Rare-earth ion exchanged Cu-SSZ-13 zeolite from organotemplate-free synthesis with enhanced hydrothermal stability in NH3-SCR of NOx†
Received
30th September 2018
, Accepted 31st October 2018
First published on 26th November 2018
Abstract
The relatively low hydrothermal stability of Al-rich Cu-SSZ-13 catalysts hinders their practical application in ammonia selective catalytic reduction (NH3-SCR) reaction. Rare-earth ions were introduced into the Al-rich SSZ-13 zeolite using an organotemplate-free synthesis prior to the exchange of Cu2+ ions. Among the rare-earth ions tested (Ce, La, Sm, Y, Yb), Y shows significant enhancement of the hydrothermal stability and NH3-SCR activities after severe hydrothermal aging at 800 °C for 16 h when compared with Cu-SSZ-13 without Y. Cu–Y-SSZ-13 catalysts with various amounts of Y were prepared, and it is found that with increasing Y content, the low temperature NO conversions can be improved even after hydrothermal aging. SEM-EDX analysis together with two-dimensional multiple quantum magic-angle-spinning nuclear magnetic resonance (23Na MQ MAS NMR) confirms that the Y ions are successfully incorporated into the ion-exchange sites of the SSZ-13 zeolite. Results from 27Al MAS, 29Si MAS NMR, temperature-programmed desorption of ammonia (NH3-TPD) and quantitative 1H MAS NMR indicate that Y can stabilize the framework Al and also preserve the Brønsted acid sites in the Al-rich SSZ-13 zeolite. The hydrogen temperature programmed reduction (H2-TPR), ultraviolet-visible-near infrared spectroscopy (UV-vis-NIR) and diffuse reflectance infrared Fourier transform spectroscopy (DRIFTS) of nitric oxide (NO) or NH3 adsorption demonstrate that introduction of Y ions causes Cu2+ ions to preferentially occupy the 6-MR, which has high hydrothermal stability. However, too much of Y may lead to activity loss at both low and high temperatures. The optimized Al-rich Cu–Y-SSZ-13 with 2.8 wt% of copper (Cu) and 1.3 wt% of Y displays almost the same deNOx activities as the conventional organotemplated high silica Cu-SSZ-13 catalyst in a wide reaction temperature window of 150–650 °C after severe hydrothermal treatment. Rare-earth ions could be an effective additive for Cu-SSZ-13 catalysts to further improve their hydrothermal stability for practical applications.
1. Introduction
Ammonia selective catalytic reduction (NH3-SCR) is the most effective technique for nitrogen oxide (NOx) abatement in lean burning engine exhaust after-treatment.1 Cu-SSZ-13 has been commercialized as an NH3-SCR catalyst because of its significant advantages of excellent catalytic performance and hydrothermal stability.2–4 However, with ever more stringent restrictions on engine exhaust gases, the requirement for maximizing fuel efficiency, and especially for vehicles under cold start conditions, further enhancement of the low temperature NH3-SCR activity and hydrothermal stability of the catalyst is still highly desirable.5–7 Recently, significant advances have been made in understanding the low temperature NH3-SCR reaction mechanism on Cu-SSZ-13 zeolite, and it is recognized that low temperature activity is strongly dependent on the amount of available paired copper (Cu) ions to complete the Cu2+–Cu+ redox cycle.8–10 Indeed, higher Cu loadings will increase the probability of the formation of paired Cu ions with the increase of Cu ion densities, and will further enhance the low temperature activity. However, high silica SSZ-13 has limited ion-exchange sites, which leads to the commercialized Cu-SSZ-13 catalyst is ready to be at 100% ion exchange level to get higher Cu loadings and produce aggregated copper oxide (CuOx) species,11 and consequently sacrifices the high temperature activities. Furthermore, even with similar Cu loadings for SSZ-13 zeolites with different silicon/aluminium (Si/Al) ratios, Al-rich SSZ-13 always shows higher activity, which may benefit from the higher density of ion exchange sites and easy formation of paired Cu ions.12–15 Therefore, it is reasonable that the Si/Al ratios of the commercial Cu-SSZ-13 zeolite are optimized to be 15 to 10.16 Besides, it is found that alkaline metal ion additives such as Na+, Li+ and so on, can further enhance low temperature activity as well as the hydrothermal stability of Cu exchanged Al-rich SSZ-13 zeolite.13,17 Increasing the Al content in the SSZ-13 zeolite may be a promising method to enhance both the low and high temperature activities. In particular, the Al-rich SSZ-13 zeolite with Si/Al ratios of 4–7 can be economically synthesized using a relatively low cost structure-directing agent such as Cu2+ coordinated tetraethylenepentamine or even by using an organotemplate-free synthesis.13,18,19 The latter not only avoids using the high cost template, N,N,N-trimethyl-1-adamantanamine hydroxide, but also reduces the energy consumption and pollution from the subsequent calcination of the organic templates. However, the major problem of using the Al-rich SSZ-13 zeolite is that it has low hydrothermal stability, and the low temperature activity is significantly degraded after deep hydrothermal aging.13,19 Generally, Cu2+ ions located at 6-MR with 2Al substitution are relatively stable, whereas CuOH+ ions at the CHA cage are inclined to aggregate into CuOx or migrate to 6-MR during hydrothermal aging.16,20,21 The Al sites possessing a Brønsted acid site are vulnerable to dealumination, and therefore elevating Cu loadings within a certain range can enhance the hydrothermal stability, but too many Cu ions will result in the formation of CuOx, and further lead to permanent destruction of the zeolite structure.20 Even though these framework Al sites can also be stabilized using Na+ ions, which increase the hydrothermal stability, in some way as shown in our previous study,13 Na+ can significantly decrease the stability of the Cu ions and this side effect should never be ignored in catalyst used long-term.13,17,19 It is well known that rare-earth (RE) ions can enhance the hydrothermal stability of FAU zeolites, and these have been commercialized as the state-of-the-art fluid catalytic cracking catalysts.22,23 Additionally, RE ions with diameters of 1.05–1.15 Å can also improve the hydrothermal stability of the Fe/BEA zeolite for the NH3-SCR reaction.24 To date, very few studies on the effect of RE ions on the hydrothermal stability of Cu-SSZ-13 catalysts have been reported probably because of the fact that RE ions have relatively larger hydrated ion diameters than the pore apertures of the 8-MR windows (3.8 Å).25,26 Therefore, cerium (Ce)-exchanged Cu-SSZ-13 has no more than 1.3 wt% of Ce loading as the exchange process is carried out at relatively lower temperature of 80 °C.27 Very recently, Usui et al. reported that a very small amount of Ce (approximately 0.2–0.4 wt%) can improve the hydrothermal stability of Cu-SSZ-13, whereas higher Ce loadings obtained using solid-state ion exchange will inevitable result in cerium(IV) oxide (CeO2) aggregates.28 In fact, ion exchange of RE ions with large hydrated ion diameters can be carried out much more effectively at much higher temperatures with a prolonged exchange time.29 In this research, SSZ-13 zeolite synthesized using an organotemplate-free approach was used as the support and a series of RE ions such as Ce, lanthanum (La), samarium (Sm), ytterbium (Yb) and yttrium (Y) were successfully introduced prior to the exchange of Cu2+ ions to investigate the effect of RE ions on the hydrothermal stability of the Al-rich Cu-SSZ-13 catalyst. It is demonstrated that Y additive with the smallest ion diameter can readily enhance the hydrothermal stability of Al-rich Cu-SSZ-13. The correlation between the microstructures and hydrothermal stabilities of the resultant catalysts has been investigated using multiple characterization techniques such as X-ray diffraction (XRD), ultraviolet-visible-near infrared spectroscopy (UV-vis-NIR), DRIFTS of nitric oxide (NO) adsorption, hydrogen temperature programmed reduction (H2-TPR) and solid-state NMR spectroscopy.
