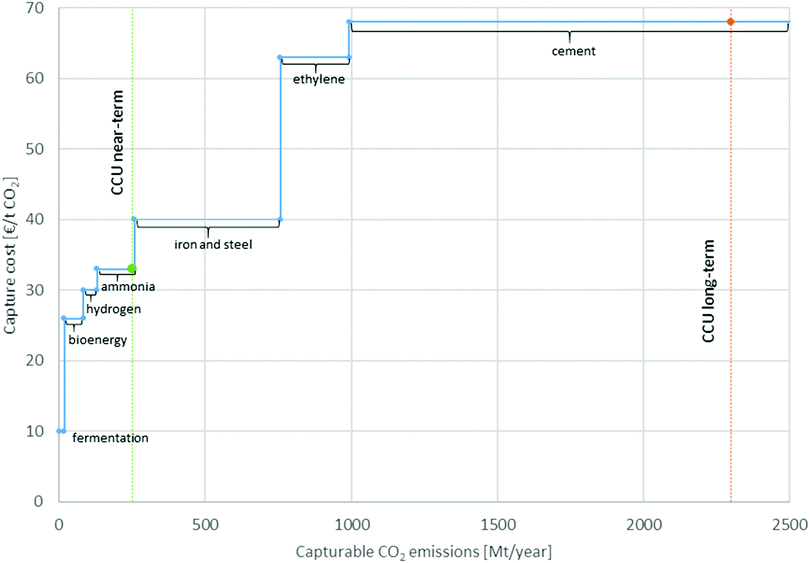 |
| Fig. 2 CO2 supply and demand scenarios without fossil-fired power generation, adopted from Naims (2016).67 | |
Hydrogen.
H2 is one of the essential raw materials in the chemical industry and is handled in reactions either by addition, also called hydrogenation, or as a reducing agent.72 H2 is utilized for the production of ammonia, methanol, hydrocarbons, and oxo synthesis products. Besides these applications, H2 is applied in refineries for hydrotreating and hydrocracking.72 The latter is the enabling technology for converting heavy and sour crude oil as well as for maximizing the yield of the middle distillate from vacuum gas oil produced in a refinery.
The market for H2 is separated into the captive and merchant H2 supply.72 Captive H2 is produced on-site and directly consumed for ammonia or methanol production of refineries. Merchant H2 is sold on the free market and accounts for 10% of the total H2 production.72 Globally the H2 supply is composed of natural gas steam reforming (50%), oil reforming (30%), coal gasification (18%), chloralkali electrolysis (3.9%) and other sources (0.1%).73
For H2 production, near- and a long-term scenarios are considered. For the near-term scenario, we assumed the default marginal supplier of H2 from the ecoinvent database 3.4 consequential. In this dataset, H2 is produced via cracking of fossil fuels. H2 produced via chloralkali electrolysis is constrained and cannot fulfill an increased demand for H2. Consequently, H2 production via chloralkali-electrolysis is not considered in the chosen dataset.35 For the long-term scenario, we assumed that the production of H2 changes to a production technology which is operated with renewable materials. Hence, we identified water electrolysis via a proton exchange membrane (PEM) as the H2-supplier for the long-term scenario. For the production of 1 kg H2 54.6 kW h electricity and 9.1 kg water are needed.74 As a by-product, 8 kg oxygen (O2) is produced and substitutes the conventional production of O2via cryogenic air separation.35,74
Background system.
Electricity and heat are the main auxiliary materials needed in the CO2 conversion process. To model the related production activities, we used data from the ecoinvent database 3.4 consequential. The marginal German market mix was used to model the electricity supply; this mix is composed primarily of electricity from wind (75%) and natural gas (19%). As a heat supplier, we chose the market of heat supply from steam within the chemical industry. Data on the energy demand for each technology under analysis were retrieved from the literature. The authors of these previous studies had either calculated such energy demand with simulation tools (Aspen Plus, CHEMCA, UniSim), or approximated it by the Gibbs free energy, or taken the value from the literature. Finally, we used background market activities to model the production of additional auxiliary materials needed for the CO2-based chemical production (natural gas, steam, water, refrigerant, and methanol) and to model the avoided production of conventional chemicals. Detailed information on all background datasets used in the model can be retrieved from the inventory data (cf. file CCU-LCI.xlsx in the ESI†).
Assumptions for sensitivity and uncertainty analysis
Sensitivity analysis.
The uncertainty associated with the modeling choices was investigated by changing assumptions that have or are expected to have a strong influence on the results. As given in the LCA literature, supply of electricity and heat is a significant contributor to the total impact of CCU.5,7,10,13,14,16,22–25,75 For the electricity supply, we assumed the marginal German market mix as the default provider and tested how the results changed when assuming that the provision of electricity is solely from lignite (as a worst case) and solely from wind (as a best case). For the heat supply, we assumed the provision of heat within the chemical industry as the default provider. Then we tested how the results changed according to changes of the heat supply. We varied between heat suppliers from central or small-scale combined heat and power plants with and without natural gas as a fuel and heat supply from district or industrial sources with and without natural gas as a fuel.
Uncertainty analysis.
