DOI:
10.1039/C8BM01348A
(Review Article)
Biomater. Sci., 2019,
7, 3566-3580
Native cardiac environment and its impact on engineering cardiac tissue
Received
23rd October 2018
, Accepted 16th April 2019
First published on 24th July 2019
Abstract
Human pluripotent stem cell-derived cardiomyocytes (hPSC-CMs) generally have an immature fetal-like phenotype when directly compared to isolated CMs from human hearts, despite significant advance in differentiation of human pluripotent stem cells (hPSCs) to multiple cardiac lineages. Therefore, hPSC-CMs may not accurately mimic all facets of healthy and diseased human adult CMs. During embryonic development, the cardiac extracellular matrix (ECM) experiences a gradual assembly of matrix proteins that transits along the maturation of CMs. Mimicking these dynamic stages may contribute to hPSC-CMs maturation in vitro. Thus, in this review, we describe the progressive build-up of the cardiac ECM during embryonic development, the ECM of the adult human heart and the application of natural and synthetic biomaterials for cardiac tissue engineering with hPSC-CMs.
Introduction
Since the first isolation of human pluripotent stem cells (hPSCs), their remarkable capacity to self-replicate and differentiate into every cell type of the human body aroused great interest and has advanced the fields of human disease modeling and pre-clinical pharmacology. Particularly because of the limited regenerative capacity of the human heart, as well as the difficulty to propagate primary cardiomyocytes (CMs) in vitro, hPSC-derived CMs (hPSC-CMs) are increasingly acknowledged as an alternative and accessible cell source for disease modeling, as well as drug and safety pharmacology or regenerative cardiac repair.1,2,3–10,11–15 Protocols to generate distinct cellular subtypes of the human heart, which include ventricular,16,17 atrial18–21 and pacemaker CMs,22,23 as well as epicardial cells and epicardial-derived smooth muscle cells and fibroblasts24–26 and endothelial cells27,28 have been developed and are continuously optimized. Despite these significant advances in directing differentiation to multiple cardiac lineages, hPSC-CMs are generally characterized by an immature fetal-like phenotype and may therefore not accurately mimic all facets of healthy and diseased human adult CMs. In a direct comparison to human hearts, gene expression patterns and morphological and functional characteristics of hPSC-CMs resemble that of fetal hearts (Fig. 1).2,29–31 For a detailed overview about the immaturity of hPSC-CMs, we refer to other comprehensive reviews on this topic.32–35
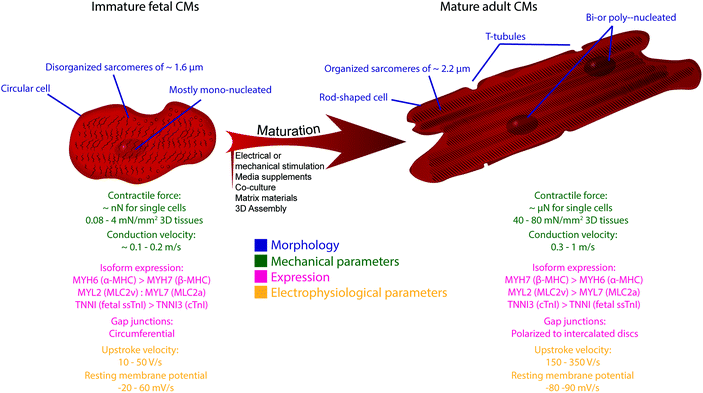 |
| Fig. 1 Strategies to enhance the maturity level of hPSC-CMs. | |
Various strategies have been applied to enhance maturity of hPSC-CMs in vitro, including prolonged time in culture, electrical or mechanical stimulation, addition of chemical or biological factors, co-culture with non-CMs, three-dimensional (3D) assembly into tissues and the use of specific scaffold materials (Fig. 1).32,33,36 Despite distinct improvements – those strategies often only induce some aspects attributed to cardiac maturity – hPSC-CMs never acquired an adult cardiac phenotype. Therefore, further progress necessitates the development of more advanced models, as for example engineering 3D cardiac tissue surrogates closely resembling the native cardiac microenvironment.
In this review, we describe the expression of extracellular matrix (ECM) proteins in the native microenvironment of human CMs with focus on developmental changes and respect to different heart compartments, such as atria and ventricles and also the right and left side of the heart. Moreover, we discuss the application of natural and synthetic biomaterials in the context of in vitro cardiac tissue engineering with hPSC-CMs and their effect on hPSC-CM maturity.
The native environment of CMs
Cells in their native environment are embedded within a network of ECM components, which are crucial for proper development and function of tissues and organs. The ECM delivers vital cues for various cellular responses, which include fate determination, migration, proliferation, morphology, cell arrangement, survival and maturation. The cardiac ECM additionally needs to provide a strong support and elastic anchorage for precisely aligned CMs and creates a specialized environment to permit electrical coupling and cardiac impulse propagation between adjacent cells and transmission of contractile forces of CMs to the surrounding matrix for the repetitive blood pumping function of the heart.37–41
The basic structural unit of the cardiac ECM is primarily composed of collagen, but also includes laminin, fibronectin, fibrillin and elastin, which are continuously secreted by different cell types and assembled into a hierarchically organized scaffold (Table 1).42
Table 1 Proteins of the ECM in the heart. Expression, structure and function of most common ECM proteins
|
Expression |
Structure |
Function |
Secreted by: |
|
Collagen I |
Extra- and intracellularly in all tissues, but not in basement membrane |
Triple-helical fibrils from fibrillary collagen |
Structural support and strength |
Fibroblasts |
|
Collagen III |
Extra- and intracellularly in all tissues, but not in basement membrane |
Triple-helical fibrils from fibrillary collagen |
Structural support and elasticity |
Fibroblasts and smooth muscle cells |
|
Collagen IV |
Predominant protein in basement membrane |
Rope-like, very long fibrils and sheet-like network |
Cell–ECM interaction, connection to other ECM proteins |
Fibroblasts, smooth muscle cells and CMs |
|
Collagen VI |
Extracellularly in all tissues, but not in basement membrane or intracellularly |
Fine filaments in 90° orientation to other collagens |
Adhesion and migration of cells, connection to other ECM proteins |
Fibroblasts and smooth muscle cells |
|
Fibronectin |
Extra- and intracellularly in all tissues and plasma |
Two chains connected by disulfide bridges |
Cell–ECM interaction via cell surface receptors, such as integrins |
Fibroblasts and smooth muscle cells |
|
Dimers connect into fibrils only via integrin receptors on cell surfaces |
|
Laminin |
Most prominent glycoprotein in basement membrane |
Self-assemble into asymmetric planar sheets |
Cell–ECM interaction via cell surface receptors, such as integrins |
Fibroblasts, smooth muscle cells and CMs |
|
Elastin and fibrillin |
Extracellularly |
Long, very hydrophobic protein |
Elasticity |
Fibroblasts and smooth muscle cells |
|
No stretch: Circular compact Stretch: elongated interconnected strains |
Fibrillary collagens type I and type III form a complex fiber scaffold composed of thicker collagen I and thinner collagen III triple helical fibrils that have interconnected into larger fibers (fibril diameter depends on the tissue: in adult healthy cardiac tissue collagen I fibril diameter varies around 75 nm and collagen III fibrils around 45 nm
43). Thicker collagen I fibers are important to convey the necessary rigidity and stability, while collagen III fibers support the elasticity of the cardiac ECM.41 Additional elastic flexibility of the cardiac wall is regulated by distribution and content of interspersed elastic fibers. Elastic fibers are primarily made from microfibrillary fibrillin with amorphous elastin as core protein.44 Together with collagen type IV, laminin has a key role in linking CMs to their surrounding ECM and serves as cell adhesive protein.45–47 Similarly, fibronectin anchors cells to the ECM and transduces extracellular signaling to mediate cellular response.48 In mice, knockout of fibronectin has been shown to result in differentiation of cardiac progenitors towards CMs while inhibiting proliferation of cardiac progenitor cells in the pre-cardiac mesoderm during cardiac morphogenesis.49 Another key component of the ECM are glycosaminoglycans, which interact with several signaling molecules to regulate differentiation, protection against oxidative stress and also cardiac remodeling after injury.50 In human left and right atrial and ventricular walls, five different glycosaminoglycans have been identified: hyaluronic acid, heparan sulfate, dermatan sulfate, chondroitin-4-sulfate and chondroitin-6-sulfate.51,52
Most ECM proteins interact with cells via transmembrane receptors, so-called integrins, which do not only function as principal anchor for the cells to their surrounding ECM, but also mediate bi-directional communication across the membrane to regulate cellular behavior via ligand binding on the outside (outside-in), or receptor activity by intracellular signaling (inside-out signaling). In the heart, integrins are also linked to the cytoskeletal protein alpha-actinin via talin and vinculin, thereby transmitting the mechanical forces from the inside of CMs to the ECM.53,54
Native cardiac ECM during human heart development
Composition of the ECM is dynamic throughout embryonic development and adulthood, thereby providing stage-dependent clues for maturation of CMs (Fig. 2).