2. Experimental
2.1 Catalyst preparation
The Na-SSZ-13 zeolite with a Si/Al ratio of 4 was obtained using organotemplate-free synthesis, and the preparation procedures have been previously described in detail by Zhao et al.13 NH4-SSZ-13 was produced using ion-exchange (twice) of Na-SSZ-13 with an aqueous solution of 0.1 M ammonium nitrate (NH4NO3) at 80 °C, then it was exchanged with 0.0015 M RE nitrate solution (Ce, La, Sm, Y, Yb) (pH = 3.5) in an autoclave at 130 °C for 12 h to obtain RE-SSZ-13. Then, the zeolite slurries were filtered, washed with deionized water and dried at 110 °C for 2 h to obtain RE-SSZ-13. In order to vary the Y content, NH4-SSZ-13 was exchanged with an aqueous solution of 0.00075–0.01 M yttrium(III) nitrate [Y(NO3)3] in an autoclave at 130 °C for 12 h. Then, catalysts with about 2.8 wt% Cu loading were prepared using the ion-exchange of the RE-SSZ-13 samples with an aqueous solution of 0.009 to 0.02 M of copper(II) nitrate [Cu(NO3)2, pH = 3.0] at 80 °C for 4 h. The resultant samples were calcined in static air at 550 °C for 5 h. The Cu-exchanged conventional high silica SSZ-13 (Si/Al = 5) zeolite obtained using the organotemplate synthesis was also prepared using similar procedures for comparison. These catalysts were denoted as (m)Cu–(n)RE-CHA, m and n (wt%) indicating the Cu and RE contents, respectively. All the aged catalysts were hydrothermally treated in 10% water (H2O)/air at 800 °C for 16 h, and these conditions are widely reported in the literature as being comparable to an aged catalyst from a vehicle which has done 135
000 miles.11
2.2 Catalyst characterizations
Cu and RE loadings of the resultant catalysts were measured using inductively coupled plasma atomic emission spectroscopy (ICP-AES, Optima 2000 DV, PerkinElmer, USA). Powder XRD analysis (D-Max Rotaflex X-ray diffractometer, Rigaku) was performed using Cu Kα radiation (λ = 1.5418 Å) with 2θ ranging from 5–50°. UV-vis-NIR diffuse reflectance spectra (Cary-5000, Agilent) were collected under ambient conditions from 190–1400 nm. Scanning electron microscopy-energy dispersive X-ray (SEM-EDX) analysis was carried out on a scanning electron microcopy (Nova NanoSEM 450, FEI) at a working voltage of 20 kV.
The H2-TPR experiments were conducted on a chemisorption analyzer (Finesorb-3010C, FINETEC Instruments, China). Typically, about 50 mg of catalyst sample was pretreated under pure argon (Ar) at 300 °C for 1 h and then cooled down to 30 °C. Then, the catalyst was heated to 650 °C at 10 °C min−1 in a 5% H2/Ar at a flow rate of 50 ml min−1.
Ammonia temperature programmed desorption (NH3-TPD) was carried out using the SCR reaction system, and the NH3 was monitored using an online mass spectrometer (MS, m/z = 17, OmniStar, Pfeiffer). About 35 mg of catalyst sample (40–60 mesh) was heated at 450 °C under 20% oxygen/nitrogen (O2/N2) for 30 min. When the temperature had decreased to 300 °C, the sample was cooled to 100 °C under pure N2. The NH3 in N2 (1000 ppm) was introduced at 100 °C until the NH3 adsorption reached equilibrium and then it was purged with pure N2 (100 ml min−1) for 1.5 h to remove the physically adsorbed NH3. Then, the temperature was ramped from 100 °C to 650 °C at a flow rate of 10 °C min−1.
All the solid-state MAS NMR measurements were carried out on a DD2 500 MHz spectrometer (Agilent). Proton (1H) and 27Al MAS-NMR spectra were collected using similar procedures to those previously reported by Zhao et al.,13 except that the 1H-MAS-NMR spectra were recorded using a 3.2 mm triple-resonance MAS-NMR probe at a spinning rate of 10 kHz. 1H-NMR quantification was performed using a known amount of 1,1,1,3,3,3-hexafluoro-2-propanol as the external standard.30,31 The 29Si MAS NMR spectra were acquired at 99.3 MHz using a 6 mm double-resonance MAS probe at a spinning rate of 4 kHz, 400 scans and a recycle delay of 4 s. The 29Si-NMR chemical shifts were compared to 4,4-dimethyl-4-silapentane sulfonate sodium. The 23Na MQ MAS NMR spectra were obtained using a z-filter 3Q MAS pulse sequence with a spinning rate of 10 kHz, 4800 transients with a 1 s recycle delay. The 23Na-NMR chemical shifts were compared to a 1 M sodium chloride (NaCl) aqueous solution.
The diffuse reflectance infrared Fourier transform spectroscopy (DRIFTS) experiments were conducted in an environmental temperature chamber (PIKE Technologies), using a Fourier transform infrared (FTIR) spectrometer (Nicolet iS 10, ThermoFisher) equipped with a mercury cadmium telluride detector. All the samples were dehydrated under a N2 flow at 450 °C for 30 min, and then cooled to room temperature. After the background spectra were collected, 500 ppm of NO in N2 was introduced. For the NH3 adsorption, the samples were pretreated at 450 °C in 10% O2/N2 for 30 min and subsequently cooled to 50 °C. After the background spectra were collected under pure N2, 500 ppm of NH3 in N2 was introduced. The spectra were collected in the wavenumber range of 4000–650 cm−1 by accumulating 64 scans until they became stable. OMNIC software (ThermoFisher Nicolet) was used to convert the absorbance data into the Kubelka–Munk format.
