DOI:
10.1039/C8BM01189C
(Paper)
Biomater. Sci., 2019,
7, 296-306
Repurposing antitubercular agent isoniazid for treatment of prostate cancer†
Received
26th September 2018
, Accepted 9th November 2018
First published on 12th November 2018
Abstract
The development of versatile antitumor agents with tumor-imaging, targeting and therapeutic activity is promising for clinical cancer therapy. Prostate cancer is still the one of the leading threats to males. Current therapies have restricted clinical efficiency for patients with advanced and metastatic prostate cancer. Recent studies demonstrate that monoamine oxidase A (MAOA) levels elevate with prostate cancer aggression and metastasis. In addition, MAOA inhibitor therapies have been reported as an effective means to reduce the metastasis of prostate cancer and extend mouse survival. Thus, these findings provide evidence that MAOA is promising for the treatment of metastatic and advanced prostate cancer. Herein, three isoniazid (INH)–dye conjugates were synthesized by conjugating MAOA inhibitor INH with mitochondria-targeting NIRF heptamethine dyes to improve the therapeutic efficacy of prostate cancer. These INH–dye conjugates could accumulate in PC-3 cellular mitochondria via organic anion transport peptide (OATP), increase ROS generation, and induce cancer cells apoptosis. In prostate cancer bearing xenografts, INH–dye conjugates showed significantly improved tumor-homing characteristics, resulting in potent antitumor activity via a reduction in MAOA activity. These results suggest that INH–dye conjugates have great potential to be used as versatile antitumor agents with prostate cancer targeting, NIR imaging, and potent antitumor efficacy.
1 Introduction
Prostate cancer ranks first amongst common types of cancer and second highest as a cause of cancer mortality in American males.1,2 Despite of progress in early diagnosis and surgical intervention, existing therapies (hormonal therapy, radiation, and cytotoxic chemotherapy) are efficacious only in localized prostate cancer and induce undesirable side effects.3,4 These therapies have limited clinical efficiency for patients with advanced castration resistant prostate cancer (CRPC) and metastatic prostate cancer. Additionally, CRPC has been demonstrated to be resistant to conventional chemotherapy.5,6 Discovering new prostate cancer targets and efficacious mechanism-based prostate cancer treatment is critical for efficient therapy of advanced prostate cancer.
Recent studies demonstrated a strong correlation between elevated monoamine oxidase A (MAOA) levels and prostate cancer aggression/metastasis. MAOA inhibitor therapies could effectively reduce metastasis and prolong mouse survival in prostate cancer bearing xenografts.7,8 MAOA plays a vital role in epithelial–mesenchymal transition (EMT) via the production of excessive hydrogen peroxide, a main ROS by-product generated by the MAOA-mediated oxidation reaction. EMT enhances the aggressiveness and metastasis of prostate cancer cells.9 In addition, MAOA expression elevates following docetaxel treatment and results in chemotherapy resistance.5 These findings provide evidence that targeting MAOA could be promising for the treatment of metastatic and advanced prostate cancer. Isoniazid (INH) is an irreversible inhibitor of MAOA that is widely regarded as a major anti-tuberculosis drug.10,11 Moreover, its hydrazone and hydrazide derivatives show better efficacy and less hepatotoxicity than INH itself, due to the blockage of N-acetylation of INH.12,13
Near-infrared fluorescent (NIRF) heptamethine cyanine dye has great potential to be used in noninvasive cancer imaging and diagnosis. These dyes could improve the sensitivity and selectivity of cancer diagnosis, owing to their low background autofluorescence with an emission wavelength in NIR region (700–900 nm).14–16 Recently, several structure-inherent targeting NIRF heptamethine cyanine dyes (IR-783, IR-808 DB, and MHI-148) have been developed for preferential accumulation in cancers including prostate cancer, with increased uptake in cellular mitochondria without chemical modification.17–21 Mitochondria are regarded as a preferred subcellular target for cancer therapy due to their vital role in energy production and cell apoptosis.22,23 Lipophilic cationic properties were recognized as essential for targeting mitochondria, which explains the selective mitochondria accumulation of these NIRF dyes.23 Furthermore, tumor targeting mechanism studies show that the selective cancer accumulation of NIRF dyes was mainly mediated by enhanced expression of organic anion transport peptide (OATP).19 OATP is overexpressed in many types of cancers, including prostate cancer. Moreover, the OATP1B3 subtype plays a vital role in the transportation of NIRF dyes into tumor cells.18,24,25
Herein, we synthesized and characterized three INH–dye conjugates by conjugating INH with mitochondria-targeting NIRF heptamethine dyes to improve the therapeutic efficacy of prostate cancer, abbreviated as NIC-1, NIC-2, and NIC-3. Tumor-specific targeting properties of NIRF heptamethine dyes could improve the therapeutic potential of MAOA inhibitor INH and reduce its side effects. In addition, the conjugation of an NIR imaging dye may be convenient to visually evaluate the cell uptake and biodistribution of INH–dye conjugates as well as image-guided tumor diagnosis. In the present study, we investigated the potential of INH–dye conjugates to act as a multifunctional agent for tumor NIR imaging, cellular mitochondrial targeting, and antitumor activities.