The uncertainty associated with the results which depend on the uncertainty of the background model parameters was quantified by applying error propagation via Monte Carlo simulation. Values of parameter uncertainty taken directly from ecoinvent database 3.4 consequential were assumed for background exchanges, whereas no uncertainty values were used for foreground exchanges as the focus was on understanding the uncertainties related to the use of background data, while the significant uncertainties in the foreground system were addressed via sensitivity analysis (cf. the previous section). Characteristic results were calculated for each technology with 1000 iterations with dependent sampling.76 Statistical pairwise analysis of the differences between technologies was conducted via the pairwise Wilcoxon Rank Sum test as in Henriksson et al..77 The Monte Carlo simulation was performed using the Brightway2 open source LCA software.34 The statistical analysis was conducted in the R Statistical Environment.78 All code used in this study is either available on request to the author or openly available online.79
Results and discussion
Global warming impact of CO2-conversion technologies
Fig. 3 shows the GWI for the technologies under analysis in the near- and the long-term scenarios. All technologies result in carbon savings except for the electrochemically produced formic acid, production of FT products, and the DMM production in the long-term. Formic acid produced via hydrogenation and polyol production show the lowest GWI. However, the difference between formic acid (hydrogenation) and the polyol technology is not significant when considering the uncertainty (cf. the Uncertainty analysis section). Nevertheless, these two production technologies are substantially better than the remainder.
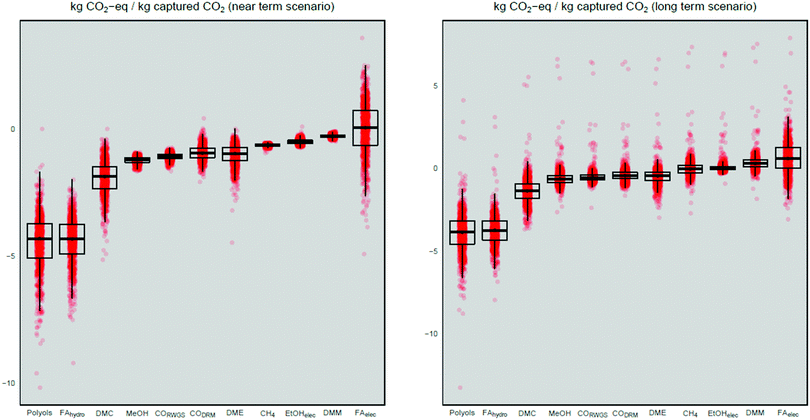 |
| Fig. 3 Global warming impact of CO2-conversion technologies (results for FT are excluded here for readability reasons and can be accessed in the ESI†). | |
The results can be explained by the ratio of CO2 to H2 needed for producing formic acid. While 1 mol CO2 and H2 is needed to form formic acid, the ratio of CO2 to H2 for methane production is less efficient, as 4 mol of energy-intense produced H2 and 1 mol of CO2 are needed to form 1 mol of methane. Additionally, the formation of formic acid is the only technology for producing basic chemicals where no by-products are produced. In all other production technologies, water is obtained as a by-product, i.e., when producing 1 mol of methane 2 mol of water are formed, which is an indication of a less efficient conversion than the conversion to formic acid. In the case of formic acid conversion, the avoided marginal production has a higher GWI (−4.52 vs. −4.49 kg CO2-eq per kg captured CO2) compared to other avoided marginal production. This is in accordance with the results of previous studies.5 However, the electrochemical production of formic acid leads to a positive GWI due to the high thermal energy demand (35.79 kg CO2-eq per kg captured CO2) needed for the distillation of the low-concentration formic acid solution (4.5 wt%) obtained from the electrochemical reactor. For the polyol production, the reason for the low GWI compared to the other CO2 based technologies is that CO2 is incorporated in the polymer. Hence, CO2 incorporation avoids the production of a high impact chemical, which is the case for the polyol production in the marginal production technology. These findings are in accordance with results from a previous study.28
All results for the long-term scenario lead to higher impacts than those for the near-term scenario, but the ranking of technologies did not change. This increase in impacts is due to the different marginal market mix used in the long-term scenario for the supply of CO2. In the long-term, CO2-sources where CO2 is more diluted are included in the suppliers’ mix. Hence, the CO2-capturing process in the long-term has higher energy-intensity and higher impacts compared to the high-purity CO2 sources of the near-term mix. The GWI for the marginal market mix of CO2 for the near-term is −0.80 kg CO2-eq per kg captured CO2 (mean = −0.80; standard deviation = 0.01; median = −0.79) and for the long-term −0.34 kg CO2-eq per kg captured CO2 (mean = −0.35; standard deviation = 0.06; median = −0.34). This contradicts the idea of postponing CCU since CO2 capture pursued today would allow emitting fewer greenhouse gases compared to future capture. In the long-term scenario, H2 is supplied by PEM water electrolysis. The GWI for the marginal market mix is 1.70 kg CO2-eq per kg H2 (mean = 1.70; standard deviation = 0.00; median = 1.70) and for the PEM in the long term is 1.90 kg CO2-eq per kg H2 (mean = 1.97; standard deviation = 1.06; median = 1.93). Hence, the impacts of CCU technologies using H2 are slightly higher due to the higher impacts of H2 production in the long-term. Still for all but the production of DMM, electrochemically produced formic acid and FT-products in the long-term CCU is an option leading to negative GWI.