2.3 Catalytic evaluations
DeNOx tests were conducted in a fixed-bed quartz reactor with 500 ppm NH3, 500 ppm NO, 10% O2, 5% H2O in N2, as reactants, and the gas hourly space velocity (GHSV) was 80
000 h−1 or 400
000 h−1. The other experimental details are the same as in the previous paper by Zhao et al.13
3. Results
3.1 Effects of rare-earth ions and Y loadings on NH3-SCR activity
Table 1 shows the Cu and RE contents of Cu–RE-SSZ-13 catalysts obtained using ICP-AES analysis, and the degrees of ion-exchange were obtained by assuming that each Cu2+ or RE3+ was exchanged ideally with two or three ion-exchange sites produced by the framework Al. All the catalysts had 20–31% degrees of exchange for Cu2+ ions whereas there were similar degrees of exchange for RE3+ at 10–14%. The total exchange level was below 50%. Fig. 1 shows the NO conversions of Cu-SSZ-13 catalysts containing RE ions with different ionic radii, and their corresponding nitrous oxide (N2O) yields are displayed in Fig. S1 (ESI†). It should be noted that similar molar amount of RE atoms were introduced for these catalysts and the weight differences result from the different atomic masses. Introduction of RE ions had no significant impact on the low temperature activity in the fresh catalyst except that there was a minor enhancement of the low temperature NO conversion with the Ce additive. However, high temperature NO conversions were decreased for all the RE additives compared to the conversions obtained with the Cu-SSZ-13 catalyst. After the 800 °C hydrothermal aging, there was a significant decrease of low temperature NO conversions for the Cu-SSZ-13 catalyst. All the catalysts with RE additives show different degrees of enhancement of low temperature NO conversions, whereas the loss of high temperature NO conversions was observed for Ce, La, and Sm additives. For the additives with the smallest ion radius, Y and Yb, the aged Cu–Y-SSZ-13 seems to have relatively better NO conversions than the aged Cu–Yb-SSZ-13 at higher temperatures. In this research, Y was chosen to be the representative RE to be used for optimization of its content in Cu-SSZ-13 catalysts with a similar Cu loading. To exclude the direct contribution of Y on SCR activity, Y-SSZ-13 without Cu was also tested, and it exhibited low NO conversions over the whole reaction-temperature window (see Fig. S3, ESI†). This means that Y may have no SCR activity. However, Cu–Y-SSZ-13 catalysts have much better NO conversions as shown in Fig. 2 and S2 (ESI†). The low temperature NO conversions remained unchanged with Y contents up to 1.3 wt%, and an obvious decrease of low temperature NO conversions was observed when the Y content was above 2.3 wt%. At the same time, high temperature NO conversions decreased as the Y content increased. After hydrothermal aging at 800 °C, all the catalysts with the Y additive showed higher, low temperature NO conversions when compared with Cu-SSZ-13, whereas there was a more obvious decrease of high temperature NO conversions Y contents above 2.3 wt%. Fig. 3 illustrates the effect of Y content on the low temperature NO conversions for fresh and aged Cu–Y-CHA catalysts. It was clear that after hydrothermal treatment with higher Y contents, the degradation of low temperature activity was significantly inhibited, and enhancement of low temperature NO conversions was observed when the Y content was above 2.3 wt%. To further determined the effects of Y, NH3-SCR reactions of all fresh and aged Cu–Y-CHA catalysts were also conducted at a much higher GHSV of 400
000 h−1 (Fig. S4, ESI†). Similar trends were observed when compared with the reactions conducted at a lower space velocity. Again, hydrothermally aged Cu-CHA showed significantly lower NO conversions than the aged Cu–Y-CHA, but the difference between the Cu–Y-CHA catalysts was not very obvious and 2.8Cu–1.3Y-CHA still showed a slightly better performance. It is worth noting that the effect of Y on the high temperature NO conversions became less dramatic because the NH3 oxidation would be inhibited at a very high space velocity.
Table 1 Cu, rare-earth ion contents and corresponding degrees of ion-exchange for Cu–RE-CHA catalysts
Catalyst |
Cu content (wt%) |
Degree of Cu exchange (%) |
RE content (wt%) |
Degree of RE exchange (%) |
Total degree of exchange (%) |
2.8Cu-CHA |
2.8 |
25 |
— |
— |
25 |
2.8Cu–1.3Y-CHA |
2.8 |
25 |
1.3 |
13 |
38 |
2.2Cu–1.6Ce-CHA |
2.2 |
20 |
1.6 |
10 |
30 |
3.0Cu–2.7Yb-CHA |
3.0 |
27 |
2.7 |
14 |
41 |
3.4Cu–1.8La-CHA |
3.4 |
31 |
1.8 |
11 |
42 |
3.4Cu–2.3Sm-CHA |
3.4 |
31 |
2.3 |
13 |
44 |
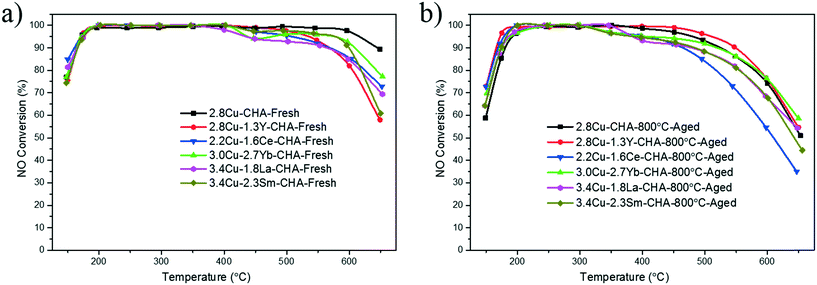 |
| Fig. 1 NO conversions as a function of temperature on various RE ion exchanged Cu-SSZ-13(4): (a) fresh and (b) 800 °C aged. Reaction conditions: 500 ppm NO, 500 ppm NH3, 10% O2, 5 vol% H2O, balance N2; GHSV = 80 000 h−1. | |
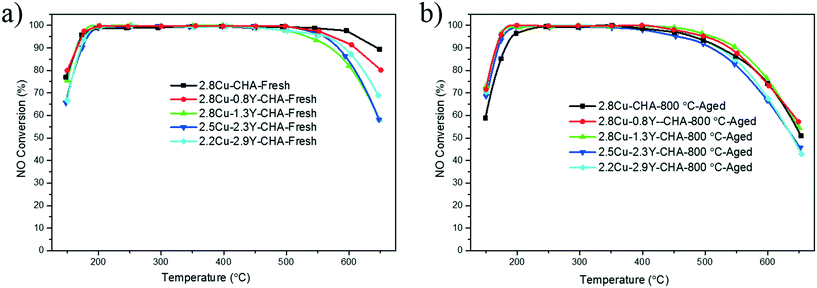 |
| Fig. 2 NO conversions as a function of temperature on (a) fresh and (b) hydrothermally aged Al-rich Cu–Y-SSZ-13(4) catalysts with different amounts of Y. Reaction conditions: 500 ppm NO, 500 ppm NH3, 10% O2, 5% H2O, balance N2; GHSV = 80 000 h−1. | |
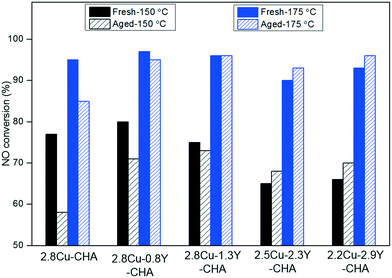 |
| Fig. 3 Low temperature (150 °C and 175 °C) NO conversions of fresh and hydrothermally aged Al-rich Cu–Y-SSZ-13 catalysts with different amounts of Y. Reaction conditions: 500 ppm NO, 500 ppm NH3, 10% O2, 5% H2O, balance N2; GHSV = 80 000 h−1. | |
3.2 Characterizations of fresh and aged Cu–Y-SSZ-13 catalysts