2 Materials and methods
2.1 Cell line and reagents
Human prostate cancer cells (PC-3) were purchased from the Chinese Academy of Sciences (Shanghai, China) and cultured in F12K (GIBCO, USA) containing 10% FBS and 1% penicillin/streptomycin (GIBCO, USA). INH was obtained from Aladdin (Shanghai, China). Ethyl 4-bromobutanoate was obtained from Meryer (Shanghai, China). MHI-148 was synthesized as reported earlier.21 Hoechst, MitoTracker, and Matrigel were purchased from BD Biosciences (USA). The PSA levels and MAOA activities test ELISA kit was purchased from Cusabio Biotech (Wuhan, China). The TUNEL assay kit was purchased from KeyGen Biotech (Nanjing, China).
2.2 Synthesis of INH–dye conjugates
See the synthesis methods in the ESI.†
2.3 Optical properties
To evaluate the optical properties of INH–dye conjugates, NIC-1, NIC-2, and NIC-3 were dissolved in MeOH or 100% FBS at a final concentration of 10 μM. MHI-148 dyes were used as a control. UV-Vis absorption spectra were acquired using a microplate reader. Photoluminescence was detected using a microplate reader (740 nm excitation, continuous wavelength from 760 to 840 nm emission) and an IVIS system (PerkinElmer, USA). To evaluate the photostability in serum, INH–dye conjugates were incubated in FBS at 37 °C and fluorescence signals were detected at timed intervals.
2.4
In vitro cytotoxicity assay
The in vitro cytotoxicity of INH–dye conjugates was investigated by MTT viability assay against PC-3 cells. Briefly, cells were seeded in a 96-well plate (3000 cells per well) for 12 h attachment. Then, cells were treated with serial dilutions of INH, NIC-1, NIC-2, or NIC-3 for 48 h. Untreated wells were utilized as a control. At the end of incubation, 20 μL of MTT (5 mg mL−1) was added, and the plate was incubated for additional 4 h. The medium was discarded and the intracellular blue-violet formazan crystals were dissolved in DMSO (200 μL). The optimal density of each well was detected at 570 nm using a microplate reader (Thermo Scientific, USA). The cell viability was calculated using the following formula: (ODtreated/ODcontrol) × 100%. All experiments were carried out in triplicate.
2.5 Apoptosis assay
Apoptotic PC-3 cells resulting from treatment with INH and INH–dye conjugates were evaluated by double staining with AV-FITC and PI. Briefly, PC-3 cells seeded in 6-well plates were incubated with INH and INH–dye conjugates at a final concentration of 20 μM for 24 h. Thereafter, cells were collected, stained with AV-FITC and PI for another 15 min, and analyzed by fluorescence-activated cell sorting (FACS) analysis (Becton Dickinson, USA).
2.6 Detection of intracellular ROS
Intracellular ROS levels were evaluated by confocal laser scanning microscopy (CLSM) and flow cytometry. Briefly, cells were seeded on 12 mm cover glasses for 24 h attachment. After incubation with INH and INH–dye conjugates (20 μM) for 24 h at 37 °C, cells were washed with PBS and incubated with F12K medium containing 10 μM DCFH-DA for an additional 30 min. The nuclei were stained with Hoechst at 37 °C for 15 min. Finally, cells were washed and the prepared covered slip was observed using CLSM (Nikon Corp., Japan). For quantitative determination, PC-3 cells incubated with drugs (20 μM) were collected and suspended in F12K medium containing 10 μM DCFH-DA for an additional 30 min. Cells were washed and detected by FACS analysis.
2.7 Cell uptake and subcellular localization
In vitro cellular uptake was evaluated using CLSM and flow cytometry. PC-3 cells were seeded on 12 mm cover glasses for 12 h attachment. After incubation with INH–dye conjugates (20 μM) for 1 h at 37 °C, cells were stained with MitoTracker for another 30 min. The nuclei were stained with Hoechst at 37 °C for 15 min. Finally, the prepared covered slips were observed using CLSM. For quantitative determination, after incubation with INH–dye conjugates (20 μM) for 1 h at 37 °C or 0 °C, PC-3 cells were re-suspended in PBS. Fluorescence signals were detected by FACS analysis. To confirm the contribution of OATP to the cell uptake of INH–dye conjugates, cells were pre-incubated with excess BSP (an OATP competitive inhibitor) prior to the uptake study.
2.8 Animal and tumor xenografts
Male athymic nude BALB/C mice (23–26 g) were afforded by the Laboratory Animal Center of Shenyang Pharmaceutical University (Shenyang, Liaoning, China). All animal experiments were conducted according to the Guidelines for the Care and Use of Laboratory Animals approved by the Institutional Animal Ethical Care Committee (IAEC) of Shenyang Pharmaceutical University. Human prostate cancer PC-3 cells (5 × 106 in 50% Matrigel) were injected into the armpit region of the mice to establish tumor xenograft models.
2.9
Ex vivo biodistribution
PC-3 tumor bearing mice were used to investigate the biodistribution of INH–dye conjugates. When the tumor size was approximately 150 mm3, NIC-1, NIC-2 and NIC-3 were administered intravenously (2.5 μM kg−1). At 48 h post-injection, the major organs (heart, liver, spleen, lung and kidney) and tumors were collected. The ex vivo imaging signals and fluorescence intensity were determined using an IVIS imaging system.