Contribution analysis
The activity with the most significant contribution to the GWI is the avoided production of chemicals (see Fig. 4). This is the case for nine technologies in the near and ten technologies in the long-term scenario and especially for technologies producing formic acid, polyols, and DMC. The supply of CO2 is the second largest contributor to the total GWI of each technology. Electricity and heat generation are the third largest contributor to the total GWI. The provision of H2, which is mentioned in the literature as one of the major contributors for CCU-systems, has a rather low impact within our study. This is explained by the fact that in the near-term the H2 is supplied by the market mix of H2 and in the long-term H2 supply via PEM electrolysis is assumed. The electrolysis – although based on energy-intensive water splitting – utilizes electricity supplied from the marginal electricity mix, which is primarily composed of electricity from wind (75%) and natural gas (19%). H2-Supply is, thus, a rather low greenhouse gas emitting process.
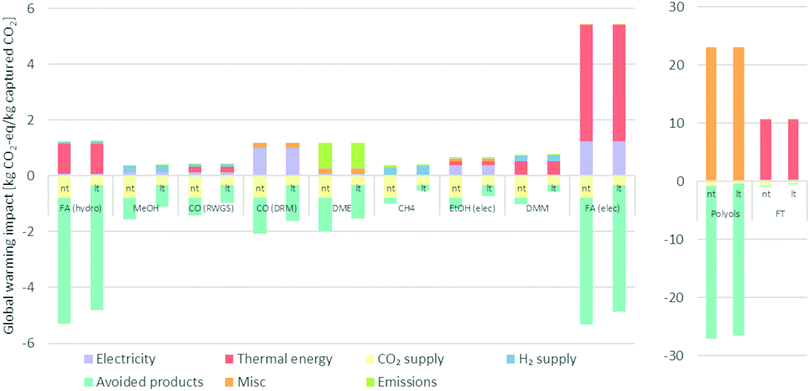 |
| Fig. 4 Contribution analysis for the global warming impact of CO2-conversion technologies (divided into two graphs due to different scales). | |
Further environmental impacts of CO2-conversion systems
Analyzing the correlation of the GWI with the other impacts shows the categories where further analysis is needed. The values of the correlation analysis and the rankings of each technology in each IC are provided in the ESI† (cf. file CCU-LCIA.xlsx). A high correlation (R2 > 0.93) was observed for GWI and freshwater, terrestrial acidification, freshwater, marine, and terrestrial eutrophication, as well as photochemical ozone creation. These categories are not further discussed as they show the same ranking of the technologies as the GWI. The observed correlation between GWI and other IC was lower (R2 < 0.93) and therefore the ranking of the technologies changed for those IC compared to the GWI. Thus these IC need to be discussed further. Compared to the climate change impact category, a substantially different ranking of alternatives and of process contributions was observed for the case of freshwater ecotoxicity, ecosystem quality, human health (ionizing radiation) and resources (minerals, fossils and renewables).
The production of electricity is the largest contributor to the freshwater ecotoxicity impact and this contribution can be traced back to the construction of windmills and from there to the market for scrap copper. Copper is hazardous and therefore a substance which leads to ecotoxicity impacts. Moreover, impacts from the avoided products contribute to the ecotoxicity impact. For the impact of ionizing radiation on ecosystem quality, the contribution from CO2 is lower compared to GWI and the contribution from the avoided product is higher compared to GWI. The impacts of ionizing radiation on human health are influenced by the avoided production. This is the case for all technologies except the conversion to produce methane. In the case of the conversion technology for producing methane, the main contributing activity is the CO2 supply. Impacts on resources (mineral, fossils, and renewables) are directly dependent on the impact of the avoided product. Additionally, for the conversion technology to produce polyols the provision of the needed input materials like propylene oxide is the other main contributor (47% of the overall impact). In summary, polyol production remains the most promising option for CCU as the average ranking is the highest rank compared to the other CO2-conversion technologies.
Difference between the near- and long-term scenarios
The ranking for the technologies changed for 13 IC from the near- to the long-term scenario. However, for the GWI and marine eutrophication, the ranking was still the same as for the near term. As only the processes for CO2- and H2-supply were changed, differences in the ranking between scenarios are traced back to those processes. Substantial changes in the ranking of the technologies compared to the near term are observed for the IC ecotoxicity, due to the high influence of the electricity mix for producing H2 in the long term. Another IC for which different rankings are observed in each scenario is human health (ionizing radiation). This is explained by the fact that CO2-supply in the long term has a lower impact on human health (ionizing radiation) and thus the impact on ionizing radiation is reduced drastically for all technologies using H2. That is why for instance the treatment of CO2 to produce CH4 changed from the 6th best technology to the most favored technology considering human health (ionizing radiation).