The XRD patterns of fresh and hydrothermally aged Al-rich Cu–Y-SSZ-13 catalysts shown in Fig. S5 (ESI†) show a typical CHA structure, and there is no significant change between the samples. This means that the long-range ordering of the CHA structure was maintained for all the catalysts after hydrothermal aging. The 27Al MAS NMR was conducted to probe the effect of Y on the coordination status of Al in Al-rich SSZ-13, with the spectra normalized to sample weight as shown in Fig. 4. The signals near 58, 37 and −2 ppm were assigned to the four-coordinated framework Al, and five- and six-coordinated extra-framework Al, respectively.13 To further exclude the effect of paramagnetic Cu2+ ions on the quantitative observation of the framework Al, the 27Al MAS NMR spectra of Na-SSZ-13 were compared with the Cu exchanged Na-SSZ-13. As shown in Fig. S6 (ESI†), both Na-SSZ-13 and Cu-SSZ-13 have no extra-framework Al. After exchange with Cu2+, there was nearly no broadening of the peak compared with Na-CHA because of the paramagnetic effect. The signal intensities were integrated and the areas were normalized with the sample weight. There was no more than 1% difference for the normalized total signal intensities. This means that the framework Al shown in Fig. 4 was not affected by the paramagnetic Cu2+. It is clearly shown in Fig. 4a and S7 (ESI†) that dealumination readily takes place during the calcination process for the preparation of fresh 2.8Cu-CHA, and the dealumination was also observed previously when Al-rich Na-CHA was transformed into H-CHA.13 The amount of framework Al increases as the Y content increases in the fresh catalysts. After hydrothermal treatment at 800 °C, all the catalysts showed a significant decrease of the signal intensities of framework Al at 57 ppm (Fig. 4b). Furthermore, the signal loss of framework Al became much more severe with the decrease of Y content, which means that the Y additive can stabilize the framework Al. The amount of framework Al can be directly determined using the 29Si MAS NMR spectra, as is shown in Fig. 5. The 29Si NMR peaks near −101, −107 and −113 ppm were assigned to Si(2Al), Si(1Al) and Si(0Al) groupings, respectively. After deconvolution and integration of the 29Si MAS NMR spectrum, the framework Si/Al ratios were obtained and are shown in Fig. 5. For the fresh catalyst, the signals of Si(2Al) and Si(1Al) increase with the Y content, which resulted in the framework Si/Al ratios in the Cu–Y-CHA decreasing from 6.9 to 5.2. This trend becomes more obvious for the aged catalysts and the framework Si/Al ratios decrease from 12.4 to 7.1. All these results indicated that the introduction of Y can stabilize the framework Al in Al-rich Cu-SSZ-13 zeolite.
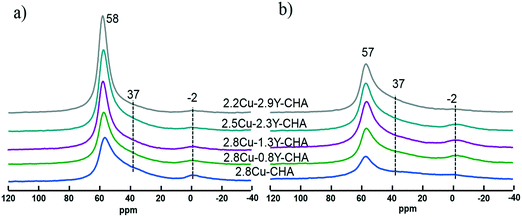 |
| Fig. 4
27Al MAS NMR spectra of Cu–Y-SSZ-13(4) catalysts: fresh (a) and hydrothermally aged (b). | |
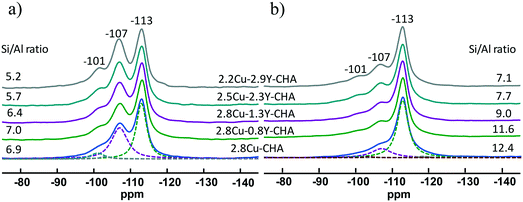 |
| Fig. 5
29Si MAS NMR spectra of Cu–Y-SSZ-13(4) catalysts: fresh (a) and hydrothermally aged (b). | |
1H MAS NMR was also carried out to study the Brønsted acidity of the Cu-SSZ-13 catalysts with various amounts of Y and the spectra normalized with the sample weight are shown in Fig. 6, and the quantified amounts of Brønsted sites are listed in Table S1 (ESI†). The signals at 4.0, 2.7, and 1.8 ppm were assigned to the Brønsted acid site, extra-framework AlOH and isolated silanol groups, respectively.32 All the fresh catalysts showed an appreciable amount of Brønsted acid sites, and the quantity of Brønsted acid sites varies from 0.41 to 0.64 mmol g−1 zeolite depending on Cu and Y loadings (Table S1, ESI†). After hydrothermal aging, most of the Brønsted acid sites disappear because of the dealumination of the SSZ-13 framework. However, the amount of residual Brønsted acid sites increased from 0.01 to 0.07 mmol g−1 zeolite as more Y was introduced [Fig. 6b and Table S1 (ESI†)] Thus, the Y additive can preserve the Brønsted acid sites in Cu-SSZ-13 because it can stabilize the framework Al which is directly associated with Brønsted acidity during hydrothermal aging.
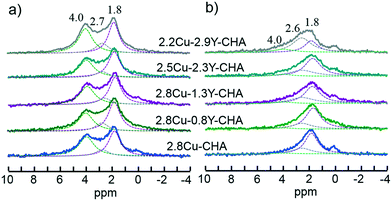 |
| Fig. 6
1H MAS NMR spectra of Cu–Y-SSZ-13(4) catalysts: fresh (a) and hydrothermally aged (b). | |
NH3-TPD profiles (Fig. 7a) of fresh Cu–Y-SSZ-13 catalysts typically show three desorption peaks near 200, 300 and 450 °C, respectively. The peak at 200 °C may be assigned to NH3 desorption at weak acid sites and/or physisorbed NH3.33 The peak near 300 °C may be ascribed to NH3 desorption at medium strength acid sites which may come from the Cu and/or Y ions [Fig. S8 (ESI†)], and the peak near 450 °C may be because of the NH3 desorption at strong acid sites which were probably the Brønsted acid sites. After hydrothermal aging, only one main broad peak near 230 °C and minor peak near 400 °C were observed (Fig. 7b). The disappearance of most strong acid sites, which were probably the Brønsted acid sites agrees well with the 1H MAS NMR results. However, aged Cu–Y-SSZ-13 catalysts showed the enhanced signal intensity of strong acid sites, which also indicated that addition of Y can maintain the Brønsted acid sites of Cu-SSZ-13. Furthermore, after hydrothermal aging, the amount of NH3 desorption at weak and medium strength acid sites is much smaller on Cu-SSZ-13 than on Cu–Y-SSZ-13.
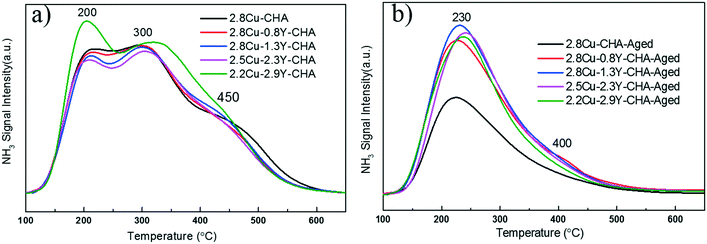 |
| Fig. 7 NH3-TPD profiles of Cu–Y-SSZ-13(4) catalysts: fresh (a) and hydrothermally aged (b). | |
Fig. 8 shows the UV-vis-NIR spectra of fresh and hydrothermally treated Cu–Y-SSZ-13 catalysts. All the spectra of the fresh catalysts show signals near 48
000 and 12
500 cm−1 which corresponded to the O2− → Cu2+ charge transfer, and the d–d transition of the isolated Cu2+ ions in an octahedral environment, respectively.34,35 After hydrothermal aging only 2.8Cu-CHA without the Y additive showed significant enhancement of the signal intensities at 40
000 cm−1 because of the formation of CuOx species (Fig. 8b).35 The H2-TPR experiments were conducted to further study the reducibility of Cu species in the fresh and aged Cu–Y-SSZ-13 catalysts, and the results are shown in Fig. 9. All the fresh catalysts exhibit a main broad reduction peak near 370 °C. This peak was assigned to the reduction of Cu2+ to Cu+ ions at 6-MR in the CHA zeolite.36,37 An overlapped peak near 245 °C may be attributed to the reduction peak of Cu2+ → Cu+ at 8-MR.36 The weak broad peaks near 200 °C may be because of the reduction of the very small amount of highly dispersed CuOx, which was formed during the high temperature ion exchange process.20,37 After hydrothermal aging, the reduction peak of Cu2+ → Cu+ at 6-MR shifts to around 340 °C for Cu–Y-CHA with Y loadings up to 1.3 wt%. However, with the increase of Y content, the main reduction peaks moved from 340 °C to 370 °C, and for aged 2.2Cu–2.9Y-CHA catalyst no change of reduction temperature of Cu2+ → Cu+ at 6-MR was observed. At the same time, the reduction peak of CuOx near 200 °C become inconspicuous for Cu–Y-CHA with Y content above 1.3 wt%.