2.10
In vivo antitumor efficacy
PC-3 tumor bearing mice were used to investigate the antitumor efficacy of INH–dye conjugates. When the tumor size reached approximately 100 mm3, mice were randomly divided into four groups: (1) saline, (2) INH, (3) NIC-1, and (4) NIC-3. These formulations were administrated intravenously via tail veins every two days for a total of five injections (37.5 μM kg−1). Tumor volume and body weight were monitored. After two days of the last treatment, the mice serum was collected to evaluate hepatiorenal function. The serum MAOA activities and PSA levels were evaluated using ELISA. The major organs were collected and fixed by formalin for H&E staining. Tumor apoptosis was evaluated by TUNEL test.
2.11 Statistical analysis
Data are expressed as mean ± SD. The comparison between groups was analyzed with Student's t-test and one-way ANOVA. Statistical difference was significant at P < 0.05.
3 Results and discussion
3.1 Design and synthesis of INH–dye conjugates
As illustrated in Scheme 1, we synthesized three novel INH–dye conjugates by conjugating MAOA inhibitor INH to NIRF heptamethine dye MHI-148 and its derivatives, abbreviated as NIC-1, NIC-2, and NIC-3. NIC-1 and NIC-3 were employed with a cyclohexenyl ring in the polymethine chain, which has been proven to be important for its tumor-homing capacity and enhanced photostability.23,26–28 For comparison, NIC-2 was synthesized by conjugating INH with a MHI-148 derivative without a fused substituted cyclohexenyl ring. The chemical structures of the three INH–dye conjugates were confirmed by MS and 1H NMR (Fig. S1–S3†). The integration of tumor-specific targeting characteristic into the conjugates could improve the therapeutic efficacy of INH and reduce its undesired side effects. Additionally, the fluorescence of heptamethine dyes could be used to evaluate the cell uptake and biodistribution of INH–dye conjugates as well as image-guided tumor diagnosis.
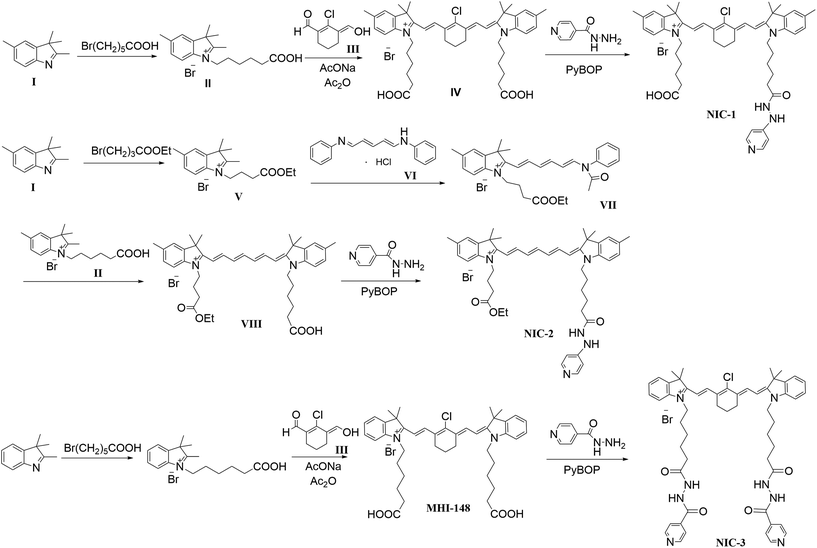 |
| Scheme 1 Synthesis of three INH–dye conjugates (NIC-1, NIC-2, and NIC-3). | |
3.2 Optical properties of INH–dye conjugates
The optical properties of INH–dye conjugates were evaluated in MeOH and FBS. INH–dye conjugates exhibited different optical properties and photostability due to their structural differences in the polymethine chain and chemical conjugation. As shown in Fig. 1 and Fig. S4,† the maximal excitation wavelength of NIC-1 was approximately 800 nm, and the maximal emission wavelength was 808 nm in FBS and 820 nm in MeOH. NIC-1 displayed similar optical properties with MHI-148 dye, suggesting that the chemical modification on one side chain of NIRF heptamethine cyanine dye did not change its fluorescence properties. However, NIC-3 exhibited weaker fluorescence intensity when compared with NIC-1 at the same concentration (Fig. 1B and D). This may be attributed to the change in electronic properties of aromatic backbones and fluorescence properties due to conjugation with both side chains of MHI-148 dye.19 The maximal excitation and emission wavelength of NIC-2 exhibited a blue-shift compared with those of NHI-148 dye. As shown in Fig. 1C, the fluorescence intensity of NIC-1 and NIC-3 was fairly stable for 24 h in serum, supporting their potential use in biomedical imaging and probably in future image-guided tumor diagnosis. However, the fluorescence intensity of NIC-2 gradually decreased with prolonged incubation time, and dropped to 61.07% at 24 h. These results may be attributed to the rigid cyclohexenyl ring introduced in the polymethine chain of NIC-1 and NIC-3, leading to enhanced photostability.27,28