Sensitivity analysis
We changed the electricity and heat sources in the near-term to understand how this modeling choice affects the results (cf.Table 1). The results show that they are more sensitive to the choice of electricity supplier than to the choice of heat supplier. For ten out of twelve technologies the GWI is at least 10% higher or lower compared to the default scenario (near-term) when changing the electricity supplier. In the case of changing the heat supply, six out of twelve technologies indicate at least 10% higher or lower GWI compared to the default scenario. However, there are also technologies which are more sensitive to changes in heat supplier than in electricity supplier. This is the case for the conversion technologies to CO via rWGS, DMM, EtOH, formic acid via hydrogenation and FT-products. The GWI for conversion technologies to produce CH4, CO via DRM, DME, formic acid via electrochemical reduction and MeOH is sensitive to changing the electricity supplier. The GWI for CO2-conversion to DMC is sensitive to changing the heat supplier.
Table 1 Sensitivity analysis for heat and electricity supply; absolute values for the deviation from the default in kg CO2-eq
|
CH4 |
CODRM |
COdwgs |
DMC |
DME |
DMM |
EtOHdec |
FAdec |
FAhycfro |
FT |
MeOH |
Polyol |
Heat supply |
Central or small-scale, natural gas |
−0.01 |
0.00 |
−0.08 |
−0.32 |
−0.01 |
−0.19 |
−0.06 |
−1.47 |
−0.39 |
−3.61 |
−0.01 |
−0.01 |
Central or small-scale |
−0.03 |
0.01 |
−0.21 |
−0.84 |
−0.03 |
−0.49 |
−0.17 |
−3.87 |
−1.02 |
−9.53 |
−0.03 |
−0.03 |
District or industrial, natural gas |
0.01 |
0.00 |
0.08 |
0.33 |
0.01 |
0.19 |
0.06 |
1.50 |
0.39 |
3.69 |
0.01 |
0.01 |
District or industrial |
0.01 |
0.00 |
0.08 |
0.32 |
0.01 |
0.19 |
0.06 |
1.49 |
0.39 |
3.66 |
0.01 |
0.01 |
|
Electricity supply |
Lignite |
0.26 |
8.40 |
1.20 |
0.14 |
0.64 |
0.28 |
3.32 |
10.13 |
0.72 |
2.20 |
1.14 |
0.21 |
Wind |
−0.03 |
−0.85 |
−0.12 |
−0.01 |
−0.06 |
−0.03 |
−0.34 |
−1.03 |
−0.07 |
−0.22 |
−0.12 |
−0.02 |
Interestingly, for the conversion technology producing polyols, the GWI is insensitive to changes in the electricity or heat supplier. This is because the main contributing processes to the overall GWI for polyol production are the provision of the feedstocks besides CO2 (46%) and the avoided burden for substituting the marginal production of the polyols (52%). Consequently, the contribution to the overall GWI is rather low for the supply of heat and electricity when producing polyols.
Uncertainty analysis
The testing for differences between alternatives shows that due to the uncertainties in the background data some comparisons were not statistically significant. In the near-term scenario, we cannot conclude that the treatment of CO2 to produce polyols is significantly different from the treatment of CO2 to produce formic acid by hydrogenation (p = 1). Another non-significant difference is between CO2 conversion into dimethyl ether and carbon monoxide via DRM (p = 0.410). Similarly, in the long-term scenario, we cannot find a significant difference between the treatment of CO2 to produce polyols and the treatment of CO2 to produce formic acid by hydrogenation (p = 0.749) and between CO2 conversion into dimethyl ether and carbon monoxide (p = 1). Additionally in the long-term scenario we cannot conclude that the impact of the treatment of CO2 to produce ethanol is significantly different from the impact of the treatment of CO2 to produce methane (p > 0.0001) and there is no observed strong difference between CO2 conversion into dimethyl ether and carbon monoxide via rWGS (p = 0.0000116). It can, therefore, be concluded with strong confidence that technologies for producing formic acid and polyols have a significantly lower global warming impact than the other technologies. Full results of the statistical testing are provided in the ESI† (cf. file CCU-UA.xlsx).
Study scope, assumptions, and validity
The validity of these results should be discussed in light of the scope of the study and its main assumptions. The study focused on CCU technologies with a TRL equal to or higher than three, replacing a fossil-based production technology, and with data available beyond the lab scale. However, new data on lower tier technologies might be available in the near future, as conversion technologies currently experience substantial development. This new evidence could be used to improve the current analysis possibly leading to different conclusions. Data on technologies with a lower TRL than three were deemed too uncertain to be used in this comparative context, and appropriate techniques, for example technology upscaling, should be applied when using such data, but this was beyond the scope of the current study.80 New or systemic process designs for CCU technologies, for example by including heat integration or downstream processing, could, for example, change our results drastically (e.g., building a plant which only uses solar energy in this specific case).
Additionally, the study did not focus on the impacts generated from the production of catalysts and infrastructure, since the contribution of such activities is expected to be rather small compared to the impacts coming from primary inputs like electricity, heat, and CO2 supply.81
Within the study, the best available knowledge was applied to identify the marginal suppliers. As there is yet no established market for CO2, the identification of marginal supplier for CO2, especially in the long-term, is dependent on several assumptions. However, a change in the CO2 marginal supplier would change the results of all technologies and therefore should not change the overall conclusions. Additionally, the choice to assess H2 from PEM as a marginal supplier was motivated by pragmatic reasons, given that market studies forecasting future H2 suppliers are scarce. Despite these assumptions, this study offers insights into the environmental assessment of CCU technologies which are in line with results of previous studies5,28 and extend current CCU technology knowledge.