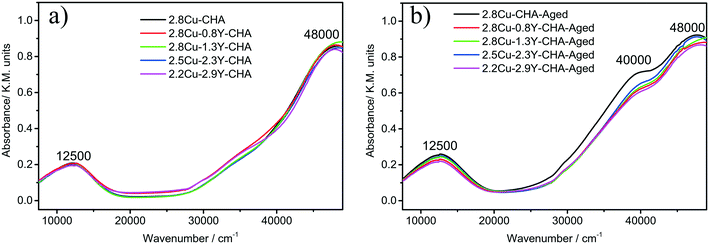 |
| Fig. 8 UV-vis-NIR spectra of Cu–Y-SSZ-13(4) catalysts: fresh (a) and hydrothermally aged (b). | |
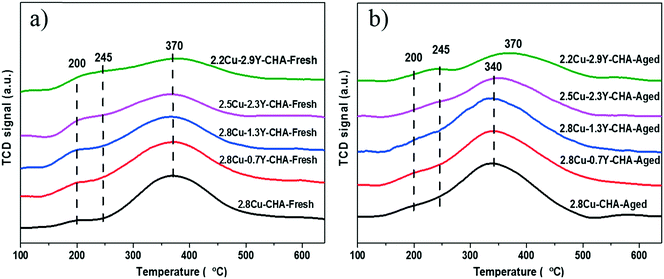 |
| Fig. 9 H2-TPR profiles of Cu–Y-SSZ-13(4) catalysts: fresh (a) and hydrothermally aged (b). | |
4. Discussion
Different RE ions all show different degrees of enhancement of low temperature activity after hydrothermal aging when compared to Cu-SSZ-13 without the addition of RE ions. This effect may partly result from the stabilization effect of RE ions on the zeolite. As has been previously reported, different RE ions including light group (La, Ce, Nd, Sm, Gd) and heavy group [dysprosium (Dy), erbium (Er), holmium (Ho), terbium (Tb), thulium (Tm)] all show enhancement of the thermal stability of the FAU zeolite.38 However, RE ions with different ion diameters and redox properties may be the major factors which influence the NH3-SCR activity. As there is rather limited space for diffusion of Cu ions during the ion-exchange process as well as the adsorption of reactants during the SCR reaction in CHA cage, RE ions with a larger diameter will lower the reaction activity. This is shown by the fact that Y with the smallest ion diameters show better catalytic performances. Whereas, for Ce ions, the formation of CeO2 shows oxidation properties and the NH3 oxidation reaction may proceed at high temperatures which leads to the significant loss of high temperature NO conversions after hydrothermal aging (Fig. 1b). In light of practical applications, Y could be a promising additive because of its higher natural abundance and the higher SCR performance of Cu–Y-SSZ-13. It should be noted that trivalent RE ions may exist as RE3+, RE(OH)2+, RE(OH)2+ and multinuclear OH-bridged cationic aggregate species such as rare-earth La ions in the FAU zeolite proposed by Schüßler et al.23 However, the signal of the Y–OH groups was not observed or cannot be distinguished using the 1H MAS NMR spectra in Fig. 6 for both fresh and aged samples. Nevertheless, Y species should be incorporated into SSZ-13 because from the SEM-EDX analysis of the Y distribution on the zeolite surface [Fig. S9 (ESI†)], it is clear that the Y content in the selected areas was almost the same as 1.3 wt%, and very close to the ICP-AES result for 2.8Cu–1.3Y-CHA. However, the Y contents in 2.2Cu–2.9Y-CHA were also very homogeneous ranging from 3.4 to 3.9 wt%, which was slightly higher than the ICP result of 2.9 wt%. The surface enrichment of the Y species was partly because more Y ions were exchanged near the surface because of the shorter diffusion distance during the exchange process if the analysis errors were excluded. To further confirm that Y ions could be successfully introduced into the ion-exchange sites of the SSZ-13 zeolite, two-dimensional 23Na MQ MAS NMR was conducted using Na+ as the probe because as shown in a previous paper by Zhao et al., the extra-framework Na+ ions at the exchange sites were very sensitive to their location in the SSZ-13 zeolite.39 The 23Na MQ MAS NMR spectra of Na-CHA and Na,Y-CHA are shown in Fig. 10. In the Na-CHA zeolite (Fig. 10a), the signals with chemical shifts at isotropic dimension (F1) at approximately 3 and −13 ppm corresponded to Na+ ions at the 6-MR and 8-MR windows, respectively.39,40 After introduction of the Y ions, the signals of Na+ at 8-MR decreased significantly (Fig. 10b). This clearly demonstrated that Y ions can be exchanged with Na+ to enter the ion-exchange sites of the CHA zeolite.