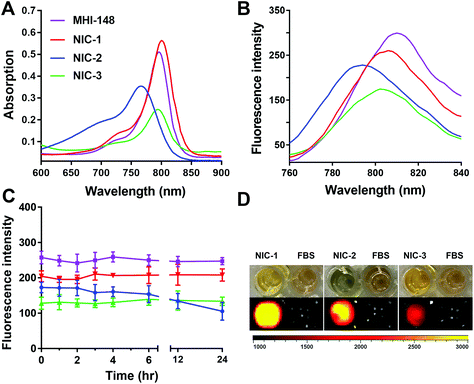 |
| Fig. 1 Optical properties of three INH–dye conjugates in FBS. (A) The absorption spectra of three INH–dye conjugates in FBS. (B) The emission spectra of three INH–dye conjugates in FBS. (C) Fluorescence stability of three INH–dye conjugates in FBS for 24 h. (D) Optical images of three INH–dye conjugates in FBS. | |
3.3 Cellular uptake and subcellular localization of INH–dye conjugates
NIRF heptamethine cyanine dye MHI-148 has been proven to show tumor-homing capacity via targeting cellular mitochondria, especially for prostate cancer.9,18 We further evaluated the potential of INH–NIRF dye conjugates as drug delivery carriers for image-guided active-targeted antitumor therapy. CLSM was utilized to investigate the subcellular localization of INH–dye conjugates in PC-3 cells stained with MitoTracker. The confocal imaging demonstrated that INH–dye conjugates accumulated in cellular mitochondria, as localized with mitochondria-specific dye MitoTracker (Fig. 2). This result indicates that chemical modification does not change the mitochondria-targeting function of the MHI-148 dye and that mitochondria may be a key target for the INH–dye conjugates to kill cancer cells.
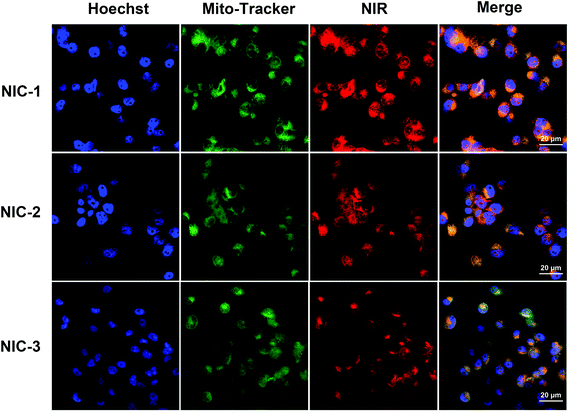 |
| Fig. 2 Subcellular localization of INH–dye conjugates NIC-1, NIC-2, and NIC-3. | |
Mitochondria have been found to be a good target for cancer therapy due to its vital role in energy production and cell apoptosis.29–31 Lipophilic cationic properties were recognized as essential for targeting mitochondria, which explains the selective mitochondria accumulation of INH–dye conjugates.23 Further studies show the selective cancer accumulation of NIRF dyes was mainly mediated by OATP. OATP is overexpressed in a spectrum of cancers, including prostate cancer.24 To investigate uptake mechanism, cells were co-cultured with drugs at 4 °C or 37 °C. As shown in Fig. 3, the uptake of NIC-1 (NIR signal) was significantly reduced with the 4 °C incubation compared to 37 °C. This result suggests that the uptake of NIC conjugates is energy-dependent. Moreover, cells pre-incubated with BSP showed a significantly reduced NIR dye uptake of NIC-1. This data demonstrates that OATP mediated the uptake of INH–dye conjugates in PC-3 cells.
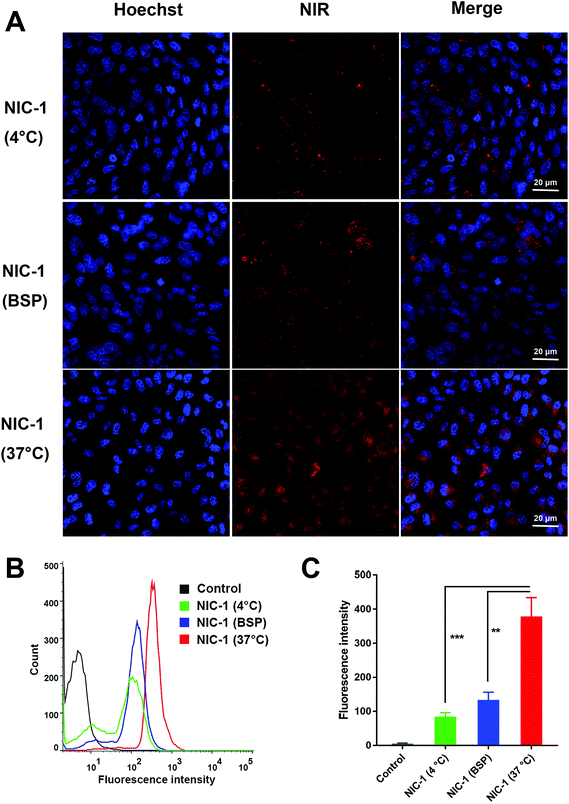 |
| Fig. 3 Cell uptake of INH–dye conjugate NIC-1. (A) Confocal images of prostate cancer cells incubated with NIC-1 in different conditions. (B) Cell uptake of NIC-1 detected with flow cytometry. (C) The fluorescence signals of cells after incubation with NIC-1. | |
3.4
In vitro cytotoxicity, cell apoptosis and intracellular ROS
The in vitro cytotoxicity of INH–dye conjugates was evaluated using a MTT assay. INH exhibited no evident cytotoxicity (Fig. 4A). Notably, INH–dye conjugates displayed concentration-dependent cytotoxicity, and NIC-1 could eliminate nearly all the cells at the concentration of 200 μM. Results of IC50 values suggested INH–dye conjugates had higher cytotoxicity than that of INH against PC-3 cells (Fig. 4B).