Conclusions
This study determined the life-cycle impacts of CO2 conversion technologies to support the choice between different CO2 conversion technologies from an environmental perspective and with a particular focus on climate-related impacts. The main highlight is that a negative GWI was observed for all CO2 conversion technologies besides DMM, electrochemically produced formic acid, and FT production, in both a near- and a long-term scenario. The research has also shown that formic acid produced via hydrogenation and polyol production are the CCU conversion technologies with the highest reduction potential in terms of GWI. The second significant finding was that holistically polyol production is the CO2 conversion technology with the highest potential for reducing the climate impact. Based on these findings it seems recommendable to introduce CCU within the chemical industry from an environmental perspective. This research is the first comprehensive investigation of comparing CO2 conversion technologies within a broad scope and lays the ground for future work in the field of environmental assessment of CCU.
Additionally, the study contributes to our understanding of the environmental consequences of introducing CO2 conversion technologies by assessing the environmental impacts with the consequential LCA approach. The question raised by this study is a rather general question on the introduction of CCU in the chemical industry. Further research should, therefore, determine environmental impacts by using a consequential LCA approach on a site-specific basis (e.g., environmental assessment of an existing CCU pilot plant).
Abbreviations
CCU | Carbon capture and utilization |
DMC | Dimethyl carbonate |
DME | Dimethyl ether |
DMM | Dimethoxymethane |
DRM | Dry reforming of methane |
elec | Electrochemical reduction |
EtOH | Ethanol |
FA | Formic acid |
FT | Fischer–Tropsch |
GWI | Global warming impact |
hydro | Hydrogenation |
IC | Impact category |
LCA | Life cycle assessment |
LCI | Life cycle inventory |
MeOH | Methanol |
PEM | Proton exchange membrane |
rWGS | Reverse water gas shift |
ESI | Electronic supplementary information |
TRL | Technology readiness level |
Conflicts of interest
There are no conflicts to declare.
Acknowledgements
The authors thank Prof. Bo Weidema for his contribution to the initial idea and the study design of this article. Additionally, the authors thank Tim Schulzke and Dr Axel Kraft for their helpful comments on the assessed chemical conversion technologies.
References
- Intergovernmental Panel on Climate Change (IPCC), Global Warming of 1.5 °C. An IPCC special report on the impacts of global warming of 1.5 °C above pre-industrial levels and related global greenhouse gas emission pathways, in the context of strengthening the global response to the threat of climate change, sustainable development, and efforts to eradicate poverty, 2018.
- N. von der Assen, J. Jung and A. Bardow, Energy Environ. Sci., 2013, 6, 2721–2734 RSC.
-
N. von der Assen, A. M. L. Lafuente, M. Peters and A. Bardow, in Carbon dioxide utilisation. Closing the carbon cycle, ed. P. Styring, E. A. Quadrelli and K. Armstrong, Elsevier, Amsterdam, Boston, Heidelberg, 2015, pp. 45–56 Search PubMed.
- N. von der Assen, P. Voll, M. Peters and A. Bardow, Chem. Soc. Rev., 2014, 43, 7982–7994 RSC.
- A. Sternberg, C. M. Jens and A. Bardow, Green Chem., 2017, 19, 2244–2259 RSC.
- A. Sternberg and A. Bardow, Energy Environ. Sci., 2015, 8, 389–400 RSC.
- A. Sternberg and A. Bardow, ACS Sustainable Chem. Eng., 2016, 4, 4156–4165 CrossRef CAS.
- A. Dominguez-Ramos, B. Singh, X. Zhang, E. G. Hertwich and A. Irabien, J. Cleaner Prod., 2015, 104, 148–155 CrossRef CAS.
- M. Rumayor, A. Dominguez-Ramos and A. Irabien, Appl. Sci., 2018, 8, 914–925 CrossRef.
- P. Collet, E. Flottes, A. Favre, L. Raynal, H. Pierre, S. Capela and C. Peregrina, Appl. Energy, 2017, 192, 282–295 CrossRef CAS.
- W. B. Schakel, G. D. Oregionni, B. Singh, A. H. Strømman and A. Ramírez, J. CO2 Util., 2016, 16, 138–149 CrossRef CAS.
- V. Uusitalo, S. Väisänen, E. Inkeri and R. Soukka, Energy Convers. Manage., 2017, 134, 125–134 CrossRef CAS.
- W. Hoppe, S. Bringezu and N. Thonemann, J. Cleaner Prod., 2016, 121, 231–237 CrossRef CAS.
- W. Hoppe, N. Thonemann and S. Bringezu, J. Ind. Ecol., 2018, 22, 327–340 CrossRef CAS.
- G. Reiter and J. Lindorfer, Int. J. Life Cycle Assess., 2015, 20, 477–489 CrossRef CAS.