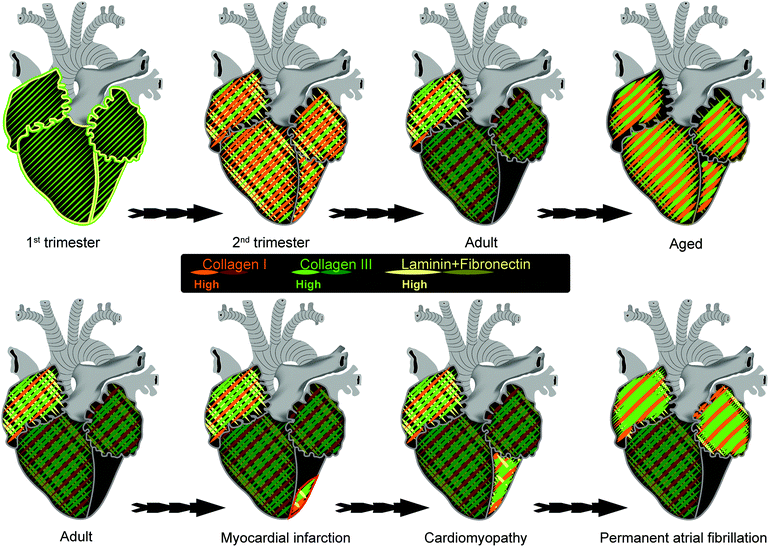 |
| Fig. 2 Upper panel: Schematic representation of the dynamic changes of the expression of ECM proteins in the heart. During the first trimester, the ECM network is primarily composed of collagen III. Laminin and fibronectin localize to endo- and epicardial layers. In the second trimester, collagen I, as well as laminin and fibronectin become more widely expressed and collagen fiber thickness increases. The premature collagen network develops into a highly organized network. In postnatal and adult hearts, laminin strands have interconnected to form a complex fibrillary network. Collagen III is now twice more abundant than collagen type I. Additionally, collagen I and III are twice more abundant in the myocardium of both atria than ventricles and collagen I and III amounts are higher in the right heart compared to the left. With increasing age the ratio of thick, densely packed collagen, as well as the fiber thickness increase. Lower panel: Schematic representation of the ECM changes in the diseased heart. Post myocardial infarction around the infarct zone (marked in red in left ventricle) or in fibrotic scar tissue in cardiomyopathy (see left ventricle), enhanced deposition of collagen type I, III, IV and VI, as well as fibronectin and laminin has been observed. Similarly, permanent atrial fibrillation leads to an increase in collagen I, III and IV in both atria. | |
Early during heart development, around the end of the first trimester of human development, laminin, fibronectin and most collagen fibers predominantly localize along the endocardial and epicardial layers45,55 CMs within the myocardium are supported by a premature collagen network primarily build from thin, loose fibers of collagen type III only.55
At the beginning of the second trimester with the onset of increased fetal growth and higher energy demands in the embryo, fibronectin and notably laminin become more widely distributed and strongly expressed within the myocardium.45,47 Laminin is particularly expressed in the basal membrane of CMs, but also smooth muscle and capillary endothelial cells.46 The basal membrane is a dense, highly organized layer that connects each cell with their surrounding ECM. Laminins are heteromerically formed by cell subtype-specific structural subchains, e.g. the A1-chain is primarily present in the basal membrane of vascular cells and not CMs, whereas the A2-chain is only present around CMs.45,47,56,57 Fibronectin is predominantly localized around blood vessels close to smooth muscle cells and the basal membrane of fibroblasts and along endo- and epicardial layers.45,47,56,57 Along with progressive build-up of the ECM, collagen fiber thickness increases and the premature collagen network quickly develops into a highly aligned, interconnected and intercoiled network with similar ratios of collagen type I and III, or at the most a small shift in favor of collagen I.41,46,55
Postnatally, laminin strands have interconnected and built a fine, complex fibrillary network throughout the myocardium.45,47,56,57 Despite no differences in total laminin, cardiac A2-laminin, fibronectin and total collagen proportions decrease immediately after birth, leading to a reduction of approximately 50% in the adult heart.41,45,56
Less attention has been paid to the expression and distribution of less prominent collagens, such as collagen type IV and VI, as well as fibrillin and elastin in human myocardium during development. Moreover, little is known about expression of fibronectin isoforms in human fetal or adult cardiac tissues. However, in the mouse heart, three different isoforms of highly conserved fibronectin are generated by alternative splicing. Domains A and B, but not C of fibronectin are specifically expressed during embryonic development of the heart, but not in adult stages.58
Native ECM in adult heart
In adulthood, collagen type III is twice more abundant than collagen type I.41,46 It is important to note that collagen is not evenly distributed throughout the adult heart, most of the collagen resides in the endo- and epicardium with a dense, thick subendocardial network, which gradually changes to a thinner subepicardial network. Similarly a dense, thick network of collagen fibrils can be observed at the base of the heart, close to the atrioventricular valves below the papillary muscles, which gradually declines in thickness in the direction of the apex of the heart.55 In conjunction with lower atrial pressures and less compliant atrial compared to ventricular muscle, collagen I and III are twice more abundant in the myocardium of both atria than ventricles.59,60 Similarly, collagen I and III content is higher in the right heart compared to the left.59,60 In contrast to intraventricular differences which are already obvious during the first trimester of human development,55 differences in collagen expression between heart chambers only become apparent after birth, coinciding with the first respiration of the newborn and transition to independent systemic circulation resulting in pressure differences between the four cardiac chambers.59
In adult hearts, collagen VI has an overlapping expression pattern with collagen I and III, although fibers are much finer. To assemble into a complex fibrillary network, collagen VI fibers orientate in perpendicular orientation to collagen I and III.46 Collagen IV is the only collagen secreted by CMs and the majority of collagen IV associates with laminin and fibronectin in the basal membrane of single atrial or ventricular CMs.46,61–63 In human adult ventricular myocardium, collagen IV, laminin and fibronectin span the inner lining of the t-tubular network, thereby preventing collapse of t-tubules during cardiac contraction.45–47 In the adult heart, elastin fibers assemble into an irregular network with fibers parallel to the long axis of muscle fibers. Importantly, not only the elastin content, but also fiber length is at least twice as long in the ECM of the right atria and ventricle compared to that of the left heart compartments.60,64 Moreover, as opposed to irregular, less interconnected and more circular elastin in the left atrium, straight, parallel elastic fiber strands interconnect into a highly organized fibrillary scaffold in the right atrium.60 Differences between right and left atrium might be due to diverse biomechanical stimulation of cardiac fibroblasts or different cell origins, but need further investigation for clarification.60
With increasing age the ratio of thick, densely packed collagen and elastin fibers increase in all chambers of the heart, but predominantly in the left ventricle.64–66
The sinoatrial node is a specialized region in the posterior wall of the right atrium responsible for the initiation of the electrical impulse during each cardiac contraction cycle. The uniformly distributed bundles of collagen fibers are only sparsely dispersed within the human sinoatrial node during embryonic development, but comprise about 40% of the entire nodal volume after birth and up to ∼70% in adult hearts to form a dense regular framework. In rats, fibroblasts in the sinoatrial node predominantly secrete collagen I and III,67 but it is not confirmed which collagens predominate in the human sinoatrial node. The collagen network of the sinoatrial node, is key to the mechanical support of nodal CMs, which rely on extra support because of their underdeveloped contractile machinery when compared to CMs of the working myocardium. The dense collagen network, which isolates groups of sinus node cells plays a negative role in maturation by limiting contact between groups of sinoatrial CMs, as well as sinoatrial CMs and their adjacent atrial CMs.65,68,69 In combination with fatty tissues, collagen may also be important in insulating pacemaker automaticity from surrounding atrial CMs.70
Matrix changes have not only been associated with cardiac development, but also various cardiac diseases. Post myocardial infarction around the infarct zone or in fibrotic scar tissue of hearts with cardiomyopathy, not only enhanced deposition of collagen type I, but also III, IV and VI, as well as fibronectin and laminin has been observed.59,71–76 Interestingly, also permanent atrial fibrillation leads to an increase in collagen I, III and IV in both atria.63,77 No gender differences in collagen content or distribution have been detected during development or later.41,59,65
Desired biomaterial properties for cardiac tissue engineering and in vitro modeling of hPSC-CMs
CMs display a high sensitivity to environmental factors, such as elasticity, geometry and topography78,79 and extracellular substrates have already been shown to induce some aspects of cardiac maturity.32,36 In order to emulate the native cardiac environment, the preferred biomaterial needs to meet the following essential criteria:
(1) Long-term elasticity and mechanical strength (suitable stiffness) to sustain the high repetitive mechanical stress and non-linear elasticity of contracting muscle
(2) Adaptable biodegradability long enough to facilitate cell attachment and bear the proteolytic activity of diseased myocardium, while avoiding a persisting response against a foreign structure
(3) Biocompatibility that is not toxic for the cells and supports cell survival
(4) Structural facilitation of neovascularization and remodeling
(5) Controllable electrical properties that do not interfere with the electrical conductance of the action potential
(6) Outside-in-signaling enabling optimal CM attachment, survival, growth, maturation and function in vitro, and support of functional cardiac contraction and integration into the host tissue after implantation in vivo
Natural and synthetic biomaterials
Although a variety of biomaterials have been used for tissue-engineering replacements for different tissues, including bone, cartilage, tendon or skin, lungs and bladder,80 the ability to utilize natural or synthetic biomaterials for cardiac repair has proven more challenging than originally anticipated.
The use of natural biomaterials for engineering of tissue constructs shows many obvious benefits for mimicking endogenous tissues and organs. Nonetheless, important drawbacks of natural materials are their limited availability and shelf life, as well as batch-to-batch variation and high costs. Even more importantly, reproducible scaffold design from pure natural materials often can be challenging. Alternatively, synthetic polymers have adjustable mechanical and degradation properties and unlimited availability. Nevertheless, in contrast to natural biomaterials, synthetic materials have reduced biocompatibility and may provoke adverse rejection responses. Especially for the in vitro generation of soft tissue, such as mechanically challenging cardiac tissue, only few synthetic materials with appropriate combination of biodegradability, biocompatibility and mechanical properties without compromising cellular function of CMs have been applied so far.80–82 Selection of natural and synthetic biomaterials for cardiac tissue engineering are presented in Tables 2 and 3, respectively.