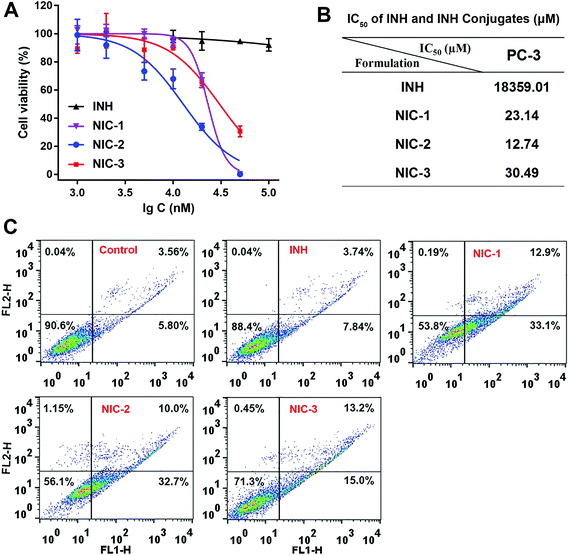 |
| Fig. 4 Cytotoxicity and cell apoptosis assay of INH–dye conjugates against PC-3 cells. (A) In vitro cytotoxicity of INH–dye conjugates (n = 3). (B) The IC50 value of PC-3 cells treated with INH and INH–dye conjugates. (C) Flow cytometry analysis of the apoptosis rate for INH and INH–dye conjugates for 24 h. | |
Flow cytometry was used to determine both early and late stages of apoptosis (Fig. 4C). NIC-1 (46.0%), NIC-2 (42.7%) and NIC-3 (28.2%) resulted in a robust increase in cell apoptosis compared with INH (11.6%). INH solution barely induced cell apoptosis, which might be due to its inferior passive uptake by cells (log
P = −0.69). The hydrazone and hydrazide derivatives of INH showed better efficacy and less hepatotoxicity than INH itself, owing to the blockage of the N-acetylation of INH.12,13 Therefore, we reason that INH–dye conjugates would possess better MAOA inhibition activity than INH by conjugating its terminal amino group with MHI-148 dye. In addition, the preferential accumulation of NIH conjugates in prostate cancer cells mediated by OATP resulted in an enhanced anticancer efficiency and apoptosis rates for INH–dye conjugates.
Intracellular ROS production was recognized as a key mechanism in drug-induced cell death. Studies show that NIRF dye could accumulate in the cancer cellular mitochondria, enhance ROS generation, reduce mitochondrial membrane potential, and finally cause cancer cells apoptosis.18,20 Therefore, intracellular ROS level was detected using a DCFH-DA probe after INH and INH–dye conjugates treatment. Upon oxidation by ROS, DCFH-DA was converted to a fluorescent moiety (DCFH). Accordingly, intracellular fluorescence signals were detected using CLSM (Fig. 5A) and flow cytometry (Fig. 5B and C). As shown in Fig. 5, ROS production significantly increased after treatment with INH–dye conjugates. Moreover, NIC-1 could induce more ROS production in PC-3 cells compared with INH and NIC-2. Mitochondria are commonly regarded as a preferred target for cancer therapy and are especially susceptible to excessive ROS.23 Therefore, we reason that INH–dye conjugates would possess potent antitumor efficacy via accumulation in the cancer cellular mitochondria and enhanced ROS generation.
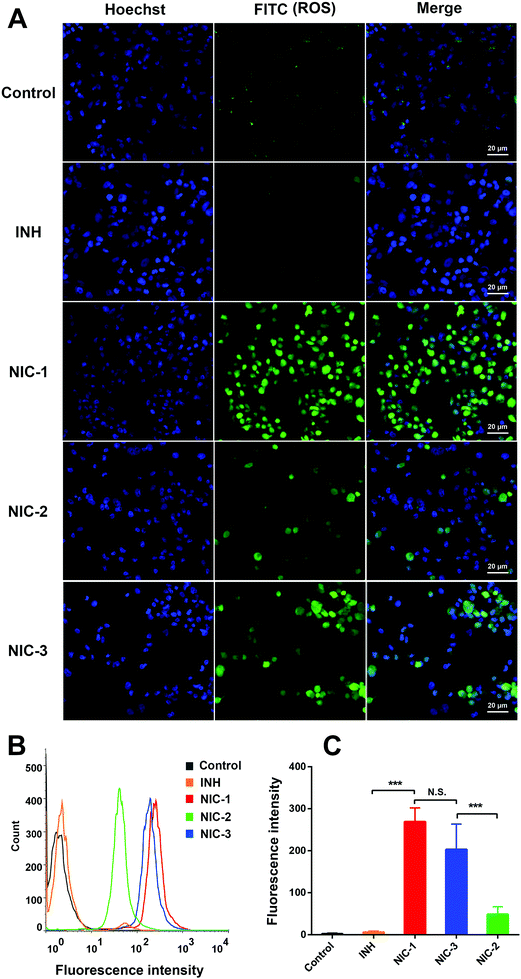 |
| Fig. 5 Intracellular ROS production of PC-3 cancer cells after INH–dye conjugate treatment. (A) ROS production tested by CLSM. (B) ROS production detected with flow cytometry. (C) The average fluorescence signals of prostate cancer cells treated by DCFH-DA. | |
3.5
Ex vivo biodistribution
Ex vivo biodistribution of INH–dye conjugates was investigated in prostate cancer bearing mice and measured by IVIS. As shown in Fig. 6, NIC-2 exhibited high retention in the lung, but negligible fluorescence signal was detected in the tumor, which may be due to its poor photochemical stability. In comparison, NIC-1 and NIC-3 exhibited distinctly higher fluorescence intensity in the tumor, and the fluorescence signal for tumors was the strongest apart from the lung. The enhanced tumor retention supports the use of NIC-1 and NIC-3 for future image-guided tumor diagnosis and therapy. The conjugation, with proper modification of NIRF heptamethine cyanine dye, did not change its tumor specific delivery properties.