- D. Parra, X. Zhang, C. Bauer and M. K. Patel, Appl. Energy, 2017, 193, 440–454 CrossRef.
- M. Aresta and M. Galatola, J. Cleaner Prod., 1999, 7, 181–193 CrossRef.
-
(a) P. Biernacki, T. Röther, W. Paul, P. Werner and S. Steinigeweg, J. Cleaner Prod., 2018, 191, 87–98 CrossRef CAS;
(b) J. Kim, C. A. Henao, T. A. Johnson, D. E. Dedrick, J. E. Miller, E. B. Stechel and C. T. Maravelias, Energy Environ. Sci., 2011, 4, 3122–3132 RSC.
- M. Matzen and Y. Demirel, J. Cleaner Prod., 2016, 139, 1068–1077 CrossRef CAS.
- S. C. Stouten, A. Anastasopoulou, V. Hessel and Q. Wang, React. Chem. Eng., 2017, 2, 688–695 RSC.
- C. Fernández-Dacosta, V. Stojcheva and A. Ramírez, J. CO2 Util., 2018, 23, 128–142 CrossRef.
- I. Garcia-Herrero, R. M. Cuéllar-Franca, V. M. Enríquez-Gutiérrez, M. Alvarez-Guerra, A. Irabien and A. Azapagic, ACS Sustainable Chem. Eng., 2016, 4, 2088–2097 CrossRef CAS.
- S. Deutz, D. Bongartz, B. Heuser, A. Kätelhön, L. Schulze Langenhorst, A. Omari, M. Walters, J. Klankermayer, W. Leitner, A. Mitsos, S. Pischinger and A. Bardow, Energy Environ. Sci., 2018, 11, 331–343 RSC.
- C. Falter, V. Batteiger and A. Sizmann, Environ. Sci. Technol., 2016, 50, 470–477 CrossRef CAS PubMed.
- C. van der Giesen, R. Kleijn and G. J. Kramer, Environ. Sci. Technol., 2014, 48, 7111–7121 CrossRef CAS PubMed.
- N. von der Assen, A. Sternberg, A. Kätelhön and A. Bardow, Faraday Discuss., 2015, 183, 291–307 RSC.
- C. Fernández-Dacosta, M. W. van der Spek, C. R. Hung, G. D. Oregionni, R. Skagestad, P. Parihar, D. T. Gokak, A. H. Strømman and A. Ramírez, J. CO2 Util., 2017, 21, 405–422 CrossRef.
- J. Artz, T. E. Müller, K. Thenert, J. Kleinekorte, R. Meys, A. Sternberg, A. Bardow and W. Leitner, Chem. Rev., 2018, 118, 434–504 CrossRef CAS PubMed.
-
R. M. Cuéllar-Franca and A. Azapagic, in Encyclopedia of Sustainable Technologies, ed. M. Abraham, Elsevier, Amsterdam, 2017, pp. 447–459 Search PubMed.
- R. M. Cuéllar-Franca and A. Azapagic, J. CO2 Util., 2015, 9, 82–102 CrossRef.
-
A. W. Zimmermann, J. Wunderlich, G. Buchner, L. J. Müller, K. Armstrong, S. Michailos, A. Marxen, H. Naims, F. Mason, G. Stokes and E. Williams, Techno-Economic Assessment & Life Cycle Assessment. Guidelines for CO2 Utilization, 2018 Search PubMed.
- R. Aldaco, I. Butnar, M. Margallo, J. Laso, M. Rumayor, A. Dominguez-Ramos, A. Irabien and P. E. Dodds, Sci. Total Environ, 2019, 663, 738–753 CrossRef CAS PubMed.
- A. Ciroth, Int. J. Life Cycle Assess., 2007, 12, 209–210 CrossRef.
- C. L. Mutel, J. Open Source Software, 2017, 2, 236–237 CrossRef.
- G. Wernet, C. Bauer, B. Steubing, J. Reinhard, E. Moreno-Ruiz and B. P. Weidema, Int. J. Life Cycle Assess., 2016, 21, 1218–1230 CrossRef.
- European Commission-Joint Research Centre (EC-JRC), International Reference Life Cycle Data System (ILCD) Handbook. Recommendations for Life Cycle Impact Assessment in the European context. Based on existing environmental impact assessment models and factors, EUR 24571 EN, Luxemburg, 2011.
-
(a) ISO, Environmental management – Life cycle assessment – Requirements and guidelines, 2006, vol. 13.020.10, 13.020.10;
(b) ISO, Environmental management – Life cycle assessment – Principles and framework, 2009, vol. 13.020.10, 13.020.10.
-
J. Bierhals, Ullmann's encyclopedia of industrial chemistry, Wiley, Chichester, 2010, vol. 6, pp. 679–693 Search PubMed.
- Y. A. Daza and J. N. Kuhn, RSC Adv., 2016, 6, 49675–49691 RSC.
- M.-S. Fan, A. Z. Abdullah and S. Bhatia, ChemCatChem, 2009, 1, 192–208 CrossRef CAS.
