DOI:
10.1039/C9DT01359H
(Communication)
Dalton Trans., 2019,
48, 12391-12395
Reactions of hydrazones and hydrazides with Lewis acidic boranes†
Received
29th March 2019
, Accepted 19th June 2019
First published on 9th August 2019
Abstract
The reaction of (diphenylmethylene)hydrazone or 1,4-bis-hydrazone-ylidene(phenylmethyl)benzene with Lewis acidic boranes B(2,4,6-F3C6H2)3 or B(3,4,5-F3C6H2)3 generates the Lewis acid–base adducts. Alternatively, when (9H-fluoren-9-ylidene)hydrazone is employed several products were isolated including 1,2-di(9H-fluoren-9-ylidene)hydrazone, the 2
:
1 borane adduct of NH2–NH2 and the 1-(diarylboraneyl)-2-(9H-fluoren-9-ylidene)hydrazone in which one ArH group has been eliminated. The benzhydrazide starting material also initially gives an adduct when reacted with Lewis acidic boranes which upon heating eliminates ArH generating a CON2B heterocycle.
With over a century since the dawn of the Haber–Bosch process, the activation and subsequent functionalisation of dinitrogen remains a challenge. Typically, metal catalysts, such as those based upon Fe or Mo, have been trialled for the reduction of N2 to NH3. The ability of transition metal complexes to bind to N2 has been attributed to the roles of the metal d-orbitals in bonding and back-bonding interactions with N2.1 Recently however, the activation of nitrogen–nitrogen bonds such as azides, diazo-compounds, hydrazones and dinitrogen using boranes has gained momentum.2,3 Both Szymczak4 and Simonneau5 reported the use of B(C6F5)3 to activate M–N2 (M = Fe, Mo, W) complexes (Fig. 1) towards protonation or borylation/silylation. A major advance in this area was to remove the transition metal altogether. While (N2)BF3 has been generated via supersonic expansion at 600 Torr and 170 K,6 it was only last year that the metal-free activation of N2 was reported using a cyclic (alkyl)(amino)carbene (CAAC) stabilised borylene (Fig. 1).7 Prior to this, Stephan3a,8 had shown that the strong Lewis acid B(C6F5)3 can be used to release N2 from Ph2CN2, and can be used in reactions with Ph2C
NNH2 to form an adduct (Fig. 1). Yamashita9 has subsequently shown that a highly Lewis acidic diborane can react with azobenzene and pyridazine via diboration and, when phthalazine is employed, N
N bond cleavage occurs. At a similar time, we have been investigating the synthesis and comparative reactivity of new boranes bearing fluorinated aryl groups as an alternative to the archetypical B(C6F5)3.10 In this regard, we were interested in comparing the reactivity of boranes other than B(C6F5)3 with a variety of N–N bonded compounds including hydrazones and hydrazides, which are reported herein (Fig. 1). These may provide alternative reactivity and reaction rates depending upon the borane Lewis acidity.11 Initially, the hydrazone compounds 1a–c (Schemes 1 and 2) were prepared from the condensation reaction of the corresponding ketone with hydrazine monohydrate. Upon reaction of 1a with B(2,4,6-F3C6H2)3 or B(3,4,5-F3C6H2)3 the corresponding N → B adducts 2a and 2b were formed in which the –NH2 group of the hydrazone coordinated to the Lewis acidic boron atom analogous to that reported by Stephan when 1a was combined with B(C6F5)3.8 Similarly, when bis-hydrazone 1b was reacted with B(2,4,6-F3C6H2)3 in a 1
:
2 stoichiometric ratio a 1
:
2 adduct (2c) was formed. Compounds 2a, 2b and 2c were isolated in 61%, 65% and 56% yield respectively. Adducts 2a–c all displayed a broad peak ranging between −2.6 and −6.2 ppm in the 11B NMR spectrum. Recrystallisation of the reaction mixtures from a CH2Cl2/pentane solution resulted in single crystals of the adducts whose structures were determined by single crystal X-ray diffraction (Fig. 2). In these structures the boron atom adopted a tetrahedral arrangement with B–N bond lengths ranging from 1.646(5)–1.696(4) Å, similar to those observed in previous reports (1.631(7) Å).7 The single N–N bond lengths (range 1.438(4)–1.449(2) Å) were also similar to those reported previously.8 Likewise, the C
N bond lengths of 1.287(3)–1.294(5) Å are typical of C
N double bonds.12 In all cases, heating adducts 2 resulted in an unidentifiable mixture of compounds and no elimination of ArFH was observed, in contrast with previous reports where B(C6F5)3 was employed as the Lewis acid.8 Alternatively, if the compound 1c was used in the reactions with boranes BArF3 (ArF = 3,4,5-F3C6H2, C6F5), the reaction gave a complex and inseparable mixture of products. However, if 1c is reacted with the less Lewis acidic borane B(2,4,6-F3C6H2) several products could be selectively crystallised from the reaction mixture to shed light on this reactivity. These could be structurally identified by single crystal X-ray diffraction as the previously reported 1,2-di(9H-fluoren-9-ylidene)hydrazone (3),13 the bis borane adduct of hydrazone, NH2NH2 (4) and the 1-(diarylboraneyl)-2-(9H-fluoren-9-ylidene)hydrazone (5) (Scheme 2 and Fig. 3). It is unclear how the three products are formed from the reaction, but it is likely that initially an adduct is formed between 1c and the borane. Indeed, in situ NMR studies showed that when 1c is added to the borane in toluene then a characteristic broad shift at δ = −5.1 ppm in the 11B NMR spectrum was observed. Two divergent pathways then operate to generate either 3 and 4, or compound 5. Elimination of ArFH generates 5 which is similar to observations made by Stephan for hydrazone Ph2C
NNH2 with B(C6F5)3.8 The trigonal planar boron atom in 5 can be observed in the solid-state structure with N–B–C bond angles of 117.8(1)° and 119.7(1)°, and a C–B–C bond angle of 122.5(1)°. The B–N bond length of 1.397(2) Å was also significantly shorter than observed in the adducts 2 indicating some π-donation from N → B generating a trans-diene structure in which the CN2B atoms lie in the same plane. If the reaction of 1c with B(2,4,6-F3C6H2)3 is performed at low temperatures (0 °C) in toluene then compound 5 is formed selectively in 46% yield.
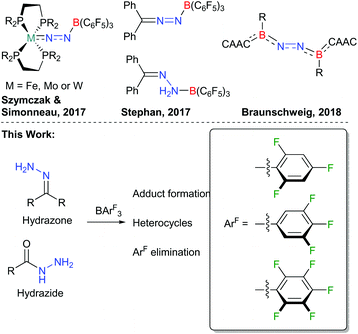 |
| Fig. 1 Previous work on activation of N–N bonds using B(C6F5)3 and borylenes, and outline of the work described in this article. | |
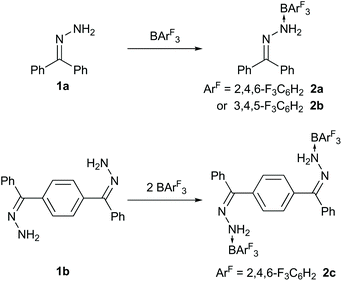 |
| Scheme 1 Reactions of hydrazones with Lewis acidic boranes. | |
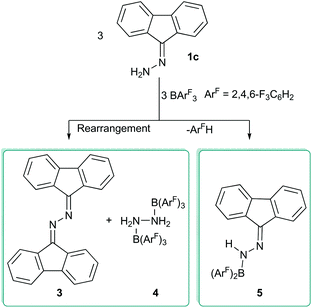 |
| Scheme 2 Reactions of 1c with B(2,4,6-F3C6H2)3. | |
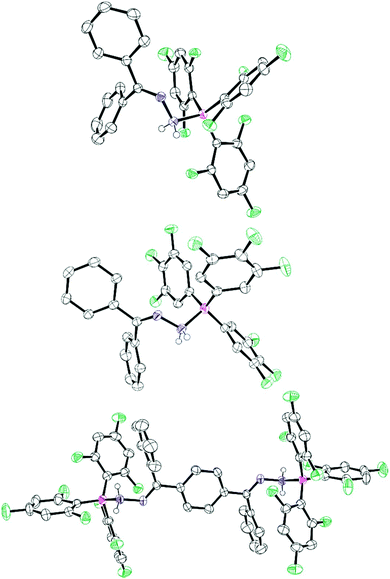 |
| Fig. 2 Solid-state structures of 2a (top), 2b (middle) and 2c (bottom). C: black, N: blue, B: pink, F: green. Thermal ellipsoids drawn at 50% probability. H atoms except N–H omitted for clarity. | |
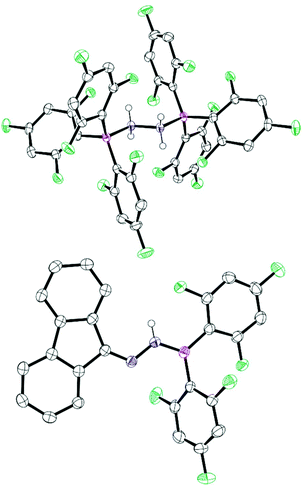 |
| Fig. 3 Solid-state structures of 4 (top) and 5 (bottom). C: black, N: blue, B: pink, F: green. Thermal ellipsoids drawn at 50% probability. H atoms except N–H omitted for clarity. | |