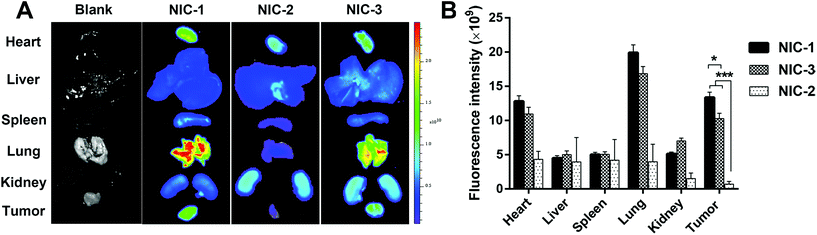 |
| Fig. 6
Ex vivo distribution in prostate cancer bearing mice. (A) NIR fluorescence images of NIC-1, NIC-2, and NIC-3 at 48 h. (B) NIR fluorescence signals of tissues treated with NIC-1, NIC-2, and NIC-3. | |
3.6
In vivo antitumor efficacy of INH–dye conjugates
PC-3 bearing mice were used to investigate the antitumor efficacy of INH–dye conjugates. INH exhibited a moderate tumor inhibition effect as compared to the control group. Notably, mice treated with NIC-1 showed a significant reduction in tumor size, exhibiting no significant difference with NIC-3 (Fig. 7A). Serum prostate-specific antigen (PSA) levels were conventionally screened as a serological indicator for prostate cancer. High levels of PSA have been reported in advanced and metastatic prostate cancer patients, suggesting that PSA plays a key role in prostate cancer progression, invasion, and metastasis. In addition, PSA testing is widely used to evaluate the effect and response of prostate cancer treatment.32,33 After two days of the last treatment, mice serum was collected, and the serum PSA level and MAOA activity was measured by ELISA. As shown in Fig. 7B, INH–dye conjugate treated mice demonstrated a significant decrease in PSA level compared with INH solution. In addition, INH–dye conjugates exhibited a significant decrease in MAOA inhibition activity compared with INH solution. These results validated that INH–dye conjugates had more potent MAOA-inactivating efficacy than INH.
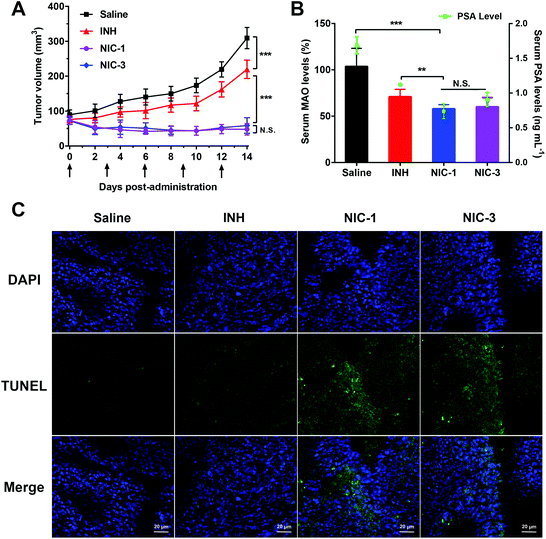 |
| Fig. 7
In vivo antitumor efficacy of INH–dye conjugates in prostate cancer bearing mice. (A) Tumor volume after injection of saline, INH, NIC-1, and NIC-3 (n = 5). (B) Serum PSA level and MAOA activity in prostate cancer bearing mice analyzed by ELISA at the end of the in vivo antitumor efficacy study (n = 3). (C) Confocal images of tumor sections analyzed by the TUNEL assay at the end of the antitumor efficacy study. **P < 0.01, ***P < 0.001. | |
The TUNEL test was utilized to investigate the apoptosis of tumor cells. A robust apoptosis was detected in tumor sections after NIC-1 and NIC-3 treatment, compared with INH (Fig. 7C). The potent antitumor efficacy of INH–dye conjugates may be due to the preferential accumulation of NIH conjugates in prostate cancer via OATP transportation and the enhanced MAOA-inactivating efficacy. In addition, no significant change in body weight and hematological parameters was detected in all groups. No significant histological abnormalities were detected in H&E stained tissue sections (Fig. 8). These results suggested that the INH–dye conjugates showed good safety with negligible treatment-induced toxicity.