-
J. Hietala, A. Vuori, P. Johnsson, I. Pollari, W. Reutemann and H. Kieczka, Ullmann's encyclopedia of industrial chemistry, Wiley, Chichester, 2010, pp. 1–22 Search PubMed.
- C. Oloman and H. Li, ChemSusChem, 2008, 1, 385–391 CrossRef CAS.
-
(a) M. Pérez-Fortes, J. C. Schöneberger, A. Boulamanti, G. Harrison and E. Tzimas, Int. J. Hydrogen Energy, 2016, 41, 16444–16462 CrossRef;
(b) C.-L. Chiang, K.-S. Lin and H.-W. Chuang, J. Cleaner Prod., 2018, 172, 1957–1977 CrossRef CAS.
-
J. Ott, V. Gronemann, F. Pontzen, E. Fiedler, G. Grossmann, D. B. Kersebohm, G. Weiss and C. Witte, Ullmann's encyclopedia of industrial chemistry, Wiley, Chichester, 2010, pp. 1–27 Search PubMed.
-
A. English, J. Brown, J. Rovner and S. Davies, in Encyclopedia of chemical technology, ed. R. E. Kirk and D. F. Othmer, Wiley, New York, NY, 2003, pp. 1–19 Search PubMed.
-
(a) L. K. Rihko-Struckmann, A. Peschel, R. Hanke-Rauschenbach and K. Sundmacher, Ind. Eng. Chem. Res., 2010, 49, 11073–11078 CrossRef CAS;
(b) M. Pérez-Fortes, J. C. Schöneberger, A. Boulamanti and E. Tzimas, Appl. Energy, 2016, 161, 718–732 CrossRef;
(c) É. S. Van-Dal and C. Bouallou, Chem. Eng. Trans., 2012, 29, 463–468 Search PubMed;
(d)
M. Aresta, A. Caroppo, A. Dibenedetto and M. Narracci, in Environmental Challenges and Greenhouse Gas Control for Fossil Fuel Utilization in the 21st Century, ed. M. M. Maroto-Valer, C. Song and Y. Soong, Springer, Boston, 2002, pp. 331–347 Search PubMed;
(e) A. A. Kiss, J. J. Pragt, H. J. Vos, G. Bargeman and M. T. de Groot, Chem. Eng. J., 2016, 284, 260–269 CrossRef CAS;
(f) H. Al-Kalbani, J. Xuan, S. García and H. Wang, Appl. Energy, 2016, 165, 1–13 CrossRef CAS.
-
G. Hammer, T. Lübcke, R. Kettner, M. R. Pillarella, H. Recknagel, A. Commichau, H.-J. Neumann and B. Paczynska-Lahme, Ullmann's encyclopedia of industrial chemistry, Wiley, Chichester, 2010, vol. 23, pp. 739–792 Search PubMed.
-
K. E. Woodcock and M. Gottlieb, in Encyclopedia of chemical technology, ed. R. E. Kirk and D. F. Othmer, Wiley, New York, NY, 2003, pp. 365–386 Search PubMed.
- K. Ghaib and F.-Z. Ben-Fares, Renewable Sustainable Energy Rev., 2018, 81, 433–446 CrossRef CAS.
-
J. E. Logsdon, in Encyclopedia of chemical technology, ed. R. E. Kirk and D. F. Othmer, Wiley, New York, NY, 2003, pp. 1–72 Search PubMed.
-
N. Kosaric, Z. Duvnjak, A. Farkas, H. Sahm, S. Bringer-Meyer, O. Goebel and D. Mayer, in Ullmann's encyclopedia of industrial chemistry, Wiley, Chichester, 2010, vol. 13, pp. 1–72 Search PubMed.
- Y. E. Fouih and C. Bouallou, Energy Procedia, 2013, 37, 6679–6686 CrossRef CAS.
-
M. Müller and U. Hübsch, in Ullmann's encyclopedia of industrial chemistry, Wiley, Chichester, 2010, vol. 11, pp. 305–308 Search PubMed.
-
P. R. Worsham, in Encyclopedia of chemical technology, ed. R. E. Kirk and D. F. Othmer, Wiley, New York, NY, 2003, pp. 1–22 Search PubMed.
-
S. B. Damle, in Encyclopedia of chemical technology, ed. R. E. Kirk and D. F. Othmer, Wiley, New York, NY, 2003, pp. 1–10 Search PubMed.
- F. F. T. de Groot, R. R. G. J. Lammerink, C. Heidemann, M. P. M. van der Werff, T. C. Garcia, L. A. G. J. van der Ham and H. van den Berg, Chem. Eng. Trans., 2014, 39, 1561–1566 Search PubMed.
- X. Zhang, S. Zhang and C. Jian, Chem. Eng. Res. Des., 2011, 89, 573–580 CrossRef CAS.
-
A. de Klerk, in Encyclopedia of chemical technology, ed. R. E. Kirk and D. F. Othmer, Wiley, New York, NY, 2003, pp. 1–36 Search PubMed.
- N. von der Assen and A. Bardow, Green Chem., 2014, 16, 3272–3280 RSC.