Table 2 Selection of natural polymers
|
Structure |
Origin |
Properties |
Gelatin |
Fine fibrillary scaffold |
Hydrolysis of collagen |
Deforms rapidly, low tensile strength, rapidly bioresorbable |
Matrigel |
Hydrogel |
Secretion by mouse sarcoma cells: Laminin, collagen IV, heparin sulfate proteoglycans, entactin/nidogen |
Resistance against remodeling |
Fibrin |
Fibrillary scaffold |
Plasma-glycoprotein for blood clotting Fibrinogen as precursor |
Tunable properties and quickly remodeled |
Vitronectin |
Single or two chain structure |
Plasma glycoprotein present in ECM and bone |
Quick adsorption to surfaces, considerable charge heterogeneity |
Hyaluronic acid |
Long polymer of disaccharides |
Non-sulfated glycosaminoglycan |
Energetically stable |
Alginate |
Linear co-polymer |
Polysaccharide isolated from the cell wall of algae |
Hydrophilic and forms viscous hydrogel when bound to water. |
Table 3 Selection of synthetic polymers
|
|
Approval for clinical application |
Application |
Properties |
Poly-esters |
Poly-glycolic acid (PGA) |
Approved by the FDA |
Re-absorbable suture material |
Biodegradable, hydrophobic and processability |
Poly-lactic acid (PLA) |
Approved by the FDA |
Rigid thermoplastic materials |
Biodegradable, hydrophobic and processability |
Co-polymers poly-lactic-glycolic acid (PLGA) |
Approved by the FDA |
Scaffold for controlled drug release |
Biodegradable, hydrophobic and processability |
Poly-lactones |
Poly-caprolactone (PCL) |
Approved by the FDA |
Implantable drug delivery |
Semi-crystalline, biodegradable, slow degradation and hydrophobic |
Carboxylated-PCL (cPCL) |
Negatively-charged |
Elastomers |
Poly-dimethyl-siloxane (PDMS) |
Approved by the FDA |
Medical devices |
Viscoelastic |
Poly-glycerol sebacate (PGS) |
Approved by the FDA |
Nerve and vascular tissue engineering |
Bioresorbable, tunable elasticity |
PEG |
Poly-ethylene glycol |
Approved by the FDA |
PEGylated drugs |
Biocompatible, hydrophilic, and repellent |
Scaffold-mediated engineered cardiac tissues
Natural materials as ECM and multicellular approaches in human cardiac tissues engineered from hPSC-CMs
To generate cardiac tissues which include the natural ECM components in a developmentally and physiologically relevant organization, approaches have been undertaken to decellularize cardiac tissue, resulting in a 3D cell-free cardiac ECM meshwork, followed by repopulation with cardiac cells or their progenitors in order to study processes like survival, migration, proliferation, maturation and communication. Repopulation of a decellularized mouse heart with hPSC-derived KDRlow/C-KITneg cardiovascular progenitor cells (CPCs) with the capacity to differentiate into CMs, smooth muscle cells and endothelial cells allowed formation of a bioartificial heart suitable for analyzing ECG traces, contraction and drug response.83 Here, stable interaction between the ECM of the decellularized heart allowed about 10–15% of the CPSs to be retained in the natural cardiac ECM meshwork. These CPCs formed a revascularized spontaneously beating tissue within 20 days after repopulation. Interestingly, endothelial cells formed a single layer along the endocardial surface, as well as the inner surface of small coronary vessels suggesting that the natural ECM effectively supported correct arrangement of in situ differentiated cardiac cells. Although these studies indicate the importance of a natural scaffold of ECM components for the formation of 3D cardiac tissue, alternative strategies are required for controlled production of cardiac tissues, since material source is limited and morphology is difficult to adjust to the desired shape of the tissue. Instead of using a complex ECM network, many approaches for generation of cardiac tissues are based on only a few components or a mixture thereof. Several features attributed to maturation of hPSC-CMs have been achieved in engineered 3D human cardiac tissues utilizing fibrin in combination with matrigel, a mixture of laminin together with collagen IV and proteoglycan derived from mouse sarcoma cells, as ECM material.84–89 Fibrin is an insoluble fibrous protein, involved in blood clotting and wound healing, with little presence in the healthy fetal or adult heart. Instead, increased plasma levels of fibrin have been acknowledged as important cardiovascular risk factor.90 However, practical reasons, such as tunable physical and mechanical properties, as well as biodegradability, made fibrin the protein of choice for tissue engineering. Nevertheless, fibrin requires the combination with matrigel to support survival of CMs. Consistent with enhanced expression of genes important for cardiac contractile function and calcium handling, sarcomeres in cardiac tissues with fibrin as scaffold material were highly organized and evenly distributed with a longitudinal orientation along force lines and enhanced sarcomere length of approximately 1.6 μm
86 and 2.1 μm.88 Structural maturation led to a sustained contraction force of ∼0.15 mN
86 or ∼3 mN
88 and higher conduction velocities (10 cm s−1 up to 25 cm s−1 with increased hPSC-CM purity).88 Interestingly, hPSC-CMs in heterogeneous cardiac tissues, typically consisting of hPSC-CMs mixed with fibroblasts and other non-CMs, developed immature t-tubular-like structures.86 T-tubules are a manifestation of CM maturation typically absent in hPSC-CMs. However, as evidenced by metabolic phenotype, sarcomeric organization, intercellular connection, electrical function and low sensitivity to calcium, 3D cardiac tissues failed to induce full maturation. Continuous electrical pacing29,87 or mechanical load89,91 by stretching had additive positive effect on CM morphology, as proven by sarcomeric organization, transcriptional profile and contraction force. Recently, it was shown that physical conditioning by electromechanical stimulation of early-stage hPSC-derived CMs in fibrin hydrogel cardiac tissues with supporting dermal fibroblasts induces maturation to an adult-like stage regarding gene expression, ultrastructural organization, such as physiological sarcomere length of 2.2 μm, density of mitochondria and t-tubules, as well as oxidative metabolism, positive force–frequency relationship and functional calcium handling. However, electromechanics did not reach maturity seen in adult human myocardium.92
Since fibrin only has a minor role in the cardiac ECM and therefore may be less optimal as ECM component for CMs in engineered cardiac tissues, it is well possible that collagens may be more appropriate for cardiac tissue engineering based on cardiac expression of ECM proteins. However, in contrast to cardiac tissues with fibrin as scaffold, cardiac tissues with collagen require at least 25% of non-CMs.86 Depending on the quantification method, the majority of non-CMs in the heart are either cardiac fibroblasts which play a key role in the production and remodeling of cardiac ECM proteins, including collagen I and III, fibronectin and laminin93,94 or endothelial cells.95 Importantly, in scaffold-free cardiac tissues, only co-culture with adult cardiac fibroblasts, but not fibroblasts of dermal origin, successfully improved CM maturity, as evidenced by enhanced calcium signaling and amended contractile response to inotropic agents.96 This indicates that the origin of cells plays an important role for proper CM function and suggests that co-culture with cardiac cells such as endothelial cells, smooth muscle cells and fibroblasts are required to closely mimic the native cardiac environment and induce CMs maturity. While the co-culture of hPSC-CMs with irradiated human foreskin fibroblasts or hPSC-derived fibroblasts yielded synchronously contracting engineered cardiac tissues based on collagen I in combination with matrigel,97,98 only treatment of hPSC-CMs with ascorbic acid in combination with mechanical stimulation yielded some degree of structural maturation with a contraction force of 4.4 mN mm−2 and a conduction velocity of up to 4.9 cm s−1;97 similarly alignment of collagen I and electrical stimulation yielded some aspects of maturation, such as increased transcriptional activity of genes encoding for structural proteins and high conduction velocity (25 cm s−1).98
Not only the presence of fibroblasts, but also endothelial or smooth muscle cells has been demonstrated to advance structural or electrical maturity of hPSC-CMs.28,99,100 However, based on gene expression patterns and morphological characteristics, such as increased cell surface area with well-defined Z discs and nascent intercalated discs, the maturation of hPSC-CMs was only partially accomplished. hPSC-CMs maturity was further enhanced by electrical stimulation as indicated by improved calcium handling. Nonetheless, hPSC-CMs did not develop crosslinking of myosin thick filaments, so-called M-bands, nor t-tubules.29
Similarly, the inclusion of hPSC-derived vascular endothelial and mural cells (smooth muscle cells and pericytes) into collagen I-matrigel scaffold-based 3D cardiac tissues especially led to structural maturation in hPSC-CMs, which was indicated by organized mitochondria localized between myofibers and enhanced expression of structural proteins, such as β-MHC. Together this led to more organized myofibrillar structure with highly aligned, parallel sarcomeres that demonstrated distinct Z-lines between adjacent sarcomeres, thin filaments (I-bands) and thick filaments (A-bands), but no thick filament crosslinking (M-bands). This resulted in medium force generation (average force 0.62 mN mm−2). Despite immature calcium signaling, structural maturation allowed multicellular engineered 3D cardiac tissues to sustain high frequency pacing by maintaining higher active force.101
Under defined culture conditions where all components are known and fully defined (without matrigel or serum), using a selected cocktail of growth factors, FGF-2, IGF-1, TGF-β, and extracellular calcium led to improved CM maturity in collagen I-based 3D cardiac tissues with hPSC-CMs and fibroblasts, as evidenced by increased sarcomeric organization with defined M-bands and a sarcomere size of 1.9 μm, yielding a positive force-frequency behavior with a contraction force of 6 mN mm−2 and N-cadherin-positive intercalated disc-like structures. However, despite some improvement, the transcriptional profile was comparable to fetal heart at 13 weeks of development. Unexpectedly, laminin and fibronectin failed to improve function of 3D cardiac tissues.102 Together this indicates that collagen I or fibrin may not be sufficient for mimicking the ECM in order to engineer cardiac tissues in vitro. See Table 4 for a summary of CM properties in tissues with natural materials.