Alternatively, another reaction can take place when 1c is reacted with B(2,4,6-F3C6H2)3. Here a hydrazone metathesis reaction occurs to yield the azine (3) and borane adduct of hydrazone (4). This reaction is more predominant when the reaction is performed at elevated temperatures (e.g. r.t. to 50 °C). N–N-linked diimines (azines) are an important class of compounds isoelectronic to 1,3-butadiene. These compounds have a variety of uses including applications as chromophores in optoelectronic devices.14 Previously, 3 has been reported to have been synthesised by the decomposition of diazo compounds catalysed by Pt(C2H4)(PPh3)2.15 Similar reactions which generate a diazine from hydrazones have been reported and involve harsh conditions and long time periods e.g. refluxing in ethanolic hydrogen chloride for several hours.16 More interesting is that, in our case, the borane adduct of hydrazone 4 is also generated in the reaction. Previously, the hydrazine bisborane N2H4(BH3)2 has been postulated as a good hydrogen storage material containing 16.9 wt% hydrogen. Additionally, coordinated hydrazine has been identified as a potential intermediate in nitrogen fixation reactions.17–19 While N2H4(BH3)2 is easily prepared from the reaction of hydrazine sulfate with sodium borohydride, other borane adducts are more challenging to synthesise and a search of the literature reveals that generally intramolecular bis boranes are employed. Previously, Gabbaï20 and Szymczak17 have reported that intramolecular bisboranes can react with hydrazine monohydrate (N2H4·H2O). In these reactions, the use of a bisborane appears key to trapping and activating the hydrazone unit. We attempted to generate 4 independently from the reaction BArF3 (Ar = 2,4,6-F3C6H2) and hydrazine monohydrate (N2H4·H2O) in a 2
:
1 ratio with the use of molecular sieves to trap the water by-product. Pleasingly, this led to the formation of compound 4 as observed by in situ multinuclear NMR spectroscopy. The structure of 4 displays a B–N bond length of 1.673(2) Å which is longer than that in hydrazine bisborane (1.609 Å)21 and slightly shorter than that reported by Gabbaï (1.688(2) Å) and Szymczak (1.697(2) Å and 1.698(2) Å) for the chelating bisborane complexes.17,20 The N–N distance of 1.461(2) Å is similar to that reported for other hydrazine bisborane complexes (range 1.356(2) Å–1.469(2) Å).17,20,22
Finally, we turned our attention to other N–N bonded substrates, namely hydrazides (Scheme 3). When commercially available benzhydrazide was reacted with BArF3 an adduct was formed initially between the NH2 group and the borane. In the case of the boranes where Ar = 2,4,6-F3C6H2 or C6F5 the adducts 6a–b could be isolated in 56% and 71% yield, respectively. Recrystallisation of the solutions afforded crystals of the adducts which could be structurally determined (Fig. 4 and ESI†). The 11B NMR spectra showed a broad peak at δ = −6.1 ppm (6a) and −6.2 ppm (6b) similar to that observed with the hydrazone borane adducts. In the case of 6bin situ heating of the BArF3 adduct of benzhydrazide to 110 °C in toluene led to loss of ArFH and generation of a CON2B heterocycle (7). Recrystallisation of the reaction mixture afforded pink crystals of the product in 82% yield. Compound 7 crystallised in the P
space group with two molecules in the asymmetric unit. The N–H hydrogen atoms were identified in the difference map. X-ray diffraction analysis revealed the CON2B rings to be planar with r.m.s.d. of 0.010 Å and 0.023 Å. The B–N bond lengths of 1.621(2) Å and 1.608(3) Å are slightly shorter than that in the adduct 6a–b (1.635(3) Å and 1.643(2) Å respectively) presumably due to the formation of a 5-membered intramolecular chelate. Conversely, the N–N bond in 7 is slightly longer at 1.468(2) Å and 1.464(3) Å compared to 1.429(3) Å and 1.426(2) Å in 6a–b.