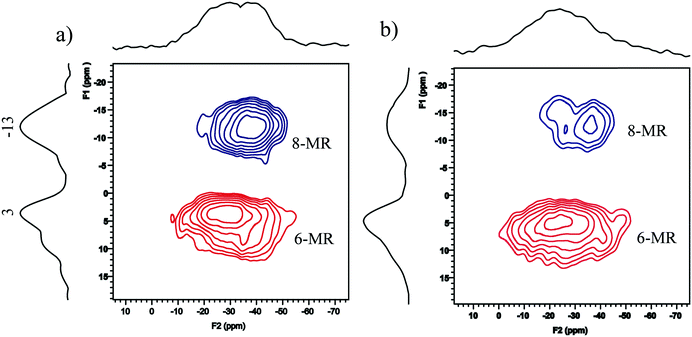 |
| Fig. 10
23Na MQ MAS NMR spectra of parent Na-SSZ-13 (a) and Y3+ exchanged Na-SSZ-13 (1.3 wt% Y) (b). | |
The hydrothermal stability of Cu-SSZ-13 is mainly dependent on the stability of the framework Al in the zeolite and Cu ions at the ion-exchange sites. For Al-rich SSZ-13 zeolite, dealumination readily happened even in fresh Cu-SSZ-13 catalyst, and the dealumination mainly occurred at Al sites possessing Brønsted acidity. With more Brønsted acid sites substituted by Y ions more framework Al will be preserved in the Cu-SSZ-13 zeolite. The stabilization effect of RE ions on zeolite for Al-rich Cu-SSZ-13 may be similar to that of Na+ previously reported by Zhao et al. and Gao et al.13,17 The significant difference was that too much amount of Na+ was detrimental to the hydrothermal stability of the Cu-SSZ-13 zeolite,13,17,19 whereas in this case more Y can still enhance the hydrothermal stability of Cu-SSZ-13. The detrimental effect of Na+ in the Cu-SSZ-13 zeolite was ascribed to the weakening of the interaction between Cu2+ and zeolite, which resulted in sintering of the Cu active center. For the fresh Y exchanged Cu-SSZ-13 catalysts, the H2-TPR profiles of Cu–Y-SSZ-13 indicated that the reduction peak of Cu2+ → Cu+ tended to be unchanged with different Y loadings, and after hydrothermal aging the reduction peak even shifted to a higher temperature with the increase of the Y loadings (Fig. 9). This means that the introduction of Y can stabilize Cu2+ ions and even maintain the Cu2+ ion in its fresh state for Cu–Y-CHA with the highest Y loading of 2.9 wt% after aging at 800 °C. The strong polarization effect of the trivalent RE ions altered the Si–O–Al, Si–O–Si angles as well as the local charge density of the zeolite framework, which may play an important role in stabilizing the Cu2+ ions.41
To probe the status of the Cu ions in the Cu–Y-SSZ-13 zeolite, DRIFT spectra of NO adsorption were acquired for the Cu–Y-SSZ-13 zeolite and are shown in Fig. 11. The peaks at 1947 cm−1 were assigned to NO adsorbed on the Cu2+ ion at 6-MR, and the signals near 1910 and 1890 cm−1 were assigned to NO adsorbed on Cu(OH)+ in the CHA cage.42 No peak near 1810 cm−1 of NO adsorbed on Cu+ was observed (not shown). The IR spectra of NO adsorption in the first 1–3 min indicated that most of the Cu2+ ions were located at 6-MR for all the fresh Cu–Y-CHA zeolites, which was in agreement with the H2-TPR results.43 With prolonged NO exposure time, the peak intensities of NO adsorbed at Cu(OH)+ in the CHA cage increased significantly, which was similar to results in a previous paper by Szanyi et al.44 However, the significant difference among the Cu–Y-CHA zeolites was that the relative signal intensity near 1947 cm−1 becomes much stronger with prolonged NO exposure time for the Cu–Y-CHA with a higher Y content. These results mean that with more Y introduced into the Cu-CHA zeolites, Cu2+ ions were much more inclined to stay at 6-MR, and therefore, it is relatively more stable. After hydrothermal aging, for Cu-SSZ-13 without Y, the relative intensity of NO adsorbed on Cu2+ ion at 6-MR obviously decreases. However, its relative intensity increases with increase of the Y content. Another peak near 1930 cm−1 becomes less pronounced with increase of Y content, which was assigned to the NO adsorbed at Cu2+ located at energetically less favorable 6-MR sites.42 Additionally, the DRIFT spectra (Fig. S10, ESI†) of adsorbed NH3 subtracted from the background spectra acquired before the NH3 adsorption indicated that the relative amount of Cu2+ ions at 6-MR (perturbed T–O–T vibration at ∼900 cm−1)45 increased with Y loadings for both fresh and aged catalysts, which also means that the introduction of Y caused the occupation of 6-MR by the Cu2+ ions. All these results indicated that addition of Y may stabilize Cu2+ ions at 6-MR. It was recognized that the excellent hydrothermal stability of the Cu-SSZ-13 zeolite was attributed to the occupation of the 6-MR 2Al site by Cu2+ ions.16,20 During hydrothermal treatment, the Cu2+ at 6-MR may migrate to the CHA cage to form hydrated ions in the presence of steam,36 and furthermore form unstable Cu(OH)+ species because of the dealumination at high temperatures, and this species will finally transform into CuOx or react with dealuminated Al(OH)3 species.21 Introduction of Y can enhance the stability of Cu2+ at 6-MR as well as at the Al framework, which may prohibit this process. A very recent paper shows that a small amount of Ce can significantly enhance the hydrothermal stability of Al-rich SSZ-13 with a higher Cu loading of 3.6 wt%.28 In this research, similarly, even 0.8 wt% of Y additive can inhibit the loss of NO conversion at a low temperature after hydrothermal aging, but 27Al and 29Si MAS NMR results clearly indicate that if there are not enough Y ions the dealumination of the zeolite cannot be effectively prohibited.
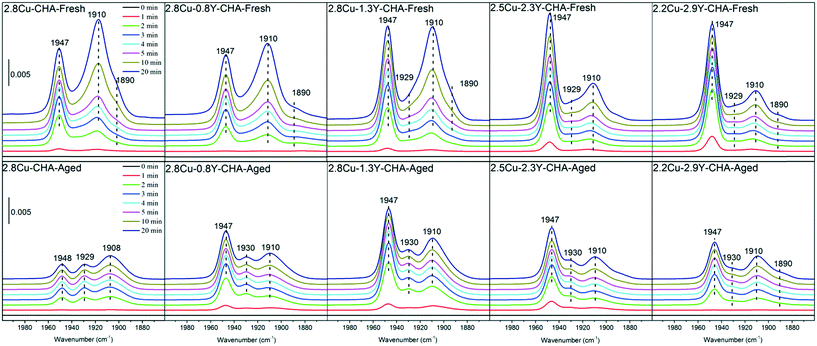 |
| Fig. 11 DRIFT spectra of fresh and aged Cu–Y-SSZ-13 zeolites acquired at 298 K with prolonged NO exposure time from 0 to 20 min. | |
It is worth noting that the amount of Y additive should be moderate. For hydrothermal stability, more Y will be beneficial, whereas it will lower both low and high temperature NO conversions in the fresh catalyst. One direct reason for this is that introduction of Y ions will hinder the exchange of Cu2+. As Y ions have large hydrated ion diameters, once Y is introduced into CHA cages at a high temperature, diffusion of Cu ions from solution to the zeolite matrix would become difficult during the ion-exchange process. Therefore, less Cu was loaded for Cu–Y-SSZ-13 with a Y content above 2.3 wt%. The decrease of low temperature activity of fresh Cu–Y-SSZ-13 was also significantly different from that with a Na+ additive, which can enhance the low temperature activity.13,17 Recent advances on low temperature NH3-SCR reaction mechanism indicated that the formation of the Cu2+ dimer determined the low temperature activity. The Na+ additive can weaken the interaction between Cu2+ and the zeolite framework, which favored the migration of Cu ions to form dimers. Whereas, the normalized reaction rates of fresh Cu–Y-CHA series catalysts shown in Table S2 (ESI†) are very close, which means the intrinsic activities of Cu2+ ions are the same for all the Cu-CHA and Cu–Y-CHA catalysts.