Table 4 Properties of CMs in human cardiac tissues engineered with natural materials as ECM
Synthetic materials as supportive scaffold in human engineered cardiac tissues
A small range of synthetic materials has been tested as alternative for natural scaffold materials in engineered cardiac tissues from hPSC-CMs. Porous scaffolds engineered from poly(2-hydroxyethyl methacrylate-co-methacrylic acid) (pHEMA-co-MAA) enhanced selected survival and increased proliferation of hPSC-CMs in mixed cardiac tissues, but failed to induce maturity.103 In combination with natural materials, such as collagen, gelatin, matrigel or vitronectin, for functionalization, synthetic polymers like PEG, PCL, c-PCL, PLGA, polyethylene and PGS successfully supported viability and permitted cell adhesion, spontaneous contraction and also migration of hPSC-CMs in vitro or after transplantation in vivo.104–109 Precisely aligned hPSC-CMs on micropatterned PLGA (∼30 μm), coated with gelatin, and matrigel-coated wrinkled poly-ethylene, exhibited accelerated conduction velocity (PLGA: velocity along transverse direction 4.60 ± 1.91 cm s−1; along longitudinal direction 2.31 ± 0.95 cm s−1) (poly-ethylene: velocity along transverse direction 3.10 ± 0.6 cm s−1; along longitudinal direction 5.40 ± 1.40 cm s−1) and reduced predisposition to arrhythmia in optical mappings in vitro. Analogous results on PLGA109 and poly-ethylene108 suggest that the decreased sensitivity to arrhythmia can be attributed to the physical alignment of the CMs on micromolded grooves and not the substrate matrix. Interestingly, a co-polymer of 4% PEG and 96% PCL (coated with vitronectin), was able to induce expression of genes attributed to cardiac maturity and improved some functional aspects of cardiac maturation, such as organized sarcomeres, enhanced contraction force (221.9 nM) and mitochondrial function, as well as increased expression of structural proteins, intermediate filaments, isoform switch from the fetal ssTnI to the postnatal cTnI, mitochondrial function, calcium handling and contractility during in vitro culture.106
Synthetic biodegradable, porous PLLA/PLGA scaffolds in combination with matrigel allowed survival and synchronous contraction of hPSC-CMs in engineered 3D human cardiac tissues.110 Importantly, inclusion of endothelial cells into the tissue had an advantageous effect on CM proliferation. However, prominent vascularization occurred only in multicellular tissues with hPSC-CMs, endothelial cells and embryonic fibroblasts. Interestingly, hPSC-CMs within vascularized tissues exhibited features of maturation, such as increased sarcomeric organization and increased functional coupling.110
PEGylated fibrinogen is a biosynthetic and rapidly biodegradable hydrogel that photo-polymerizes in response to low intensity, long wave UV-light.80,111–113 Importantly, physical and mechanical properties, such as matrix stiffness, can accurately be regulated by the ratio of synthetic PEG to biological active fibrinogen or the amount of cross linker.80,111–113 PEGylated fibrinogen hydrogels were the first biosynthetic materials to promote survival and reorganization of isolated neonatal rat CMs, as well as hPSC-CMs into functional contracting tissues without negatively affecting their contractile phenotype even after several weeks.80,112,113 Typical response to chronotropic agents, carbamylcholine and the beta-adrenergic agonist isoproterenol, validated responsiveness to cardiac drugs.80 Engineered cardiac patches with PEGylated fibrinogen as scaffold for hPSC-CMs abridged adverse cardiac remodeling and proved beneficial for ventricular performance post myocardial infarction due to improved fractional shortening.113 By preserving contractile function, promoting some features of maturation in hPSC-CMs, PEGylated fibrinogen may serve as promising biosynthetic material for engineering 3D in vitro cardiac tissues.
Recent advances in the design of hydrated polymeric hydrogels renders them very useful for mimicking the native ECM environment and controlled tissue engineering either for in vitro applications or for in vivo tissue regeneration. In general, hydrogels have a high water content, a high biocompatibility and elasticity and consist of either natural materials such as hyaluronic acid, collagen and alginate, or synthetic polymers such as PEG. The chemical, mechanical and degradable properties of hydrogels can be modified and biological processes, such as proliferation, differentiation, migration and maturation can be influenced by incorporation and crosslinking of bioactive molecules, indicating the versatile nature of hydrogels. It goes beyond the scope of this review to discuss all the latest developments in hydrogel technology and their possible applications for regenerative medicine and in vitro assays. Excellent reviews on this topic have been published elsewhere.114
As mentioned before, it has become clear that it is important to mimic the 3D microenvironment of the native cardiac tissue, ensuring dynamic interplay between cardiac cells, ECM components, and bioactive molecules in an optimal structural architecture and topographic organization with appropriate biomechanical (elasticity and stiffness) and electrical conductive properties Several innovative approaches have been followed in order to generate functional 3D cardiac tissues using engineered 3D fiber-based scaffolds or hydrogel-based. Recently, melting electrowriting, an electrohydrodynamic printing technology, was used to fabricate stretchable microfiber scaffolds with hexagonal microstructures using medical grade polycaprolactone as a polymer. Interestingly, these printed scaffolds supported and enhanced maturation of hPSC-derived CMs. Moreover, these scaffolds were also successfully applied on a beating porcine heart while maintaining their structure.115 Although these hexagonal structures clearly demonstrated advantages in comparison to rectangular scaffolds, the pores are much larger (approximately 20-fold) than the honeycomb structure of the heart. It remains to be seen whether these scaffolds will support correctly organized multicellular cardiac constructs for optimal cardiac function and intercellular communication. See Table 5 for a summary of CM properties in cardiac tissues with synthetic materials.
Table 5 Properties of CMs in human cardiac tissues engineered with synthetic materials as ECM
More advanced cardiac models
It is increasingly recognized that controlled and defined 3D tissue engineering, mimicking the native myocardial architecture and micro-environment lead to changes in morphological, molecular and functional characteristics that are more comparable to the adult human heart. In addition to the 3D organization of cardiac tissue, creating a functional hollow cardiac chamber, or a multi-chambered heart, capable of ejecting fluid against afterload pressure in synergy while receiving electrical signals from pacemaker cells, would be significant step closer to a functional human heart. As mentioned earlier, in a first attempt to create a human beating heart, hPSC-derived cardiac progenitor cells were able to repopulate and differentiate to contracting cardiomyocytes in perfused ECM scaffolds from decellularized mouse hearts.83 Nevertheless, lack of a specific cardiac cell types (such as cardiac fibroblasts) and inappropriate cell composition may explain why generated mechanical force was not synchronized and insufficient to pump blood. As an alternative approach, hollow heart tubes were generated by using either chitosan, a biocompatible and biodegradable aminopolysaccharide derived from chitins, or a decellularized goat artery, as tubular structures.116 Cardiac tissues were generated from primary rat heart cultures mixed in a fibrin gel and seeded as a monolayer on PDMS. Anchoring points allowed compaction of cardiac tissues in defined shapes, which were subsequently wrapped around the tubular structure. These tissue-engineered heart pumps displayed rhythmic electrical activity and contractions and small intraluminal pressure peaks could be detected.117,118
To generate highly advanced models more closely resembling cardiac geometry and to allow measurement of hemodynamic cardiac output, Li et al. created for the first time a human ventricle-like cardiac organoid chamber (hvCOC).119 In order to achieve this, dissociated hPSC-derived CMs and dermal fibroblasts (for compaction) were reaggregated in the presence of a mixture of collagen I and Matrigel and were transferred to an agarose mold with a centrally placed inflatable balloon, which allowed to form a 3D human engineered cardiac organoid. HvCOCs were able to pump fluid and ejection fraction and generation of pressure volume loops could be measured in these heart models. Although these results are very promising, cardiac maturation and function may be improved if cardiac fibroblasts will be used instead of dermal fibroblasts (vide supra). Macqueen and colleagues followed another approach to generate a ventricle-like tube.120 In this study scaffolds were made by pull spinning a mixture of PCL and gelatin onto a mandrel in the shape of an ellipsoidal ventricle. Following sterilization by UV, scaffolds were coated with fibronectin for the attachment of hPSC-derived CMs. Following suturing of the ventricular engineered tissue on a tubing system ejection fraction and pressure volume loops could be measured and were responsive to pharmaceutical compounds. Although it is clear that further improvements are required, in this regard it is essential to mention that these ventricular tissues are only one cell layer thick which is an important limitation of the study and does not resemble a human heart, it is promising that clinically relevant parameters could be measured in these ventricle-like tubes.
Advances in 3D bioprinting offers new opportunities to create 3D tissues by precise positioning of biomaterial and defined cell-types using scaffold-based or scaffold-free approaches building the cardiac tissue layer by layer or by printing 3D cardiac spheres in a spatially controlled manner.121,122 Increased knowledge of printing technologies, cell-interactive and stimuli-responsive hydrogels, viability of cardiac cells and defined differentiation and characterization of cardiac progenitor and cardiovascular cells facilitates the formation of native-like cardiac tissue over time, which will further support of 4D bioprinting approaches.