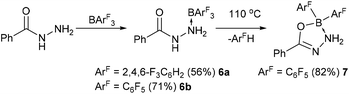 |
| Scheme 3 Reaction of hydrazides with BArF3. | |
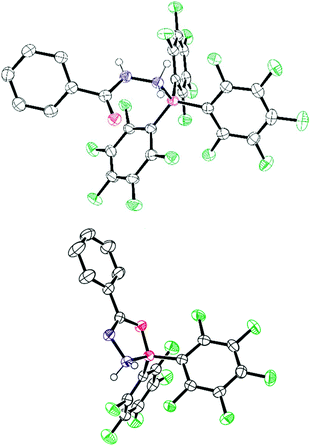 |
| Fig. 4 Solid-state structures of 6b (top) and 7 (bottom). C: black, N: blue, O: red, B: pink, F: green. Thermal ellipsoids drawn at 50% probability. Non-essential H atoms omitted for clarity. | |
Preliminary computational studies (DFT B3LYP-D3/6-31G(d,p)) to probe these elimination reactions (Scheme 3) focused on the model compound MeCONHNH2 → BPh3. Calculations revealed 1,4-elimination of C6H6 (implementing the NH2 proton) appears to be the thermodynamically favoured process and forms acyclic MeCON(H)N(H)BPh2, whereas 1,5-elimination (using the amide-NH) is a near energetically neutral process leading to formation of a 3-membered BN2 heterocycle as the initial elimination product (see ESI†).
Conclusions
In conclusion we have prepared a series of hydrazone and hydrazide adducts of Lewis acidic boranes to investigate the interactions of Lewis acidic boranes with nitrogen containing compounds which may be of interest to those working in the area of metal-free dinitrogen activation and conversion. Initially adducts are formed which upon heating lead to loss of ArFH to generate novel covalent N–N–B systems including the chain compound 5 and heterocyclic compound 7. Of particular interest is the reaction to generate the bisborane hydrazine dimer 4 from a hydrazone metathesis reaction. Subsequent studies to explore the independent synthesis and reactivity of 4 in hydrogen release are ongoing within our group.
Conflicts of interest
There are no conflicts to declare.
Acknowledgements
T. A. G. thanks the EPSRC Cardiff/Bristol/Bath CDT in Catalysis for funding (EP/L016443/1). A. D. and R. L. M. would like to acknowledge the EPSRC for an Early Career Fellowship for funding (EP/R026912/1). J. M. R. thanks NSERC for financial support.
Notes and references
-
Y. Nishibayashi, Nitrogen Fixation, Springer, New York, 2017 CrossRef CAS PubMed; K. C. MacLeod and P. L. Holland, Nat. Chem., 2013, 5, 559 CrossRef CAS PubMed; P. L. Holland, Dalton Trans., 2010, 39, 5415 RSC.
- A. J. Ruddy, D. M. C. Ould, P. D. Newman and R. L. Melen, Dalton Trans., 2018, 47, 10377 RSC.
-
(a) R. C. Neu, C. Jiang and D. W. Stephan, Dalton Trans., 2013, 42, 726 RSC;
(b) C. Schneider, J. H. W. LaFortune, R. L. Melen and D. W. Stephan, Dalton Trans., 2018, 47, 12742 RSC.
- J. B. Geri, J. P. Shanahan and N. K. Szymczak, J. Am. Chem. Soc., 2017, 139, 5952 CrossRef CAS PubMed.
- A. Simonneau, R. Turrel, L. Vendier and M. Etienne, Angew. Chem., Int. Ed., 2017, 56, 12268 CrossRef CAS PubMed.
- K. C. Janda, L. S. Bernstein, J. M. Steed, S. E. Novick and W. Klemperer, J. Am. Chem. Soc., 1978, 100, 8074 CrossRef CAS.
- M.-A. Légaré, G. Bélanger-Chabot, R. D. Dewhurst, E. Welz, I. Krummenacher, B. Engels and H. Braunschweig, Science, 2018, 359, 896 CrossRef CAS PubMed . Also see: C. Hering-Junghans, Angew. Chem., Int. Ed., 2018, 57, 6738 CrossRef PubMed; M.-A. Légaré, M. Rang, G. Bélanger-Chabot, J. I. Schweizer, I. Krummenacher, R. Bertermann, M. Arrowsmith, M. C. Holthausen and H. Braunschweig, Science, 2019, 363, 1329 CrossRef PubMed.