When compared with the conventional organotemplated high silica 2.5 wt% Cu-SSZ-13 (Si/Al = 15), 2.8Cu–1.3Y-SSZ-13 with a similar amount of Cu and optimized Y content shows similar NO conversions from the low to middle temperature range but much higher NO conversions above 500 °C (Fig. 12). After hydrothermal aging, they show almost the same NH3-SCR activity in a wide temperature window range from 150 °C to 650 °C except that the 2.8Cu–1.3Y-CHA shows slightly higher low temperature NO conversions, and this comparable activity was reproducible at even higher GHSV of 400
000 h−1 (Fig. S11, ESI†). These results demonstrate that RE ions are an effective additive to enhance the hydrothermal stability of Al-rich SSZ-13 synthesized using an organotemplate-free approach. The RE ions bring with them promising applications for the NH3-SCR reaction because of the low cost of zeolite production, much less energy consumption and less pollution for the environment because of the removal of the use of organic templates and high temperature calcination.
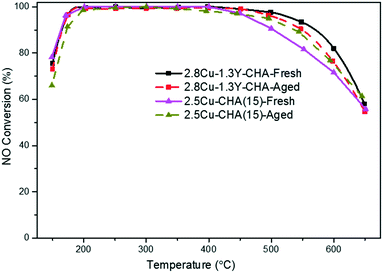 |
| Fig. 12 NO conversions as a function of temperature for fresh and hydrothermally aged Al-rich 2.8Cu–1.3Y-SSZ-13 and conventional organotemplated high silica 2.5Cu-SSZ-13(15) catalysts. Reaction conditions: 500 ppm NO, 500 ppm NH3, 10% O2, 5% H2O, balance N2; GHSV = 80 000 h−1. | |
5. Conclusions
Rare-earth ion (Ce, La, Sm, Y, Yb) stabilized Al-rich Cu-SSZ-13 zeolites from organotemplate-free synthesis have been prepared and tested in the NH3-SCR reaction. All the RE ion modified Al-rich Cu-SSZ-13 catalysts show enhancement of low temperature NO conversions after hydrothermal aging at 800 °C when compared with Cu-SSZ-13. Among the tested RE ions, addition of Y shows significant enhancement of hydrothermal stability and NH3-SCR activities after hydrothermal aging of Cu–Y-SSZ-13. The Y content in Cu–Y-SSZ-13 was optimized in the NH3-SCR using fresh and aged catalysts. The SEM-EDX analysis and 23Na MQ MAS NMR confirm that Y ions are successfully introduced into the ion-exchange sites of the SSZ-13 zeolite. The 27Al and 29Si MAS NMR demonstrate that more of the framework Al in SSZ-13 zeolite can be preserved with the increase of Y content in both fresh and hydrothermally aged catalysts. The NH3-TPD and quantitative 1H MAS NMR results illustrate that addition of Y can maintain the Brønsted acid sites of Cu–Y-SSZ-13. The UV-vis-NIR, H2-TPR results combined with the DRIFT spectra of the NO/NH3 adsorption demonstrate that the Y additive enhances the stability of Cu2+ ions at 6-MR. However, an excess of Y would hinder the effective introduction of Cu ions and decrease the low temperature NO conversions. The optimized Al-rich 2.8Cu–1.3Y-SSZ-13 catalyst shows comparable NH3-SCR performances when compared with the conventional organotemplated high silica 2.5Cu-SSZ-13 catalyst after 800 °C hydrothermal treatment, and could be a promising candidate for further practical applications.
Conflicts of interest
There are no conflicts to declare.
Acknowledgements
This work was financially supported by the National Natural Science Foundation of China (No. 2187020455, 21603022), the Fundamental Research Funds for the Central Universities in China (No. DUT16RC(3)002, DUT17TD04), Open Project Funds of the State Key Laboratory of Catalysis, Dalian Institute of Chemical Physics (No. N-16-08), the Program for Liaoning Innovative Research Team in University (No. LT2016001), and the INCOE (International Network of Centers of Excellence) project coordinated by BASF SE, Germany.
References
- R. Zhang, N. Liu, Z. Lei and B. Chen, Chem. Rev., 2016, 116, 3658–3721 CrossRef CAS PubMed.
- D. W. Fickel, E. D'Addio, J. A. Lauterbach and R. F. Lobo, Appl. Catal., B, 2011, 102, 441–448 CrossRef CAS.
- A. M. Beale, F. Gao, I. Lezcano-Gonzalez, C. H. F. Peden and J. Szanyi, Chem. Soc. Rev., 2015, 44, 7371–7405 RSC.
-
I. Bull, R. S. Boorse, W. M. Jaglowski, G. S. Koermer, A. Moini, J. A. Patchett, W. Xue, P. Burk, J. C. Dettling and M. T. Caudle, US2008226545A1, 2008.
- J. Li, H. Chang, L. Ma, J. Hao and R. T. Yang, Catal. Today, 2011, 175, 147–156 CrossRef CAS.
- W. Shan and H. Song, Catal. Sci. Technol., 2015, 5, 4280–4288 RSC.
- R. Oord, I. C. ten Have, J. M. Arends, F. C. Hendriks, J. Schmidt, I. Lezcano-Gonzalez and B. M. Weckhuysen, Catal. Sci. Technol., 2017, 7, 3851–3862 RSC.
- C. Paolucci, A. A. Parekh, I. Khurana, J. R. Di Iorio, H. Li, J. D. Albarracin Caballero, A. J. Shih, T. Anggara, W. N. Delgass, J. T. Miller, F. H. Ribeiro, R. Gounder and W. F. Schneider, J. Am. Chem. Soc., 2016, 138, 6028–6048 CrossRef CAS PubMed.
- F. Gao, D. Mei, Y. Wang, J. Szanyi and C. H. F. Peden, J. Am. Chem. Soc., 2017, 139, 4935–4942 CrossRef CAS PubMed.
- C. Paolucci, I. Khurana, A. A. Parekh, S. Li, A. J. Shih, H. Li, J. R. Di Iorio, J. D. Albarracin-Caballero, A. Yezerets, J. T. Miller, W. N. Delgass, F. H. Ribeiro, W. F. Schneider and R. Gounder, Science, 2017, 357, 898–903 CrossRef CAS PubMed.
- S. J. Schmieg, S. H. Oh, C. H. Kim, D. B. Brown, J. H. Lee, C. H. F. Peden and D. H. Kim, Catal. Today, 2012, 184, 252–261 CrossRef CAS.
- F. Gao, N. M. Washton, Y. Wang, M. Kollár, J. Szanyi and C. H. F. Peden, J. Catal., 2015, 331, 25–38 CrossRef CAS.
- Z. Zhao, R. Yu, R. Zhao, C. Shi, H. Gies, F.-S. Xiao, D. De Vos, T. Yokoi, X. Bao, U. Kolb, M. Feyen, R. McGuire, S. Maurer, A. Moini, U. Müller and W. Zhang, Appl. Catal., B, 2017, 217, 421–428 CrossRef CAS.
- A. Marberger, A. W. Petrov, P. Steiger, M. Elsener, O. Krøcher, M. Nachtegaal and D. Ferri, Nat. Catal., 2018, 1, 221–227 CrossRef.
- L. Xu, C. Shi, Z. S. Zhang, H. Gies, F. S. Xiao, D. De Vos, T. Yokoi, X. H. Bao, M. Feyen, S. Maurer, B. Yilmaz, U. Muller and W. P. Zhang, Microporous Mesoporo'us Mater., 2014, 200, 304–310 CrossRef CAS.