Conclusions and future directions
Although differences in experimental approaches, including timing, origin of cells, use of biomaterials, culture conditions, engineering technologies and endpoint evaluation, make it difficult to directly compare results from different studies, it is evident that the level of cardiomyocyte maturation can be further increased in more advanced 3D tissues when compared to standard monolayer or aggregate differentiation cultures. Nevertheless, even in multicellular 3D cardiac tissues, hPSC-CMs fail to acquire an adult phenotype. So far, little attention has been paid to mimic the native cardiac microenvironment. Instead the majority of cardiac tissues are based on fibrin or collagen I, a matrix which is far from comparable to native cardiac environment, consisting of a complex scaffold of collagen I, III, IV and VI and also laminin, fibronectin, fibrillin and elastin, as well as other components. Temporal and spatial cues from cardiac cells and their microenvironment during human cardiac development (from fetal to adult stages), exemplified by localized and progressive build-up of cardiac ECM components, are essential for a proper understanding of cardiomyocyte maturation. Mimicking the same dynamic stages with gradual assembly of matrix proteins recapitulating cardiac native environment may contribute to maturation of hPSC-CMs in vitro, which may be dependent on the self-organization of the engineered cardiac tissue in combination with physical factors such as mechanical load and electrical stimuli. In this context, it is intriguing to speculate that hPSC-derived cardiac cells, either progenitor cells or functional subtypes, and biomaterials may be positioned in the appropriate architecture by 3D-bioprinting technology as discussed above. Although many hurdles need to be overcome before functional vascularized cardiac tissues can be printed (including survival of cells and printing resolution), recent advances have shown the feasibility to generate viable organized tissues.122–124 Advances in differentiation, characterization and purification of hPSC-derived cardiac cells along with those in biomaterials, polymers and tissue engineering will facilitate controlled and defined construction of heart tissue, using fetal and adult stages of the human heart as a molecular, morphological and functional blueprint, which undoubtedly has a major impact on regenerative medicine. In addition, with this in prospect, we expect to gain insight to what level of organization and maturation of engineered heart tissues can be achieved in vitro and to what extent this will be sufficient to reveal key aspects of cardiac disease using patient-derived hPSCs. With the development of innovative smart hydrogels and other materials new opportunities have arisen for dynamic interaction with cells and tissues in a temporal and spatial manner. In combination with other recent advances in other fields such as microfabrication, nanotechnology, electrical engineering, biosensors, membranes, chemistry, membrane sciences, 3D printing, etc., new opportunities have been created to mimic tissue or organ function in small (normally micrometre- or millimetre-sized) chambers or chip-like devices. In these so-called “organs-on-chips” the microenvironment can be controlled and biological and functional readouts can be implemented in a flexible and multiplex manner.125 Increased understanding of how ECM components and synthetic polymers affect biological responses and function under physiological and pathophysiological conditions, will facilitate development of predictable human in vitro models for safety pharmacology and drug discovery and generation of multicellular tissue constructs for clinical use.
Conflicts of interest
R. P. is a cofounder of Pluriomics (Ncardia).
Acknowledgements
We would like to thank Aisen De sa Vivas for critical reading of the document and Christian B Schwach for illustrations.
References
- O. Caspi, I. Itzhaki, I. Kehat, A. Gepstein, G. Arbel and I. Huber,
et al. In vitro electrophysiological drug testing using human embryonic stem cell derived cardiomyocytes, Stem Cells Dev., 2009, 18(1), 161–172 CrossRef CAS PubMed.
- S. R. Braam, L. Tertoolen, A. van de Stolpe, T. Meyer, R. Passier and C. L. Mummery, Prediction of drug-induced cardiotoxicity using human embryonic stem cell-derived cardiomyocytes, Stem Cell Res., 2010, 4(2), 107–116 CrossRef CAS PubMed.
- K. R. Doherty, D. R. Talbert, P. B. Trusk, D. M. Moran, S. A. Shell and S. Bacus, Structural and functional screening in human induced-pluripotent stem cell-derived cardiomyocytes accurately identifies cardiotoxicity of multiple drug types, Toxicol. Appl. Pharmacol., 2015, 285(1), 51–60 CrossRef CAS PubMed.
- H. M. Himmel, Drug-induced functional cardiotoxicity screening in stem cell-derived human and mouse cardiomyocytes: Effects of reference compounds, J. Pharmacol. Toxicol. Methods, 2013, 68(1), 97–111 CrossRef CAS PubMed.
- P. Liang, F. Lan, A. S. Lee, T. Gong, V. Sanchez-Freire and Y. Wang,
et al. Drug screening using a library of human induced pluripotent stem cell-derived cardiomyocytes reveals disease-specific patterns of cardiotoxicity, Circulation, 2013, 127(16), 1677–1691 CrossRef CAS PubMed.
- H. Liang, M. Matzkies, H. Schunkert, M. Tang, H. Bonnemeier and J. Hescheler,
et al. Human and murine embryonic stem cell-derived cardiomyocytes serve together as a valuable model for drug safety screening, Cell. Physiol. Biochem., 2010, 25(4–5), 459–466 CrossRef CAS PubMed.
- E. G. Navarrete, P. Liang, F. Lan, V. Sanchez-Freire, C. Simmons and T. Gong,
et al. Screening drug-induced arrhythmia events using human induced pluripotent stem cell-derived cardiomyocytes and low-impedance microelectrode arrays, Circulation, 2013, 128, S3–S13 CrossRef CAS PubMed.
- A. Sharma, C. Marceau, R. Hamaguchi, P. W. Burridge, K. Rajarajan and J. M. Churko,
et al. Human induced pluripotent stem cell-derived cardiomyocytes as an in vitro model for coxsackievirus B3-induced myocarditis and antiviral drug screening platform, Circ. Res., 2014, 115(6), 556–566 CrossRef CAS PubMed.
- T. Shinozawa, K. Imahashi, H. Sawada, H. Furukawa and K. Takami, Determination of Appropriate Stage of Human-Induced Pluripotent Stem Cell-Derived Cardiomyocytes for Drug Screening and Pharmacological Evaluation In Vitro, J. Biomol. Screening, 2012, 17(9), 1192–1203 CrossRef PubMed.
- D. R. Talbert, K. R. Doherty, P. B. Trusk, D. M. Moran, S. A. Shell and S. Bacus, A multi-parameter in vitro screen in human stem cell-derived cardiomyocytes identifies ponatinib-induced structural and functional cardiac toxicity, Toxicol. Sci., 2015, 143(1), 147–155 CrossRef CAS PubMed.
- M. Stancescu, P. Molnar, C. W. McAleer, W. McLamb, C. J. Long and C. Oleaga,
et al. A phenotypic in vitro model for the main determinants of human whole heart function, Biomaterials, 2015, 60, 20–30 CrossRef CAS PubMed.
- J. J. H. Chong, X. Yang, C. W. Don, E. Minami, Y.-W. Liu and J. J. Weyers,
et al. Human embryonic-stem-cell-derived cardiomyocytes regenerate non-human primate hearts, Nature, 2014, 510(7504), 273–277 CrossRef CAS PubMed.
- L. W. van Laake, R. Passier, J. Monshouwer-Kloots, A. J. Verkleij, D. J. Lips and C. Freund,
et al. Human embryonic stem cell-derived cardiomyocytes survive and mature in the mouse heart and transiently improve function after myocardial infarction, Stem Cell Res., 2007, 1(1), 9–24 CrossRef PubMed.
- M. a. Laflamme, K. Y. Chen, A. V. Naumova, V. Muskheli, J. a. Fugate and S. K. Dupras,
et al. Cardiomyocytes derived from human embryonic stem cells in pro-survival factors enhance function of infarcted rat hearts, Nat. Biotechnol., 2007, 25(9), 1015–1024 CrossRef CAS PubMed.
- Y. Shiba, T. Gomibuchi, T. Seto, Y. Wada, H. Ichimura and Y. Tanaka,
et al. Allogeneic transplantation of iPS cell-derived cardiomyocytes regenerates primate hearts, Nature, 2016, 538(7625), 388–391 CrossRef CAS PubMed.
- C. Mummery, J. Zhang, E. Ng, D. Elliott, A. G. Elefanty and T. J. Kamp, Differentiation of Human ES and iPS Cells to Cardiomyocytes: A Methods Overview, Circ. Res., 2012, 111(May), 344–358 CrossRef CAS PubMed.
- P. W. Burridge, E. Matsa, P. Shukla, Z. C. Lin, J. M. Churko and A. D. Ebert,
et al. Chemically defined generation of human cardiomyocytes, Nat. Methods, 2014, 11(8), 855–860 CrossRef CAS PubMed.
- H. D. Devalla, V. Schwach, J. W. Ford, J. T. Milnes, S. El-Haou and C. Jackson,
et al. Atrial-like cardiomyocytes from human pluripotent stem cells are a robust preclinical model for assessing atrial-selective pharmacology, EMBO Mol. Med., 2015, 7(4), 394–410 CrossRef CAS PubMed.
- Z. Laksman, M. Wauchop, E. Lin, S. Protze, J. Lee and W. Yang,
et al. Modeling Atrial Fibrillation using Human Embryonic Stem Cell- Derived Atrial Tissue, Sci. Rep., 2017, 7, 1–11 CrossRef CAS PubMed.
- V. Schwach, A. O. Verkerk, M. Mol, J. J. Monshouwer-Kloots, H. D. Devalla and V. V. Orlova,
et al. A COUP-TFII Human Embryonic Stem Cell Reporter Line to Identify and Select Atrial Cardiomyocytes, Stem Cell Rep., 2017, 9(6), 1–15 Search PubMed.
- F. Pei, J. Jiang, S. Bai, H. Cao, L. Tian and Y. Zhao,
et al. Chemical-defined and albumin-free generation of human atrial and ventricular myocytes from human pluripotent stem cells, Stem Cell Res., 2017, 19, 94–103 CrossRef CAS PubMed.
- M. J. Birket, M. C. Ribeiro, A. O. Verkerk, D. Ward, A. R. Leitoguinho and S. C. den Hartogh,
et al. Expansion and patterning of cardiovascular progenitors derived from human pluripotent stem cells, Nat. Biotechnol., 2015,(July), 1–12 Search PubMed.
- S. I. Protze, J. Liu, U. Nussinovitch, L. Ohana, P. H. Backx and L. Gepstein,
et al. Sinoatrial node cardiomyocytes derived from human pluripotent cells function as a biological pacemaker, Nat. Biotechnol., 2016, 35(December), 1–16 Search PubMed.