- C. Tang, Q. Liang, A. R. Jupp, T. C. Johnstone, R. C. Neu, D. Song, S. Grimme and D. W. Stephan, Angew. Chem., Int. Ed., 2017, 56, 16588 CrossRef CAS PubMed . Also see: R. L. Melen, Angew. Chem., Int. Ed., 2018, 57, 880 CrossRef PubMed.
- Y. Katsuma, L. Wu, Z. Lin, S. Akiyama and M. Yamashita, Angew. Chem., Int. Ed., 2019, 58, 317 CrossRef CAS PubMed.
- J. R. Lawson, L. C. Wilkins and R. L. Melen, Chem. – Eur. J., 2017, 23, 10997 CrossRef CAS PubMed; Q. Yin, Y. Soltani, R. L. Melen and M. Oestreich, Organometallics, 2017, 36, 2381 CrossRef; J. L. Carden, L. J. Gierlichs, D. F. Wass, D. L. Browne and R. L. Melen, Chem. Commun., 2019, 55, 318 RSC.
- M. Santi, D. M. C. Ould, J. Wenz, Y. Soltani, R. L. Melen and T. Wirth, Angew. Chem., Int. Ed., 2019, 58, 7861 CrossRef CAS PubMed.
-
Bond Energies, Encyclopedia of Inorganic Chemistry, 2006; DOI:10.1002/0470862106.id098.
- J. Lasri, N. E. Eltayeb and A. I. Ismail, J. Mol. Struct., 2016, 1121, 35 CrossRef CAS.
- J. Safari and S. Gandomi-Ravandia, RSC Adv., 2014, 4, 46224 RSC.
- R. Bertani, M. Biasiolo, K. Darini, R. A. Michelin, M. Mozzon, F. Visentin and L. Zanotto, J. Organomet. Chem., 2002, 642, 32 CrossRef CAS.
- M. L. Trudell, N. Fukada and J. M. Cook, J. Org. Chem., 1987, 52, 4293 CrossRef CAS.
- J. J. Kiernicki, M. Zeller and N. K. Szymczak, J. Am. Chem. Soc., 2017, 139, 18194 CrossRef CAS PubMed.
- Y. Yu, W. W. Brennessel and P. L. Holland, Organometallics, 2007, 26, 3217 CrossRef CAS PubMed; Y. Lee, N. P. Mankad and J. C. Peters, Nat. Chem., 2010, 2, 558 CrossRef PubMed; C. T. Saouma, C. C. Lu and J. C. Peters, Inorg. Chem., 2012, 51, 10043 CrossRef PubMed; K. Umehara, S. Kuwata and T. Ikariya, J. Am. Chem. Soc., 2013, 135, 6754 CrossRef PubMed; Y. Li, Y. Li, B. Wang, Y. Luo, D. Yang, P. Tong, J. Zhao, L. Luo, Y. Zhou, S. Chen, F. Cheng and J. Qu, Nat. Chem., 2013, 5, 320 CrossRef PubMed.
- T. Hügle, M. F. Kühnel and D. Lentz, J. Am. Chem. Soc., 2009, 131, 7444 CrossRef PubMed.
- C.-H. Chen and F. P. Gabbaï, Chem. Sci., 2018, 9, 6210 RSC.
- S. Mebs, S. Grabowsky, D. Förster, R. Kickbusch, M. Hartl, L. L. Daemen, W. Morgenroth, P. Luger, B. Paulus and D. Lentz, J. Phys. Chem. A, 2010, 114, 10185 CrossRef CAS PubMed.
- S. Pylypko, E. Petit, P. G. Yot, F. Salles, M. Cretin, P. Miele and U. B. Demirci, Inorg. Chem., 2015, 54, 4574 CrossRef CAS PubMed.
Footnotes |
† Electronic supplementary information (ESI) available: Experimental procedures, NMR spectra and details of the X-ray analyses of compounds 2a–c, 4–5, 6a,b, 7. CCDC 1897205–1897211 and 1903201. For ESI and crystallographic data in CIF or other electronic format see DOI: 10.1039/c9dt01359h |
‡ These authors contributed equally. |
|
This journal is © The Royal Society of Chemistry 2019 |