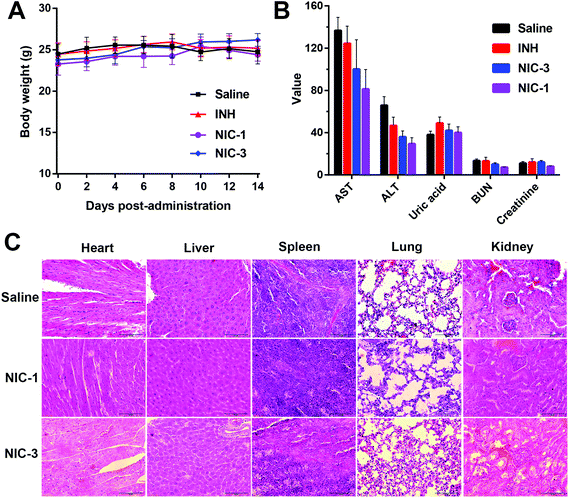 |
| Fig. 8 Body weight, hematological parameters, and H&E staining. (A) Body weight changes of prostate cancer bearing mice after administration of saline, INH, NIC-1, and NIC-3 (n = 5). (B) Hepatic and renal function indicators of prostate cancer bearing mice at the end of the antitumor efficacy study (n = 5). (C) H&E staining in organs at the end of the antitumor efficacy study. | |
4 Conclusion
In this study, three INH–dye conjugates were designed and synthesized by conjugating MAOA inhibitor INH to mitochondria-targeting NIRF heptamethine dyes (abbreviated NIC-1, NIC-2, and NIC-3) to improve the therapeutic efficacy of prostate cancer. Both in vitro and in vivo results suggest that INH–dye conjugates selectively accumulated in prostate cancer cellular mitochondria via OATP transportation. These INH–dye conjugates have great potential to be used as versatile imaging and targeting agents for the treatment of prostate cancer. The potent antitumor efficacy was mainly derived from MAOA-inactivating and cancer cellular mitochondria-targeting activity, enabling them to be used as potential agents for imaging-guided targeting therapy of prostate cancer.
Conflicts of interest
There are no conflicts of interest. This research did not receive any specific grant from funding agencies in the public, commercial, or not-for-profit sectors.
References
- R. L. Siegel, K. D. Miller and A. Jemal, CA-Cancer J. Clin., 2017, 67, 7–30 CrossRef PubMed.
- Q. Lv, J. Yang, R. Zhang, Z. Yang, Z. Yang, Y. Wang, Y. Xu and Z. He, Mol. Pharm., 2018, 15, 1842–1852 CrossRef CAS PubMed.
- U. Elsässerbeile, P. Bühler and P. Wolf, Curr. Drug Targets, 2009, 10, 118–125 CrossRef.
- O. Flores, S. Santra, C. Kaittanis, R. Bassiouni, A. S. Khaled, A. R. Khaled, J. Grimm and J. M. Perez, Theranostics, 2017, 7, 2477–2494 CrossRef CAS PubMed.
- R. R. Gordon, M. Wu, C. Y. Huang, W. P. Harris, H. G. Sim, J. M. Lucas, I. Coleman, C. S. Higano, R. Gulati, L. D. True, R. Vessella, P. H. Lange, M. Garzotto, T. M. Beer and P. S. Nelson, PLoS One, 2014, 9, e104271 CrossRef PubMed.
- H. Aloysius and L. Hu, Med. Res. Rev., 2015, 35, 554 CrossRef CAS PubMed.
- C. P. Liao, T. P. Lin, P. C. Li, L. A. Geary, K. Chen, V. P. Vaikari, J. B. Wu, C. H. Lin, M. E. Gross and J. C. Shih, Oncogene, 2018, 37, 5175–5190 CrossRef CAS PubMed.
- J. B. Wu, L. Yin, C. Shi, Q. Li, P. Duan, J. M. Huang, C. Liu, F. Wang, M. Lewis, Y. Wang, T. P. Lin, C. C. Pan, E. M. Posadas, H. E. Zhau and L. W. Chung, Cancer Cell, 2017, 31, 368–382 CrossRef CAS PubMed.
- J. B. Wu, T. P. Lin, J. D. Gallagher, S. Kushal, L. W. Chung, H. E. Zhau, B. Z. Olenyuk and J. C. Shih, J. Am. Chem. Soc., 2015, 137, 2366–2374 CrossRef CAS PubMed.
- V. S. Velezheva, P. J. Brennan, V. Y. Marshakov, D. V. Gusev, I. N. Lisichkina, A. S. Peregudov, L. N. Tchernousova, T. G. Smirnova, S. N. Andreevskaya and A. E. Medvedev, J. Med. Chem., 2004, 47, 3455–3461 CrossRef CAS PubMed.
- N. Zareifopoulos and G. Panayiotakopoulos, Clin. Drug Invest., 2017, 37, 423–437 CrossRef CAS PubMed.