- J. A. Guadix, V. V. Orlova, E. Giacomelli, M. Bellin, M. C. Ribeiro and C. L. Mummery,
et al. Human Pluripotent Stem Cell Differentiation into Functional Epicardial Progenitor Cells, Stem Cell Rep., 2017, 9(6), 1754–1764 CrossRef CAS PubMed.
- D. Iyer, L. Gambardella, W. G. Bernard, F. Serrano, V. L. Mascetti and R. a. Pedersen,
et al. Robust derivation of epicardium and its differentiated smooth muscle cell progeny from human pluripotent stem cells, Development, 2015, 142(8), 1528–1541 CrossRef CAS PubMed.
- A. D. Witty, A. Mihic, R. Y. Tam, S. A. Fisher, A. Mikryukov and M. S. Shoichet,
et al. Generation of the epicardial lineage from human pluripotent stem cells, Nat. Biotechnol., 2014, 32(10), 1026–1035 CrossRef CAS PubMed.
- V. V. Orlova, F. E. van den Hil, S. Petrus-Reurer, Y. Drabsch, P. Ten Dijke and C. L. Mummery, Generation, expansion and functional analysis of endothelial cells and pericytes derived from human pluripotent stem cells, Nat. Protoc., 2014, 9(6), 1514–1531 CrossRef CAS PubMed.
- E. Giacomelli, M. Bellin, L. Sala, B. J. van Meer, L. G. J. Tertoolen and V. V. Orlova,
et al. Three-dimensional cardiac microtissues composed of cardiomyocytes and endothelial cells co-differentiated from human pluripotent stem cells, Development, 2017, 144, 1008–1017 CrossRef CAS PubMed.
- S. S. Nunes, J. W. Miklas, J. Liu, R. Aschar-Sobbi, Y. Xiao and B. Zhang,
et al. Biowire: a platform for maturation of human pluripotent stem cell-derived cardiomyocytes, Nat. Methods, 2013, 10(8), 781–787 CrossRef CAS PubMed.
- M. C. Ribeiro, L. G. Tertoolen, J. a. Guadix, M. Bellin, G. Kosmidis and C. D'Aniello,
et al. Functional maturation of human pluripotent stem cell derived cardiomyocytes in vitro – Correlation between contraction force and electrophysiology, Biomaterials, 2015, 51, 138–150 CrossRef CAS PubMed.
- C. W. van den Berg, S. Okawa, S. M. Chuva de Sousa Lopes, L. van Iperen, R. Passier and S. R. Braam,
et al. Transcriptome of human foetal heart compared with cardiomyocytes from pluripotent stem cells, Development, 2015, 3231–3238 CrossRef CAS PubMed.
- C. C. Veerman, G. Kosmidis, C. L. Mummery, S. Casini, A. O. Verkerk and M. Bellin, Immaturity of human stem-cell-derived cardiomyocytes in culture: fatal flaw or soluble problem?, Stem Cells Dev., 2015, 24(9), 1035–1052 CrossRef CAS PubMed.
- X. Yang, L. Pabon and C. E. Murry, Engineering Adolescence: Maturation of Human Pluripotent Stem Cell-derived Cardiomyocytes, Circ. Res., 2014, 114(3), 511–523 CrossRef CAS PubMed.
- C. Robertson, D. D. Tran and S. C. George, Concise review: Maturation phases of human pluripotent stem cell-derived cardiomyocytes, Stem Cells, 2013, 31(5), 829–837 CrossRef CAS PubMed.
- C. Denning, V. Borgdorff, J. Crutchley, K. S. A. Firth, V. George and S. Kalra, , et al. Cardiomyocytes from human pluripotent stem cells: From laboratory curiosity to industrial biomedical platform, Biochim. Biophys. Acta, Mol. Cell Res., 2016, 1863(7), 1728–1748 CrossRef CAS PubMed.
- X. Sun and S. S. Nunes, Bioengineering Approaches to Mature Human Pluripotent Stem Cell-Derived Cardiomyocytes, Front. Cell Dev. Biol., 2017, 5(March), 19 Search PubMed.
- A. Chen, E. Lee, R. Tu, K. Santiago, A. Grosberg and C. Fowlkes,
et al. Integrated platform for functional monitoring of biomimetic heart sheets derived from human pluripotent stem cells, Biomaterials, 2014, 35(2), 675–683 CrossRef CAS PubMed.
- M. L. McCain, A. Agarwal, H. W. Nesmith, A. P. Nesmith and K. K. Parker, Micromolded gelatin hydrogels for extended culture of engineered cardiac tissues, Biomaterials, 2014, 35(21), 5462–5471 CrossRef CAS PubMed.
- A. G. Kléber and Y. Rudy, Basic mechanisms of cardiac impulse propagation and associated arrhythmias, Physiol. Rev., 2004, 84(2), 431–488 CrossRef PubMed.
- C. O. Heras-Bautista, A. Katsen-Globa, N. E. Schloerer, S. Dieluweit, O. M. Abd El Aziz and G. Peinkofer,
et al. The influence of physiological matrix conditions on permanent culture of induced pluripotent stem cell-derived cardiomyocytes, Biomaterials, 2014, 35(26), 7374–7385 CrossRef CAS PubMed.
- M. M. H. Marijianowski, C. M. van der Loos, M. F. Mohrschladt and A. E. Becker, The neonatal heart has a relatively high content of total collagen and type I collagen, a condition that may explain the less compliant state, J. Am. Coll. Cardiol., 1994, 23(5), 1204–1208 CrossRef CAS PubMed.
- J. E. Bishop and G. J. Laurent, Collagen turnover and its regulation in the normal and hypertrophying heart, Eur. Heart J., 1995, 16(Suppl C), 38–44 CrossRef CAS PubMed.
- R. R. de Souza, Aging of myocardial collagen, Biogerontology, 2002, 3(3), 325–335 CrossRef CAS PubMed.
- M. Votteler, D. a. C. Berrio, A. Horke, L. Sabatier, D. P. Reinhardt and A. Nsair,
et al. Elastogenesis at the onset of human cardiac valve development, Development, 2013, 140(11), 2345–2353 CrossRef CAS PubMed.
- H. Kim, C. S. Yoon, H. Kim and B.-J. Rah, Expression of extracellular matrix components fibronectin and laminin in the human fetal heart, Cell Struct. Funct., 1999, 24, 19–26 CrossRef CAS PubMed.
- B. Speiser, C. Riess and J. Schaper, The extracellular
matrix in human myocardium: Part I: Collagens I, III, IV, and VI, Cardioscience, 1991, 2(4), 225–232 CAS.
- B. Speiser, D. Weihrauch, C. Riess and J. Schaper, The extracellular matrix in human cardiac tissue. Part II: Vimentin, laminin, and fibronectin, Cardioscience, 1992, 3(1), 41–49 CAS.
- F. Farhadian, F. Contard, A. Sabri, J. L. Samuel and L. Rappaport, Fibronectin and basement membrane in cardiovascular organogenesis and disease pathogenesis, Cardiovasc. Res., 1996, 32(3), 433–442 CrossRef PubMed.
- A. Mittal, M. Pulina, S. Y. Hou and S. Astrof, Fibronectin and integrin alpha 5 play requisite roles in cardiac morphogenesis, Dev. Biol., 2013, 381(1), 73–82 CrossRef CAS PubMed.
- M. Rienks, A. P. Papageorgiou, N. G. Frangogiannis and S. Heymans, Myocardial extracellular matrix: An ever-changing and diverse entity, Circ. Res., 2014, 114(5), 872–888 CrossRef CAS PubMed.
- S. Shichijo and H. Masuda, Isolation and characterization of glycosaminoglycans from ventricles of the human heart, Biochem. Med., 1980, 23(2), 150–158 CrossRef CAS PubMed.
- H. Masuda and S. Smcmjo, Isolation and characterization of glycosaminoglycans from atria of the human heart, Int. J. Biochem, 1981, 13(5), 603–608 CrossRef CAS PubMed.
- B. Shen, M. K. Delaney and X. Du, Inside-out, outside-in, and inside-outside-in: G protein signaling in integrin-mediated cell adhesion, spreading, and retraction, Curr. Opin. Cell Biol., 2012, 24(5), 600–606 CrossRef CAS PubMed.
- K. R. Legate, S. a. Wickström, R. Fässler, R. Fa and S. a. Wickstro, Genetic and cell biological analysis of integrin outside-in signaling Genetic and cell biological analysis of integrin outside-in signaling, Genes Dev., 2009, 23, 397–418 CrossRef CAS PubMed.
- M. Jackson, M. G. Connell and A. Smith, Development of the collagen network of the human fetal myocardium: an immunohistochemical study, Int. J. Cardiol., 1993, 41, 77–86 CrossRef CAS PubMed.
- P. Oliviéro, C. Chassagne, N. Salichon, A. Corbier, G. Hamon and F. Marotte,
et al. Expression of laminin α2 chain during normal and pathological growth of myocardium in rat and human, Cardiovasc. Res., 2000, 46(2), 346–355 CrossRef.
- T. Saetersdal, T. Larsen, S. Rotevatn, H. Dalen and P. Scheie, Fibronectin and laminin in transverse tubules of cardiac myocytes studied by laser confocal microscopy and immunocytochemistry, Histochemistry, 1992, 98(2), 73–80 CAS.
- F. Lu, F. F. Ma, W. Zhang, Y. Li, F. Y. Wei and L. Zhou, Qualitative research of alternatively splice variants of fibronectin in different development stage of mice heart, J. Thorac. Dis., 2015, 7(12), 2307–2312 Search PubMed.
- D. E. Oken and R. J. Boucek, Quantitation of Collagen in Human Myocardium, Circ. Res., 1957, 5(4), 357–361 CrossRef CAS PubMed.