-
(a) M. A. Subhani, B. Köhler, C. Gürtler, W. Leitner and T. E. Müller, Polym. Chem., 2016, 7, 4121–4126 RSC;
(b) M. A. Subhani, B. Köhler, C. Gürtler, W. Leitner and T. E. Müller, Angew. Chem., Int. Ed., 2016, 55, 5591–5596 CrossRef CAS PubMed.
- T. Ekvall and B. P. Weidema, Int. J. Life Cycle Assess., 2004, 9, 161–171 CrossRef.
- B. P. Weidema, M. Pizzol, J. Schmidt and G. Thoma, J. Cleaner Prod., 2018, 174, 305–314 CrossRef.
- B. P. Weidema, N. Frees and A.-M. Nielsen, Int. J. Life Cycle Assess., 1999, 4, 48–56 CrossRef.
-
J. Gale, in IPCC special report on carbon dioxide capture and storage, ed. B. Metz, Cambridge Univ. Press, Cambridge, 2005, pp. 75–104 Search PubMed.
-
(a) C. Gebald, J. A. Wurzbacher, P. Tingaut, T. Zimmermann and A. Steinfeld, Environ. Sci. Technol., 2011, 45, 9101–9108 CrossRef CAS PubMed;
(b) A. Kumar, D. G. Madden, M. Lusi, K.-J. Chen, E. A. Daniels, T. Curtin, J. J. Perry and M. J. Zaworotko, Angew. Chem., Int. Ed., 2015, 54, 14372–14377 CrossRef CAS PubMed;
(c) K. S. Lackner, Energy, 2013, 50, 38–46 CrossRef CAS.
- S. D. Supekar and S. J. Skerlos, Environ. Sci. Technol., 2014, 48, 14615–14623 CrossRef CAS PubMed.
- H. Naims, Environ. Sci. Pollut. Res., 2016, 23, 22226–22241 CrossRef CAS.
- K. Möllersten, J. Yan and J. R. Moreira, Biomass Bioenergy, 2003, 25, 273–285 CrossRef.
-
H. D. Choi, Master thesis, Norwegian University of Science and Technology, 2013.
-
M. C. Weikl and G. Schmidt, Carbon Capture in Cracking Furnaces, AIChE Paper 12c, San Antonio, 2010 Search PubMed.
- D. García-Gusano, D. Garraín, I. Herrera, H. Cabal and Y. Lechón, J. Cleaner Prod., 2015, 104, 328–338 CrossRef.
-
P. Häussinger, R. Lohmüller and A. M. Watson, Ullmann's encyclopedia of industrial chemistry, Wiley, Chichester, 2010, vol. 19, pp. 353–392 Search PubMed.
- I. Dincer and C. Acar, Int. J. Hydrogen Energy, 2015, 40, 11094–11111 CrossRef CAS.
- A. Mehmeti, A. Angelis-Dimakis, G. Arampatzis, S. McPhail and S. Ulgiati, Environments, 2018, 5, 24–42 CrossRef.
-
(a) O. G. Griffiths, R. E. Owen, J. P. O'Byrne, D. Mattia, M. D. Jones and M. C. McManus, RSC Adv., 2013, 3, 12244–12254 RSC;
(b) X. Zhang, C. Bauer, C. L. Mutel and K. Volkart, Appl. Energy, 2017, 190, 326–338 CrossRef CAS.
-
(a) P. J. G. Henriksson, J. B. Guinée, R. Heijungs, A. de Koning and D. M. Green, Int. J. Life Cycle Assess., 2014, 19, 429–436 CrossRef;
(b) P. Lesage, C. L. Mutel, U. Schenker and M. Margni, Int. J. Life Cycle Assess., 2018, 23, 2248–2265 CrossRef.
- P. J. G. Henriksson, A. Rico, W. Zhang, S. Ahmad-Al-Nahid, R. Newton, L. T. Phan, Z. Zhang, J. Jaithiang, H. M. Dao, T. M. Phu, D. C. Little, F. J. Murray, K. Satapornvanit, L. Liu, Q. Liu, M. M. Haque, F. Kruijssen, G. R. de Snoo, R. Heijungs, P. M. van Bodegom and J. B. Guinée, Environ. Sci. Technol., 2015, 49, 14176–14183 CrossRef CAS PubMed.
- The R Development Core Team, R: A Language and Environment for Statistical Computing. Reference Index, 2008.
-
M. Pizzol, massimopizzol/CCU-LCA. Github repository, DOI:10.5281/zenodo.2652361.
-
(a) M. Caduff, M. A. J. Huijbregts, H.-J. Althaus and A. J. Hendriks, Environ. Sci. Technol., 2011, 45, 751–754 CrossRef CAS PubMed;
(b) L. Valsasina, M. Pizzol, S. Smetana, E. Georget, A. Mathys and V. Heinz, J. Cleaner Prod., 2017, 142, 2209–2217 CrossRef.
- M. Rumayor, A. Dominguez-Ramos and A. Irabien, Sustainable Prod. Consumption, 2019, 18, 72–82 CrossRef.
Footnote |
† Electronic supplementary information (ESI) available. See DOI: 10.1039/c9ee00914k |
|
This journal is © The Royal Society of Chemistry 2019 |