- D. Vila-Vicosa, B. L. Victor, J. Ramos, D. Machado, M. Viveiros, J. Switala, P. C. Loewen, R. Leitao, F. Martins and M. Machuqueiro, Mol. Pharm., 2017, 14, 4597–4605 CrossRef CAS PubMed.
- P. F. M. Oliveira, B. Guidetti, A. Chamayou, C. Andre-Barres, J. Madacki, J. Kordulakova, G. Mori, B. S. Orena, L. R. Chiarelli, M. R. Pasca, C. Lherbet, C. Carayon, S. Massou, M. Baron and M. Baltas, Molecules, 2017, 22, 1457 CrossRef PubMed.
- L. Yuan, W. Lin, K. Zheng, L. He and W. Huang, Chem. Soc. Rev., 2013, 42, 622–661 RSC.
- R. G. Thomas and Y. Y. Jeong, Chonnam Med. J., 2017, 53, 83 CrossRef PubMed.
- X. Meng, Y. Yang, L. Zhou, L. Zhang, Y. Lv, S. Li, Y. Wu, M. Zheng, W. Li, G. Gao, G. Deng, T. Jiang, D. Ni, P. Gong and L. Cai, Theranostics, 2017, 7, 1781–1794 CrossRef CAS PubMed.
- S. Luo, E. Zhang, Y. Su, T. Cheng and C. Shi, Biomaterials, 2011, 32, 7127–7138 CrossRef CAS PubMed.
- S. Luo, Z. Yang, X. Tan, Y. Wang, Y. Zeng, Y. Wang, C. Li, R. Li and C. Shi, ACS Appl. Mater. Interfaces, 2016, 8, 17176–17186 CrossRef CAS PubMed.
- J. B. Wu, C. Shi, C. Y. Chu, Q. Xu, Y. Zhang, Q. Li, J. S. Yu, H. E. Zhau and L. W. K. Chung, Biomaterials, 2015, 67, 1–10 CrossRef CAS PubMed.
- Y. Wang, T. Liu, E. Zhang, S. Luo, X. Tan and C. Shi, Biomaterials, 2014, 35, 4116–4124 CrossRef CAS PubMed.
- Q. Lv, X. Yang, M. Wang, J. Yang, Z. Qin, Q. Kan, H. Zhang, Y. Wang, D. Wang and Z. He, J. Controlled Release, 2018, 279, 234 CrossRef CAS PubMed.
- Y. Tan, Y. Zhu, Y. Zhao, L. Wen, T. Meng, X. Liu, X. Yang, S. Dai, H. Yuan and F. Hu, Biomaterials, 2018, 154, 169–181 CrossRef CAS PubMed.
- X. Tan, S. Luo, L. Long, Y. Wang, D. Wang, S. Fang, Q. Ouyang, Y. Su, T. Cheng and C. Shi, Adv. Mater., 2017, 29, 1704196 CrossRef PubMed.
- J. B. Wu, C. Shao, X. Li, C. Shi, Q. Li, P. Hu, Y. T. Chen, X. Dou, D. Sahu, W. Li, H. Harada, Y. Zhang, R. Wang, H. E. Zhau and L. W. Chung, Biomaterials, 2014, 35, 8175–8185 CrossRef CAS PubMed.
- E. Zhang, S. Luo, X. Tan and C. Shi, Biomaterials, 2014, 35, 771–778 CrossRef CAS PubMed.
- S. Luo, X. Tan, S. Fang, Y. Wang, T. Liu, X. Wang, Y. Yuan, H. Sun, Q. Qi and C. Shi, Adv. Funct. Mater., 2016, 26, 2826–2835 CrossRef CAS.
- N. S. James, Y. Chen, P. Joshi, T. Y. Ohulchanskyy, M. Ethirajan, M. Henary, L. Strekowsk and R. K. Pandey, Theranostics, 2013, 3, 692–702 CrossRef PubMed.
-
L. Strękowski and B. A. Armitage, Heterocyclic polymethine dyes : synthesis, properties and applications, Springer, 2008 Search PubMed.
- C. Wu, L. Wang, Y. Tian, X. Guan, Q. Liu, S. Li, X. Qin, H. Yang and Y. Liu, ACS Appl. Mater. Interfaces, 2018, 10, 6942–6955 CrossRef CAS PubMed.
- J. Zielonka, J. Joseph, A. Sikora, M. Hardy, O. Ouari, J. Vasquez-Vivar, G. Cheng, M. Lopez and B. Kalyanaraman, Chem. Rev., 2017, 117, 10043–10120 CrossRef CAS PubMed.
- W. S. Shin, S. K. Park, P. Verwilst, S. Koo, J. H. Lee, S. G. Chi and J. S. Kim, Chem. Commun., 2017, 53, 1281–1284 RSC.
- A. Barve, W. Jin and K. Cheng, J. Controlled Release, 2014, 187, 118–132 CrossRef CAS PubMed.
- S. A. Williams, P. Singh, J. T. Isaacs and S. R. Denmeade, Prostate, 2007, 67, 312 CrossRef PubMed.
Footnote |
† Electronic supplementary information (ESI) available. See DOI: 10.1039/c8bm01189c |
|
This journal is © The Royal Society of Chemistry 2019 |
Click here to see how this site uses Cookies. View our privacy policy here.