- N. Smorodinova, L. Lantová, M. Bláha, V. Melenovský, J. Hanzelka and J. Pirk,
et al. Bioptic Study of Left and Right Atrial Interstitium in Cardiac Patients with and without Atrial Fibrillation: Interatrial but Not Rhythm-Based Differences, PLoS One, 2015, 10(6), e0129124 CrossRef PubMed.
- T. K. Borg, R. E. Gay and L. D. Johnson, Changes in the distribution of fibronectin and collagen during development of the neonatal rat heart, Collagen Relat. Res., 1982, 2(3), 211–218 CrossRef CAS.
- R. I. Bashey, A. Martinez-Hernandez and S. A. Jimenez, Isolation, Characterization, and Localization of Cardiac Collagen Type VI Associations With Other Extracellular Matrix Components, Circ. Res., 1992, 70(5), 1006–1017 CrossRef CAS PubMed.
- Y.-Y. Jiang, Q. Yang, X.-C. Liu and G.-W. He, Mechanism of Atrial Fibrillation is Related to Changes of Type IV Collagen in Rheumatic Heart Valvular Disease, FASEB J., 2016 Search PubMed , Abstract number 1274.4. Available from: http://www.fasebj.org/content/30/1_Supplement/1274.4.abstract.
- E. T. de Carvalho Filho, C. A. de Carvalho and R. R. de Souza, Age-related changes in elastic fibers of human heart, Gerontology, 1996, 42(4), 211–217 CrossRef CAS PubMed.
- A. M. Alings, R. F. Abbas and L. N. Bouman, Age-related changes in structure and relative collagen content of the human and feline sinoatrial node. A comparative study, Eur. Heart J., 1995, 16(11), 1655–1667 CrossRef CAS PubMed.
- C. R. Gazoti Debessa, L. B. Mesiano Maifrino and R. Rodrigues de Souza, Age related changes of the collagen network of the human heart, Mech. Ageing Dev., 2001, 122(10), 1049–1058 CrossRef CAS PubMed.
- J. Yanni, J. O. Tellez, P. V. Sutyagin, M. R. Boyett and H. Dobrzynski, Structural remodelling of the sinoatrial node in obese old rats, J. Mol. Cell. Cardiol., 2010, 48(4), 653–662 CrossRef CAS PubMed.
- A. Nabipour, Comparative histological structure of the sinus node in mammals, Turk. J. Vet. Anim. Sci., 2012, 36(5), 463–469 Search PubMed.
- T. N. James, Cardiac conduction system: fetal and postnatal development, Am. J. Cardiol., 1970, 25(February), 213–226 CrossRef CAS PubMed.
- T. A. Csepe, A. Kalyanasundaram, B. J. Hansen, J. Zhao and V. V. Fedorov, Fibrosis: A structural modulator of sinoatrial node physiology and dysfunction, Front. Physiol., 2015, 6(Feb), 1–8 Search PubMed.
- M. Pauschinger, D. Knopf, S. Petschauer, a. Doerner, W. Poller and P. L. Schwimmbeck,
et al. Dilated cardiomyopathy is associated with significant changes in collagen type I/III ratio, Circulation, 1999, 99(21), 2750–2756 CrossRef CAS PubMed.
- J. E. Bishop, R. Greenbaum, D. G. Gibson, M. Yacoub and G. J. Laurent, Enhanced deposition of predominantly type I collagen in myocardial disease, J. Mol. Cell Cardiol., 1990, 22(10), 1157–1165 CrossRef CAS PubMed.
- R. Lombardi, S. Betocchi, M. A. Losi, C. G. Tocchetti, M. Aversa and M. Miranda,
et al. Myocardial collagen turnover in hypertrophic cardiomyopathy, Circulation, 2003, 108(12), 1455–1460 CrossRef CAS PubMed.
- Y. Ma, L. E. De Castro Brás, H. Toba, R. P. Iyer, M. E. Hall and M. D. Winniford,
et al. Myofibroblasts and the extracellular matrix network in post-myocardial infarction cardiac remodeling, Pflugers Arch. Eur. J. Physiol., 2014, 466(6), 1113–1127 CAS.
- M. Kitamura, M. Shimizu, H. Ino, K. Okeie, M. Yamaguchi and N. Funjno,
et al. Collagen remodeling and cardiac dysfunction in patients with hypertrophic cardiomyopathy: the significance of type III and VI collagens, Clin. Cardiol., 2001, 24(4), 325–329 CrossRef CAS PubMed.
- V. I. Kapelko, Extracellular matrix alterations in cardiomyopathy: The possible crucial role in the dilative form, Exp. Clin. Cardiol., 2001, 6(1), 41–49 CAS.
- A. Boldt, U. Wetzel, J. Lauschke, J. Weigl, J. Gummert and G. Hindricks,
et al. Fibrosis in left atrial tissue of patients with atrial fibrillation with and without underlying mitral valve disease, Heart, 2004, 90(4), 400–405 CrossRef CAS PubMed.
- T. Nakane, H. Masumoto, J. P. Tinney, F. Yuan, W. J. Kowalski and F. Ye,
et al. Impact of Cell Composition and Geometry on Human Induced Pluripotent Stem Cells-Derived Engineered Cardiac Tissue, Sci. Rep., 2017, 7(March), 45641 CrossRef CAS PubMed.
- Y. Farouz, Y. Chen, A. Terzic and P. Menasche, Concise review: Growing hearts in the right place: On the design of biomimetic materials for cardiac stem cell differentiation, Stem Cells, 2015, 33(4), 1021–1035 CrossRef CAS PubMed.
- K. Shapira-Schweitzer, M. Habib, L. Gepstein and D. Seliktar, A photopolymerizable hydrogel for 3-D culture of human embryonic stem cell-derived cardiomyocytes and rat neonatal cardiac cells, J. Mol. Cell. Cardiol., 2009, 46(2), 213–224 CrossRef CAS PubMed.
- A. K. Patel, A. D. Celiz, D. Rajamohan, D. G. Anderson, R. Langer and M. C. Davies,
et al. A defined synthetic substrate for serum free culture of human stem cell derived cardiomyocytes with improved functional maturity identified using combinatorial materials microarrays, Biomaterials, 2015, 61, 257–265 CrossRef CAS PubMed.
- F. J. O'Brien, Biomaterials & scaffolds for tissue engineering, Mater. Today, 2011, 14(3), 88–95 CrossRef.
- T.-Y. Lu, B. Lin, J. Kim, M. Sullivan, K. Tobita and G. Salama,
et al. Repopulation of decellularized mouse heart with human induced pluripotent stem cell-derived cardiovascular progenitor cells, Nat. Commun., 2013, 4, 2307 CrossRef PubMed.
- S. Schaaf, A. Shibamiya, M. Mewe, A. Eder, A. Stoehr and M. N. Hirt,
et al. Human engineered heart tissue as a versatile tool in basic research and preclinical toxicology, PLoS One, 2011, 6(10), 1–11 CrossRef PubMed.
- A. Stoehr, C. Neuber, C. Baldauf, I. Vollert, F. W. Friedrich and F. Flenner,
et al. Automated analysis of contractile force and Ca2+ transients in engineered heart tissue, Am. J. Physiol.: Heart Circ. Physiol., 2014, 306(9), H1353–H1363 CrossRef CAS PubMed.
- I. Mannhardt, K. Breckwoldt, D. Letuffe-Brenière, S. Schaaf, H. Schulz and C. Neuber,
et al. Human Engineered Heart Tissue: Analysis of Contractile Force, Stem Cell Rep., 2015, 7, 29–42 CrossRef PubMed.
- M. N. Hirt, J. Boeddinghaus, A. Mitchell, S. Schaaf, C. Börnchen and C. Müller,
et al. Functional improvement and maturation of rat and human engineered heart tissue by chronic electrical stimulation, J. Mol. Cell. Cardiol., 2014, 74, 151–161 CrossRef CAS PubMed.
- D. Zhang, I. Y. Shadrin, J. Lam, H. Q. Xian, H. R. Snodgrass and N. Bursac, Tissue-engineered cardiac patch for advanced functional maturation of human ESC-derived cardiomyocytes, Biomaterials, 2013, 34(23), 5813–5820 CrossRef CAS PubMed.
- W. Zhang, C. W. Kong, M. H. Tong, W. H. Chooi, N. Huang and R. A. Li,
et al. Maturation of human embryonic stem cell-derived cardiomyocytes (hESC-CMs) in 3D collagen matrix: Effects of niche cell supplementation and mechanical stimulation, Acta Biomater., 2017, 49, 204–217 CrossRef CAS PubMed.
- J. J. Stec, H. Silbershatz, G. H. Tofler, T. H. Matheney, P. Sutherland and I. Lipinska,
et al. Association of fibrinogen with cardiovascular risk factors and cardiovascular disease in the Framingham Offspring Population, Circulation, 2000, 102(14), 1634–1638 CrossRef CAS PubMed.
- N. L. Tulloch, V. Muskheli, M. V. Razumova, F. S. Korte, M. Regnier and K. D. Hauch,
et al. Growth of engineered human myocardium with mechanical loading and vascular coculture, Circ. Res., 2011, 109(1), 47–59 CrossRef CAS PubMed.
- K. Ronaldson, S. Ma, K. Yeager, T. Chen, L. Song and D. Sirabella, Advanced maturation of human cardiac tissue grown from pluripotent stem cells, Nature, 2018, 556, 239–243 CrossRef PubMed.
- C. Castaldo, F. Di Meglio, R. Miraglia, A. M. Sacco, V. Romano and C. Bancone,
et al. Cardiac fibroblast-derived extracellular matrix (biomatrix) as a model for the studies of cardiac primitive cell biological properties in normal and pathological adult human heart, BioMed Res. Int., 2013, 2013, 352370 Search PubMed.