- J. Song, Y. Wang, E. D. Walter, N. M. Washton, D. Mei, L. Kovarik, M. H. Engelhard, S. Prodinger, Y. Wang, C. H. F. Peden and F. Gao, ACS Catal., 2017, 7, 8214–8227 CrossRef CAS.
- F. Gao, Y. L. Wang, N. M. Washton, M. Kollar, J. Szanyi and C. H. F. Peden, ACS Catal., 2015, 5, 6780–6791 CrossRef CAS.
- L. M. Ren, L. F. Zhu, C. G. Yang, Y. M. Chen, Q. Sun, H. Y. Zhang, C. J. Li, F. Nawaz, X. J. Meng and F. S. Xiao, Chem. Commun., 2011, 47, 9789–9791 RSC.
- L. Xie, F. Liu, X. Shi, F.-S. Xiao and H. He, Appl. Catal., B, 2015, 179, 206–212 CrossRef CAS.
- Y. J. Kim, J. K. Lee, K. M. Min, S. B. Hong, I. S. Nam and B. K. Cho, J. Catal., 2014, 311, 447–457 CrossRef CAS.
- S. Han, Q. Ye, S. Cheng, T. Kang and H. Dai, Catal. Sci. Technol., 2017, 7, 703–717 RSC.
- E. F. Sousa-Aguiar, F. E. Trigueiro and F. M. Z. Zotin, Catal. Today, 2013, 218-219, 115–122 CrossRef CAS.
- F. Schüßler, E. A. Pidko, R. Kolvenbach, C. Sievers, E. J. M. Hensen, R. A. van Santen and J. A. Lercher, J. Phys. Chem. C, 2011, 115, 21763–21776 CrossRef.
- M. Iwasaki and H. Shinjoh, Chem. Commun., 2011, 47, 3966–3968 RSC.
- Y. Shu, A. Travert, R. Schiller, M. Ziebarth, R. Wormsbecher and W.-C. Cheng, Top. Catal., 2015, 58, 334–342 CrossRef CAS.
- Y. Q. Jia, J. Solid State Chem., 1991, 95, 184–187 CrossRef CAS.
- J. Wang, Z. Peng, H. Qiao, H. Yu, Y. Hu, L. Chang and W. Bao, Ind. Eng. Chem. Res., 2016, 55, 1174–1182 CrossRef CAS.
- T. Usui, Z. Liu, S. Ibe, J. Zhu, C. Anand, H. Igarashi, N. Onaya, Y. Sasaki, Y. Shiramata, T. Kusamoto and T. Wakihara, ACS Catal., 2018, 8, 9165–9173 CrossRef CAS.
-
R. Xu, W. Pang, J. Yu, Q. Huo and J. Chen, in Chemistry of Zeolites and Related Porous Materials, John Wiley & Sons, Ltd, 2010, pp. 345–395 Search PubMed.
- M. Müller, G. Harvey and R. Prins, Microporous Mesoporous Mater., 2000, 34, 281–290 CrossRef.
- X. Li, W. Zhang, S. Liu, L. Xu, X. Han and X. Bao, J. Catal., 2007, 250, 55–66 CrossRef CAS.
- W. Zhang, S. Xu, X. Han and X. Bao, Chem. Soc. Rev., 2012, 41, 192–210 RSC.
- F. Giordanino, E. Borfecchia, K. A. Lomachenko, A. Lazzarini, G. Agostini, E. Gallo, A. V. Soldatov, P. Beato, S. Bordiga and C. Lamberti, J. Phys. Chem. Lett., 2014, 5, 1552–1559 CrossRef CAS PubMed.
- J. Dědeček and B. Wichterlová, J. Phys. Chem. B, 1997, 101, 10233–10240 CrossRef.
- I. Lezcano-Gonzalez, U. Deka, H. E. van der Bij, P. Paalanen, B. Arstad, B. M. Weckhuysen and A. M. Beale, Appl. Catal., B, 2014, 154, 339–349 CrossRef.
- J. H. Kwak, H. Zhu, J. H. Lee, C. H. F. Peden and J. Szanyi, Chem. Commun., 2012, 48, 4758–4760 RSC.
- L. Ma, Y. S. Cheng, G. Cavataio, R. W. McCabe, L. X. Fu and J. H. Li, Chem. Eng. J., 2013, 225, 323–330 CrossRef CAS.
- F. E. Trigueiro, D. F. J. Monteiro, F. M. Z. Zotin and E. Falabella Sousa-Aguiar, J. Alloys Compd., 2002, 344, 337–341 CrossRef CAS.
- Z. Zhao, Y. Xing, S. Li, X. Meng, F.-s. Xiao, R. McGuire, A.-N. Parvulescu, U. Müller and W. Zhang, J. Phys. Chem. C, 2018, 122, 9973–9979 CrossRef CAS.
- L. J. Smith, H. Eckert and A. K. Cheetham, J. Am. Chem. Soc., 2000, 122, 1700–1708 CrossRef CAS.
- J. A. van Bokhoven, A. L. Roest, D. C. Koningsberger, J. T. Miller, G. H. Nachtegaal and A. P. M. Kentgens, J. Phys. Chem. B, 2000, 104, 6743–6754 CrossRef CAS.
- R. Zhang, J.-S. McEwen, M. Kollár, F. Gao, Y. Wang, J. Szanyi and C. H. F. Peden, ACS Catal., 2014, 4, 4093–4105 CrossRef CAS.
- F. Goltl, P. Sautet and I. Hermans, Angew. Chem., Int. Ed., 2015, 54, 7799–7804 CrossRef PubMed.
- J. Szanyi, J. H. Kwak, H. Zhu and C. H. F. Peden, Phys. Chem. Chem. Phys., 2013, 15, 2368–2380 RSC.
- J. Luo, D. Wang, A. Kumar, J. Li, K. Kamasamudram, N. Currier and A. Yezerets, Catal. Today, 2016, 267, 3–9 CrossRef CAS.
Footnotes |
† Electronic supplementary information (ESI) available: The N2O yields of fresh and aged Cu–RE-SSZ-13 catalysts. XRD patterns of fresh and aged Al-rich Cu–Y-SSZ-13(4) catalysts. The 27Al- and 29Si-MAS-NMR spectra of Na-SSZ-13(4), H-SSZ-13(4), Cu–Na-SSZ-13(4) and Cu,Y-SSZ-13(4). The NH3-TPD profiles of 3.2Y–H-SSZ-13(4). Quantitative results of Brønsted acid sites in fresh and aged Cu–Y-CHA(4) catalysts derived from the 1H-MAS-NMR measurements. The SEM-EDX results of representative Cu–Y-SSZ-13(4) catalysts. The DRIFT spectra of NH3 adsorbed Cu–Y-SSZ-13(4) catalysts. The NO conversions as a function of temperature for Y-CHA(4), hydrothermally aged 2.8Cu–1.3Y-CHA(4) and organotemplated high silica 2.5Cu-SSZ-13(15) catalysts at GHSV of 80 000 and 400 000 h−1. The NO conversions as a function of temperature for fresh and hydrothermally aged Al-rich Cu–Y-SSZ-13(4) at GHSV of 400 000 h−1, and their normalized reaction rates. See DOI: 10.1039/c8cy02033g |
‡ RY and ZZ contributed equally to this work. |
|
This journal is © The Royal Society of Chemistry 2019 |
Click here to see how this site uses Cookies. View our privacy policy here.