- P. Zhou and W. T. Pu, Recounting cardiac cellular composition, Circ. Res., 2016, 118(3), 368–370 CrossRef CAS PubMed.
- A. R. Pinto, A. Ilinykh, M. J. Ivey, J. T. Kuwabara, M. L. D′antoni and R. Debuque,
et al. Revisiting cardiac cellular composition, Circ. Res., 2016, 118(3), 400–409 CrossRef CAS PubMed.
- S. M. Ravenscroft, A. Pointon, A. W. Williams, M. J. Cross and J. E. Sidaway, Cardiac Non-myocyte Cells Show Enhanced Pharmacological Function Suggestive of Contractile Maturity in Stem Cell Derived Cardiomyocyte Microtissues, Toxicol. Sci., 2016, 152(1), kfw069 CrossRef PubMed.
- G. Kensah, A. R. Lara, J. Dahlmann, R. Zweigerdt, K. Schwanke and J. Hegermann,
et al. Murine and human pluripotent stem cell-derived cardiac bodies form contractile myocardial tissue in vitro, Eur. Heart J., 2013, 34(15), 1134–1146 CrossRef CAS PubMed.
- N. Thavandiran, N. Dubois, A. Mikryukov, S. Massé, C. A. Simmons and V. S. Deshpande,
et al. Design and formulation of functional pluripotent stem cell-derived cardiac microtissues, Proc. Natl. Acad. Sci. U. S. A., 2013, 111(47), 16973 Search PubMed.
- J. Pasquier, R. Gupta, D. Rioult, J. Hoarau-Véchot, R. Courjaret and K. Machaca,
et al. Coculturing with endothelial cells promotes in vitro maturation and electrical coupling of human embryonic stem cell–derived cardiomyocytes, J. Heart Lung Transplant., 2017, 36(6), 684–693 CrossRef PubMed.
- H. Vuorenpää, K. Penttinen, T. Heinonen, M. Pekkanen-Mattila, J.-R. Sarkanen and T. Ylikomi,
et al. Maturation of human pluripotent stem cell derived cardiomyocytes is improved in cardiovascular construct, Cytotechnology, 2017, 69(5), 785–800 CrossRef PubMed.
- H. Masumoto, T. Nakane, J. P. Tinney, F. Yuan, F. Ye and W. J. Kowalski,
et al. The myocardial regenerative potential of three-dimensional engineered cardiac tissues composed of multiple human iPS cell-derived cardiovascular cell lineages, Sci. Rep., 2016, 6(April), 29933 CrossRef CAS PubMed.
- M. Tiburcy, J. E. Hudson, P. Balfanz, S. F. Schlick, T. Meyer and M.-L. Chang Liao,
et al. Defined Engineered Human Myocardium with Advanced Maturation for Applications in Heart Failure Modelling and Repair, Circulation, 2017, 135(19), 1832–1847 CrossRef CAS PubMed.
- L. R. Madden, D. J. Mortisen, E. M. Sussman, S. K. Dupras, J. A. Fugate and J. L. Cuy,
et al. Proangiogenic scaffolds as functional templates for cardiac tissue engineering, Proc. Natl. Acad. Sci. U. S. A., 2010, 107(34), 15211–15216 CrossRef CAS PubMed.
- Q. Z. Chen, H. Ishii, G. A. Thouas, A. R. Lyon, J. S. Wright and J. J. Blaker,
et al. An elastomeric patch derived from poly(glycerol sebacate) for delivery of embryonic stem cells to the heart, Biomaterials, 2010, 31(14), 3885–3893 CrossRef CAS PubMed.
- X. Wang, Y. W. Chun, L. Zhong, M. Chiusa, D. A. Balikov and A. Y. Frist,
et al. A temperature-sensitive, self-adhesive hydrogel to deliver iPSC-derived cardiomyocytes for heart repair, Int. J. Cardiol., 2015, 190(1), 177–180 CrossRef PubMed.
- Y. W. Chun, D. a. Balikov, T. K. Feaster, C. H. Williams, C. C. Sheng and J.-B. Lee,
et al. Combinatorial polymer matrices enhance in vitro maturation of human induced pluripotent stem cell-derived cardiomyocytes, Biomaterials, 2015, 67, 52–64 CrossRef CAS PubMed.
- B. Xu, Y. Li, X. Fang, G. A. Thouas, W. D. Cook and D. F. Newgreen,
et al. Mechanically tissue-like elastomeric polymers and their potential as a vehicle to deliver functional cardiomyocytes, J. Mech. Behav. Biomed. Mater., 2013, 28, 354–365 CrossRef CAS PubMed.
- J. Wang, A. Chen, D. K. Lieu, I. Karakikes, G. Chen and W. Keung,
et al. Effect of engineered anisotropy on the susceptibility of human pluripotent stem cell-derived ventricular cardiomyocytes to arrhythmias, Biomaterials, 2013, 34(35), 8878–8886 CrossRef CAS PubMed.
- Y. Chen, J. Wang, B. Shen, C. W. Y. Chan, C. Wang and Y. Zhao,
et al. Engineering a freestanding biomimetic cardiac patch using biodegradable poly(lactic-co-glycolic acid) (PLGA) and human embryonic stem cell-derived ventricular cardiomyocytes (hESC-VCMs), Macromol. Biosci., 2015, 15(3), 426–436 CrossRef CAS PubMed.
- O. Caspi, A. Lesman, Y. Basevitch, A. Gepstein, G. Arbel and I. Huber,
et al. Tissue engineering of vascularized cardiac muscle from human embryonic stem cells, Circ. Res., 2007, 100(2), 263–272 CrossRef CAS PubMed.
- L. Almany and D. Seliktar, Biosynthetic hydrogel scaffolds made from fibrinogen and polyethylene glycol for 3D cell cultures, Biomaterials, 2005, 26(15), 2467–2477 CrossRef CAS PubMed.
- K. Shapira-Schweitzer and D. Seliktar, Matrix stiffness affects spontaneous contraction of cardiomyocytes cultured within a PEGylated fibrinogen biomaterial, Acta Biomater., 2007, 3(1), 33–41 CrossRef CAS PubMed.
- M. Habib, K. Shapira-Schweitzer, O. Caspi, A. Gepstein, G. Arbel and D. Aronson,
et al. A combined cell therapy and in situ tissue-engineering approach for myocardial repair, Biomaterials, 2011, 32(30), 7514–7523 CrossRef CAS PubMed.
- K. Flégeau, R. Pace, H. Gautier, G. Rethore, J. Guicheux and C. Le Visage,
et al. Toward the development of biomimetic injectable and macroporous biohydrogels for regenerative medicine, Adv. Colloid Interface Sci., 2017, 247(July), 589–609 CrossRef PubMed.
- M. Castilho, D. Feyen, M. Flandes-Iparraguirre, G. Hochleitner, J. Groll and P. A. F. Doevendans,
et al. Melt Electrospinning Writing of Poly-Hydroxymethylglycolide-co-ε-Caprolactone-Based Scaffolds for Cardiac Tissue Engineering, Adv. Healthcare Mater., 2017, 6(18), 1–9 Search PubMed.
- M. A. Mohamed, M. K. Hogan, N. M. Patel, Z.-W. Tao, L. Gutierrez and R. K. Birla, Establishing the Framework for Tissue Engineered Heart Pumps, Cardiovasc. Eng. Technol., 2015, 6(3), 220–229 CrossRef PubMed.
- L. Khait and R. K. Birla, Cell-based cardiac pumps and tissue-engineered ventricles, Regener. Med., 2007, 2(4), 391–406 CrossRef CAS PubMed.
- R. K. Birla, D. E. Dow, Y. C. Huang, F. Migneco, L. Khait and G. H. Borschel,
et al. Methodology for the formation of functional, cell-based cardiac pressure generation constructs in vitro, In Vitro Cell. Dev. Biol.: Anim., 2008, 44(8–9), 340–350 CrossRef PubMed.
- R. A. Li, W. Keung, T. J. Cashman, P. C. Backeris, B. V. Johnson and E. S. Bardot,
et al. Bioengineering an electro-mechanically functional miniature ventricular heart chamber from human pluripotent stem cells, Biomaterials, 2018, 163, 116–127 CrossRef CAS PubMed.
- L. A. MacQueen, S. P. Sheehy, C. O. Chantre, J. F. Zimmerman, F. S. Pasqualini and X. Liu,
et al. A tissue-engineered scale model of the heart ventricle, Nat. Biomed. Eng., 2018, 2, 930–941 CrossRef CAS PubMed.
- C. S. Ong, L. Nam, K. Ong, A. Krishnan, C. Y. Huang and T. Fukunishi,
et al. 3D and 4D bioprinting of the myocardium: Current approaches, challenges, and future prospects, Biomed. Res. Int., 2018, 2018 Search PubMed.
- X. Ma, J. Liu, W. Zhu, M. Tang, N. Lawrence and C. Yu,
et al. 3D bioprinting of functional tissue models for personalized drug screening and in vitro disease modeling, Adv. Drug Delivery Rev., 2018, 132, 235–251 CrossRef CAS PubMed.
- S. V. Murphy and A. Atala, 3D Bioprinting of Tissues and Organs, Nat. Biotechnol., 2014, 32(8), 773–785 CrossRef CAS PubMed.
- B. Duan, State-of-the-Art Review of 3D Bioprinting for Cardiovascular Tissue Engineering, Ann. Biomed. Eng., 2016, 45(1), 195–209 CrossRef PubMed.
- A. Van Den Berg, C. L. Mummery, R. Passier and A. D. Van der Meer, Personalised organs-on-chips: functional testing for precision medicine, Lab Chip, 2019, 19(2), 198–205 RSC.
|
This journal is © The Royal Society of Chemistry 2019 |
Click here to see how this site uses Cookies. View our privacy policy here.